Abstract
Localised fires are an important accidental scenario for the offshore industry due to the extent of their potential damage. Its consequences may be localised damage, weakening of structural elements, blast when a storage tank reaches its critical temperature, or the structure's progressive collapse. The correct sizing of the fire protection (passive and/or active) system allows reducing or delaying the potential structure's damage. This article evaluates the use of the LF-ESF methodology at the design stage to estimate the efficiency of the passive fire protection layer (PFP) in upper offshore structures exposed to localised fires. Fire conditions are assumed to result from a typical process involving the combustion of hydrocarbons. Three fire scenarios are evaluated using the LF-ESF model proposed by the authors and based on updates of simple methodologies widely used in the literature. In order to verify its accuracy, its results are compared with a model developed in a CFD-based package for a fire scenario. Thermo-mechanical analysis is performed employing a finite element model that considers the temperature-dependent physical and geometric non-linearities. The estimated thermal load is entered into the thermo-mechanical model in combination with the pre-existing operational loads to evaluate the structure's behaviour. Despite the slight overestimation of the thermal field from the LF-ESF model, compared to the CFD-FEM methodology, the results obtained allow the selection of the PFP without being too conservative and at low computational cost.











Similar content being viewed by others
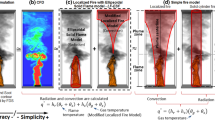
Abbreviations
- \({c}_{p}\,[\rm{J}/\rm{g}-\rm{K}]\) :
-
Specific heat
- \({e}_{\rm{r},\rm{a}\rm{b}\rm{s}}^{\prime\prime}\) [\(\rm{k}\rm{W}/{\rm{m}}^{2}\)]:
-
Radiant energy absorbed
- \({e}_{\rm{r}, {\text{emi}} }^{\prime\prime}\) [\(\rm{k}\rm{W}/{\rm{m}}^{2}\)]:
-
Radiant energy emitted
- \({e}_{\rm{r}, {\text{inc}} }^{\prime\prime}\) [\(\rm{k}\rm{W}/{\rm{m}}^{2}\)]:
-
Radiant incident energy
- \({e}_{\rm{r}, {\text{ref}} }^{\prime\prime}\) [\(\rm{k}\rm{W}/{\rm{m}}^{2}\)]:
-
Radiant energy reflected
- \(g\,[\rm{m}/{\rm{s}}^{2}]\) :
-
Gravitational acceleration
- \({h}_{{\text{tot}}}^{ {\text{AST}} }\) [\(\rm{k}\rm{W}/{\rm{m}}^{2}\rm{K}\)]:
-
Net adiabatic heat transfer coefficient
- \({h}_ {\text{conv}} \) [\(\rm{k}\rm{W}/{\rm{m}}^{2}\rm{K}\)]:
-
Convective heat transfer coefficient
- \(k\,[\rm{W}/\rm{m}\rm{K}]\) :
-
Thermal conductivity
- \({m}^{\prime\prime}\) \([\) kg/m2 − s]:
-
Burning rate per unit area
- \({q}_ {\text{conv}} ^{\prime\prime}\) [\(\rm{k}\rm{W}/{\rm{m}}^{2}\)]:
-
Convective heat flux
- \({q}_{\rm{f}}\) [\(kW/{m}^{2}\)]:
-
Heat release rate per unit area
- \({q}_{{\text{tot}}}^{\prime\prime}\) [\(kW/{m}^{2}\)]:
-
Net heat flux
- \({q}_{\rm{r}\rm{a}\rm{d}}^{\prime\prime}\) [\(kW/{m}^{2}\)]:
-
Radiant heat flux
- \(r\)[m]:
-
Radial distance to flame axis
- \({t}_{\rm{d}}\) [s]:
-
Fire decay time for NFSC curve
- \({t}_{\rm{g}}\) [s]:
-
Fire growth time for NFSC curve
- \({t}_{\rm{s}}\) [s]:
-
Fire constant behaviour time for NFSC curve
- \({A}_{\rm{f}\rm{s}}\)[m2]:
-
Free surface area of the pool fire
- \(D\) [m]:
-
Equivalent diameter
- \({H}_{ {\text{fl}} }\)[m]:
-
Flame height
- \( {\text{HRR}}\) [kW]:
-
Heat release rate
- \({\alpha }_{\rm{s}}\) [-]:
-
Absorptivity
- \(\beta \) [−]:
-
Mean beam length corrector
- \({\varepsilon }_{\rm{s}}\) [−]:
-
Surface emissivity
- \({\theta }_{ {\text{AST}} }\) [K]:
-
Adiabatic surface temperature
- \({\theta }_{ {\text{fl}} }\) [K]:
-
Real flame temperature
- \({\theta }_{\rm{g}}\) [°C, K]:
-
Gas temperature
- \({\theta }_{\rm{s}}\) [°C, K]:
-
Exposed surface’s temperature
- \({\theta }_{\infty }\,[K]\) :
-
Ambient temperature
- \(\kappa \) [1/m]:
-
Absorption–extinction coefficient of the flame
- \(\rho \,[\rm{k}\rm{g}/{\rm{m}}^{3}]\) :
-
Specific mass
- \({\rho }_{\infty }\,[\rm{k}\rm{g}/{\rm{m}}^{3}]\) :
-
Air density
- \(\sigma \) [\(\rm{k}\rm{W}/{\rm{m}}^{2}-{\rm{K}}^{4}\)]:
-
Stefan–Boltzmann constant
- \(\varphi \)[−]:
-
Fire intensity coefficient
- \(\Delta {H}_{{\text{c, eff}}}\) [kJ/kg]:
-
Combustion specific heat
References
M.J. Hurley, D. Gottuk, J.R. Hall, K. Harada, E. Kuligowski, M. Puchovsky et al., SFPE Handbook of Fire Protection Engineering, 5th edn. (Springer, New York, 2016)
J.K. Paik, J. Czujko, B.J. Kim, J.K. Seo, H.S. Ryu, Y.C. Ha et al., Quantitative assessment of hydrocarbon explosion and fire risks in offshore installations. Mar Struct 24, 73–96 (2011). https://doi.org/10.1016/j.marstruc.2011.02.002
M.R. Manco, M.A. Vaz, A. Landesmann, Analysis of oil tanker deck under hydrocarbon fire. Int. J. Model. Simul. Pet. Ind 8(2), 17–24 (2014)
M.R. Manco, A. Landesmann, M.A. Vaz, J.C.R. Cyrino, Numerical model for analysis of offshore structures subjected to pool fires. Mar. Syst. Ocean Technol. 11, 19–29 (2016). https://doi.org/10.1007/s40868-016-0014-y
Y. Jin, B.-S. Jang, Probabilistic fire risk analysis and structural safety assessment of FPSO topside module. Ocean Eng 104, 725–737 (2015). https://doi.org/10.1016/j.oceaneng.2015.04.019
M. Gillet, L. Perez, L. Autrique, A model based predictive tool for fire safety intumescent coatings design. Fire Saf. J. 110, 102908 (2019). https://doi.org/10.1016/j.firesaf.2019.102908
G.-Q. Li, C. Zhang, G.-B. Lou, Y.-C. Wang, L.-L. Wang, Assess the fire resistance of intumescent coatings by equivalent constant thermal resistance. Fire Technol. 48, 529–546 (2012). https://doi.org/10.1007/s10694-011-0243-8
A. Lucherini, L. Giuliani, G. Jomaas, Experimental study of the performance of intumescent coatings exposed to standard and non-standard fire conditions. Fire Saf. J. 95, 42–50 (2018). https://doi.org/10.1016/j.firesaf.2017.10.004
M.R. Manco, M.A. Vaz, J.C.R. Cyrino, A. Landesmann, Behavior of stiffened panels exposed to fire. In: J. Romanoff, C. Guedes Soares (eds.) Anal. Des. Mar. Struct. (CRC Press, 2013), pp. 101–108. https://doi.org/10.1201/b15120-2
M.G. Ryu, K. He, D.H. Lee, S.-I. Park, G. Thomas, J.K. Paik, Finite element modeling for the progressive collapse analysis of steel stiffened-plate structures in fires. Thin-Walled Struct. 159, 107262 (2021). https://doi.org/10.1016/j.tws.2020.107262
J.H. Kim, D.C. Kim, C.K. Kim, M.S. Islam, S.I. Park, J.K. Paik, A study on methods for fire load application with passive fire protection effects. Ocean Eng. 70, 177–187 (2013). https://doi.org/10.1016/j.oceaneng.2013.05.017
M. Friebe, B.-S. Jang, Y. Jim, A parametric study on the use of passive fire protection in FPSO topside module. Int. J. Nav. Archit. Ocean Eng. 6, 826–839 (2014). https://doi.org/10.2478/IJNAOE-2013-0216
M.R. Manco, M.A. Vaz, J.C.R. Cyrino, A. Landesmann, Ellipsoidal solid flame model for structures under localized fire. Fire Technol. 54, 1505–1532 (2018). https://doi.org/10.1007/s10694-018-0750-y
M.R. Manco, M.A. Vaz, J.C.R. Cyrino, A. Landesmann, Evaluation of localized pool fire models to predict the thermal field in offshore topside structures. J. Braz. Soc. Mech. Sci. Eng. 42, 613 (2020). https://doi.org/10.1007/s40430-020-02694-8
M.R. Manco, M.A. Vaz, J.C.R. Cyrino, A. Landesmann, Thermomechanical performance of offshore topside steel structure exposed to localised fire conditions. Mar. Struct. 76, 102924 (2021). https://doi.org/10.1016/j.marstruc.2020.102924
D.S. Simulia, Abaqus 6.12 documentation. Provid Rhode Island, US 2012:6. https://doi.org/10.1097/TP.0b013e31822ca79b.
European committee for standardizaion. EN 1993–1–2: Eurocode 3: Design of steel structures - Part 1–2: General rules - Structural fire design. Br Stand Inst 2005;2.
V. Babrauskas, R.D. Peacock, Heat release rate: the single most important variable in fire hazard. Fire Saf. J. 18, 255–272 (1992). https://doi.org/10.1016/0379-7112(92)90019-9
J.G. Quintiere, Fundamentals of Fire Phenomena (Wiley, New York, 2006)
O. Vassart, B. Zhao, L. Cajot, F. Robert, U. Meyer, A. Frangi, Eurocodes (2014). https://doi.org/10.2788/85432
K. McGrattan, S. Hostikka, R. McDermott, J. Floyd, C. Weinschenk, K. Overholt, Fire Dynamics Simulator, User’s Guide (2015). https://doi.org/10.6028/NIST.SP.1019.
K. McGrattan, S. Hostikka, R. McDermott, J. Floyd, C. Weinschenk, K. Overholt, Fire Dynamics Simulator, Technical Reference Guide, 6.2 edn, vol 1: Mathematical Model, vol 2: Verification Guide, vol 3: Validation Guide (2015).
U. Wickström, Temperature Calculation in Fire Safety Engineering (Springer, New York, 2016)
U. Wickström, S. Hunt, B. Lattimer, J. Barnett, C. Beyler, Technical comment-Ten fundamental principles on defining and expressing thermal exposure as boundary conditions in fire safety engineering. Fire Mater. 42, 985–988 (2018). https://doi.org/10.1002/fam.2660
U. Wickström, J. Anderson, J. Sjöström, Measuring incident heat flux and adiabatic surface temperature with plate thermometers in ambient and high temperatures. Fire Mater. 43, 51–56 (2019). https://doi.org/10.1002/fam.2667
U. Wickström, The adiabatic surface temperature and the plate thermometer. Fire Saf. Sci. (2011). https://doi.org/10.3801/IAFSS.FSS.10-1001
C. Zhang, J.G. Silva, C. Weinschenk, D. Kamikawa, Y. Hasemi, Simulation methodology for coupled fire-structure analysis: modeling localized fire tests on a steel column. Fire Technol. 52, 239–262 (2016). https://doi.org/10.1007/s10694-015-0495-9
G. Heskestad, T. Hamada, Ceiling jets of strong fire plumes. Fire Saf. J. 21, 69–82 (1993). https://doi.org/10.1016/0379-7112(93)90005-B
K.B. McGrattan, H.R. Baum, A. Hamins. NISTIR 6546 Thermal Radiation from Large Pool Fires (2000)
European committee for Standardizaion. EN 1991-1-2: Eurocode 1: Actions on structures—Part 1–2: General actions—Actions on structures exposed to fire. Br Stand Inst 2002.
K.H. Lien, Y.J. Chiou, R.Z. Wang, P.A. Hsiao, Nonlinear behavior of steel structures considering the cooling phase of a fire. J. Constr. Steel Res. 65, 1776–1786 (2009). https://doi.org/10.1016/j.jcsr.2009.03.015
M. Zhou, R.P.R. Cardoso, H. Bahai, A new material model for thermo-mechanical analysis of steels in fire. Int. J. Mech. Sci. 159, 467–486 (2019). https://doi.org/10.1016/j.ijmecsci.2019.05.007
C.K. Iu, S.L. Chan, X.X. Zha, Nonlinear pre-fire and post-fire analysis of steel frames. Eng. Struct. 27, 1689–1702 (2005). https://doi.org/10.1016/j.engstruct.2005.06.003
T.J. Lin, Y.B. Yang, C.W. Huang, Inelastic nonlinear behavior of steel trusses cooled down from a heating stage. Int. J. Mech. Sci. 52, 982–992 (2010). https://doi.org/10.1016/j.ijmecsci.2010.03.014
C. Zhang, G.-Q. Li, A. Usmani, Simulating the behavior of restrained steel beams to flame impingement from localized-fires. J. Constr. Steel Res. 83, 156–165 (2013). https://doi.org/10.1016/j.jcsr.2013.02.001
L.A. Louca, R.M. Mohamed Ali, Improving the ductile behaviour of offshore topside structures under extreme loads. Eng. Struct. 30, 506–521 (2008). https://doi.org/10.1016/j.engstruct.2007.04.020
E. Rizzuto, L. Brubak, G.S. Kim, M. Kõrgesaar, K. Nahshon, A. Nilva, et al., Committee V.1: Accidental Limit States. Proc. 20th Int. Sh. Offshore Struct. Congr. (ISSC 2018) - Spec. Comm. Reports (2018). https://doi.org/10.3233/978-1-61499-864-8-1
B.K. Dhurandher, R. Kumar, A.K. Dhiman, A. Gupta, P.K. Sharma, An experimental study of vertical centreline temperature and velocity profile of buoyant plume in cubical compartment. J. Braz. Soc. Mech. Sci. Eng. 39, 1813–1822 (2017). https://doi.org/10.1007/s40430-016-0629-0
J. Casal, Evaluation of the effects and consequences of major accidents in industrial plants. Ind. Saf. Ser. 8, 345–346 (2008). https://doi.org/10.1016/S0921-9110(08)80011-0
F.D.S. Santos, A. Landesmann, Thermal performance-based analysis of minimum safe distances between fuel storage tanks exposed to fire. Fire Saf. J. 69, 57–68 (2014). https://doi.org/10.1016/j.firesaf.2014.08.010
M. Muñoz, E. Planas, F. Ferrero, J. Casal, Predicting the emissive power of hydrocarbon pool fires. J. Hazard Mater. 144, 725–729 (2007). https://doi.org/10.1016/j.jhazmat.2007.01.121
K.S. Mudan, Thermal radiation hazards from hydrocarbon pool fires. Prog. Energy Combust. Sci. 10, 59–80 (1984). https://doi.org/10.1016/0360-1285(84)90119-9
Acknowledgements
The authors wish to express their gratitude to the National Agency of Petroleum, Natural Gas and Biofuels of Brazil (ANP) and National Council of Scientific and Technological Development of Brazil (CNPq) for their support to the development of this work.
Author information
Authors and Affiliations
Contributions
MMR: Conceptualization, Methodology, Software, Investigation, Formal analysis, writing original draft. MAV: Conceptualization, Investigation, Formal analysis, Writing—review & editing. JCRC: Conceptualization, Investigation, Formal analysis, Writing—review & editing. AL: Conceptualization, Investigation, Formal analysis, Writing—review & editing.
Corresponding author
Ethics declarations
Conflict of interest
No competing interests.
Additional information
Publisher's Note
Springer Nature remains neutral with regard to jurisdictional claims in published maps and institutional affiliations.
Annex I: LF-ESF model
Annex I: LF-ESF model
The model is limited to cases where the height of the flame (\({H}_{fl(t)}\) [m]) estimated according to the Heskestad model [28]) is less than the height of the compartment \({H}_{c}\) (\({H}_{ {\text{fl}} }<{H}_{\rm{c}}\)).
1.1 Convective heat flux
According to Heskestad & Hamada—H&H [28] the plume centerline temperature \({\Delta \theta }_{o}\) [oC] is calculated according to Eq. (A.2) [28, 30, 38].
where \({\Delta \theta }_{\rm{o}}\) is at most 900 °C [30], \({\chi }_{\rm{r}}\) is the radiative fraction of the \( {\text{HRR}}\) (taken as 0.2 in [30]), \(z\) [m] is the height of the selected point, \({z}_{\rm{o}}\) [m] is the height of the virtual source calculated from Eq. (A.3) [32]:
LF-ESF model proposes a modification of the H&H [28] gas temperature distribution as shown in Eq. (A.4).
where \(r\)[m] is the radial distance to flame axis, \(\stackrel{-}{r}\)[-] is the normalized distance to the flame axis (\(\stackrel{-}{r}=r/(D/2))\), \({\stackrel{-}{H}}_{ {\text{fl}} }\,[-]\) is the normalized height (\({\stackrel{-}{H}}_{ {\text{fl}} }=z/{H}_{ {\text{fl}} }\)), \(z\)[m] is the height where the temperature is evaluated and a, b are coefficient that are equal to − 1 y 1, respectively when \({\stackrel{-}{H}}_{ {\text{fl}} }\ge 1\) and 0 otherwise.
1.2 Radiant heat flux
LF-ESF model proposes the use of a flame with an ellipsoidal form to improve the representation of the radiant flux, as described in the Eq. (A.5):
The thermal radiant intensity to an element outside the flame envelope is given by the Eq. (A.6):
where \({F}_{ij}\)[-] is the geometric view factor, \(\tau \) [-] is the atmospheric transmissivity [39] and \({E}_{\rm{a}\rm{v}}\)[kW/m2] is the flame average emissive power.
This methodology describes the atmospheric transmissibility according to Casal [39] as a function of the distance \(d\) [m] between the flame and target as shown in Eq. (A.7) [40]:
The MSFM [41] considers the flame average emissive power \({E}_{\rm{a}\rm{v}}\)[kW/m2] (140 kW/m2 for hydrocarbon fires [1]) as a correlation between flame emissive power (\({E}_{ {\text{fl}} }={E}_{\rm{b}}{\varepsilon }_{ {\text{fl}} }\) [kW/m2]) and thermal radiation emitted by smoke \({E}_{\rm{s}\rm{o}\rm{o}\rm{t}}\) [kW/m2] (20 kW/m2 [1, 42]), as shown in Eq. (A.8):
where \({\chi }_{\rm{l}\rm{u}\rm{m}}\) [−] is the percentage of visible flame (0.80 and 0.20 for gasoline and ethanol, respectively [42]), \({\varepsilon }_{ {\text{fl}} }\,[-]\) is the flame emissivity (taken as 1 for simplicity), \({E}_{\rm{b}}\)[kW/m2] is the black body emissive power calculated following the Eq. (A.8.a):
where \({\theta }_{ {\text{fl}} }\) [K] is the flame's radiation temperature and \({\theta }_{\infty }\) [K] is the environmental temperature (assumed to be constant and equal to 293 K).
From Eq. (A.6) and Eq. (A.8) the equivalent flame temperature (\({\theta }_{ {\text{fl}} ,\rm{e}\rm{q}}\) [K]), assumed uniform, can be calculated as:
Rights and permissions
About this article
Cite this article
Manco Rivera, M.R., Vaz, M.A., Cyrino, J.C.R. et al. A study on the use of fireproof protection in offshore topside steel structures subject to localised fires. Mar Syst Ocean Technol 16, 55–68 (2021). https://doi.org/10.1007/s40868-021-00102-x
Received:
Accepted:
Published:
Issue Date:
DOI: https://doi.org/10.1007/s40868-021-00102-x