Abstract
A novel hybrid configuration of solar parabolic trough collectors–waste incineration power plant was recently analyzed energetically in Denmark. Taking into account the true meaning of sustainability which is environmental friendliness and cost-effectiveness, and considering the existing gap of knowledge on the thermodynamic performance aspects of this hybrid system, this work conducts a thorough thermodynamic and sustainability analysis of this power plant. The main aim is to give a clear picture of the main advantages and any possible shortcomings of the hybrid power plant. For this purpose, the performance of the system is simulated for an entire year of operation under realistic solar irradiation fluctuations. The energy performance indices of the system are quantified and discussed. The exergy assessment of the hybrid cycle is accomplished, and the main sources of exergy destruction and economic losses are identified. The results show that the steam generator and the turbine cause the largest rates of irreversibilities of 36% and 20.8%. The environmental benefits and the overall cost of energy production of the system are calculated and compared to some other alternative power plants. In addition to the consistency of electricity production, the LCOE of the hybrid power plant decreases by 67% in comparison with the solar power plant. Comparing the system with a natural gas-fired power plant in terms of CO2 emission, it is shown that the hybrid system leads to less 74.5 thousand tonnes of CO2 emitted over an entire year.
























Similar content being viewed by others
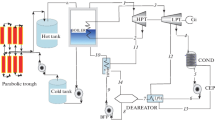
Abbreviations
- A :
-
Area (m)2
- D :
-
Diameter (m)
- \(E_{{{\text{CO}}2{\text{e}}}}\) :
-
Amount of CO2e emission (–)
- \(\dot{E}_{\text{gen}}\) :
-
Electricity power (kW)
- E λ :
-
Emission of different types of greenhouse gases (–)
- Ex:
-
Exergy (kW)
- GWPλ :
-
Global warming potential of greenhouse gases (–)
- h :
-
Enthalpy (kJ kg−1)
- \(h_{\text{c}}\) :
-
Convective heat transfer coefficient (kJ m−2K−1)
- \(I{{\$ }}_{{\rm t}}\) :
-
Installation fees (\({{\$ }}\))
- \({\text{IAM}}\) :
-
Incidence angle modifier (–)
- K :
-
Thermal conductance (kJ m−1K−1)
- \({\text{LHV}}\) :
-
Low heat value (kJ kg−1)
- \(M{{\$}}\) :
-
Maintenance fees ($)
- \(\dot{m}\) :
-
Mass flow rate of the first preheating line (kg s−1)
- \(\dot{m}_{\text{PTC}}\) :
-
Mass flow rate solar field (kg s−1)
- \(\dot{m}_{\text{s}}\) :
-
Total mass flow rate of steam (kg s−1)
- Nu:
-
Nusselt Number (–)
- \(O{{\$ }}_{{\rm t}}\) :
-
Operation fees (\({{\$ }}\))
- P :
-
Pressure (kPa)
- Pr:
-
Prandtl number (–)
- \(q_{{12{\text{Conv}}}}^{ '}\) :
-
Convective heat transfer between SHTF and the absorber (kJ m−1)
- \(q_{{23{\text{Cond}}}}^{ '}\) :
-
Conductive heat transfer through the absorber wall (kJ m−1)
- \(q_{{34{\text{Rad}}}}^{ '}\) :
-
Heat transfer from the absorber to the glass envelope (kJ m−1)
- \(q_{{3{\text{SolAbs}}}}^{ '}\) :
-
Solar Heat absorbed by the absorber (kJ m−1)
- \(q_{{45{\text{Cond}}}}^{ '}\) :
-
Conductive heat transfer through the glass envelope (kJ m−1)
- \(q_{{56{\text{Conv}}}}^{ '}\) :
-
Convective Heat transfer from the glass envelope to the atmosphere (kJ m−1)
- \(q_{{56{\text{Rad}}}}^{ '}\) :
-
Radiative Heat transfer from the glass envelope to the atmosphere (kJ m−1)
- \(q_{{57{\text{Rad}}}}^{ '}\) :
-
Radiative Heat transfer from the glass envelope to the sky (kJ m−1)
- \(q_{{5{\text{SolAbs}}}}^{ '}\) :
-
Solar absorption in the glass envelope (kJ m−1)
- \(q_{\text{cond}}^{ '}\) :
-
Heat rejected in condenser (kJ kg−1)
- \(q_{{{\text{Cond}},{\text{bracket}}}}^{ '}\) :
-
Conductive heat transfer through the bracket support (kJ m−1)
- \(q_{\text{si}}^{ '}\) :
-
Solar irradiation per receiver length (kJ m−1)
- \(\dot{Q}_{\text{cond}}\) :
-
Rate of heat rejected in condenser (kW)
- \(\dot{Q}_{\text{WI}}\) :
-
Heat released in waste incineration process (kW)
- T :
-
Temperature (K)
- y :
-
The flow rate of steam withdrawals form the first turbine (–)
- y′:
-
The flow rate of steam withdrawals form the second turbine (–)
- \(\dot{W}\) :
-
Work production rate (kW)
- \({{\$ }}_{{\rm MSW}}\) :
-
Annual cost of the municipal waste ($)
- C, H, O, N, S:
-
Mass fraction of carbon, hydrogen, oxygen, nitrogen and sulfur
- \(\alpha_{\text{abs}}\) :
-
Absorptance of the absorber
- α env :
-
Absorptance of the envelope
- \(\varepsilon\) :
-
Emittance
- \(\varepsilon x\) :
-
Exergy efficiency
- \(\varepsilon_{1}^{ '}\) :
-
Shadowing factor
- \(\varepsilon_{2}^{ '}\) :
-
Tracking error
- \(\varepsilon_{3}^{ '}\) :
-
Geometry error (mirror alignment)
- \(\varepsilon_{4}^{ '}\) :
-
Dirt on mirrors
- \(\varepsilon_{5}^{ '}\) :
-
Dirt on collector
- \(\varepsilon_{6}^{ '}\) :
-
Unaccounted factor
- \(\eta\) :
-
Efficiency
- \(\eta_{\text{abs}}\) :
-
Effective optical efficiency at absorber
- η env :
-
Effective optical efficiency at the glass envelope
- \(\eta_{\text{gen}}\) :
-
Electricity generation efficiency
- ξ :
-
Exhaust gas volume
- \(\eta_{\text{WI}}\) :
-
Thermal efficiency of waste incineration plant
- τ :
-
Transmittance
- A:
-
Air
- abs:
-
Absorber
- c:
-
Cover
- ci:
-
Cover inner
- CFWH:
-
Closed feed water heater
- ch:
-
Chemical
- conv:
-
Convection
- CT:
-
Cooling Tower
- De:
-
Destruction
- env:
-
Envelope
- FG:
-
Flue gas
- G:
-
Generator
- gen:
-
Generation
- HC:
-
Hybrid cycle
- \({\text{HPT}}\) :
-
High-pressure turbine
- \({\text{IPT}}\) :
-
Intermittent pressure turbine
- \({\text{LPT}}\) :
-
Low-pressure turbine
- MC:
-
Mixing chamber
- MSW:
-
Municipal solid waste
- Net:
-
Net
- OFWH:
-
Open feed water heater
- opt:
-
Optical
- P:
-
Pump
- PH:
-
Preheater
- PTC:
-
Parabolic trough collector
- Rad:
-
Radiation
- RC:
-
Rankine cycle
- s:
-
Steam
- SF:
-
Solar fluid
- SG:
-
Steam generator
- Sol:
-
Solar
- ST:
-
Steam turbine
- WI:
-
Waste incineration
References
Plis A, Kotyczka-Morańska M, Kopczyński M, Łabojko G. Furniture wood waste as a potential renewable energy source. J Therm Anal Calorim. 2016;125:1357–71.
Senturk Acar M, Arslan O. Energy and exergy analysis of solar energy-integrated, geothermal energy-powered Organic Rankine Cycle. J Therm Anal Calorim. 2019;137:659–66.
Peng Y, Zahedidastjerdi A, Abdollahi A, Amindoust A, Bahrami M, Karimipour A, et al. Investigation of energy performance in a U-shaped evacuated solar tube collector using oxide added nanoparticles through the emitter, absorber and transmittal environments via discrete ordinates radiation method. J Therm Anal Calorim. 2019;20:1–9.
Jeon J, Lee J-H, Seo J, Jeong S-G, Kim S. Erratum to: application of PCM thermal energy storage system to reduce building energy consumption. J Therm Anal Calorim. 2014;116:539.
Guerrero-Lemus R, Martínez-Duart JM. Renewable energies and CO2: cost analysis, environmental impacts and technological trends—2012 edition. In: Guerrero-Lemus R, Martínez-Duart JM, editors. London: Springer; 2013. p. 307–33.
Mousavi GSM, Faraji F, Majazi A, Al-Haddad K. A comprehensive review of Flywheel Energy Storage System technology. Renew Sustain Energy Rev. 2017;67:477–90.
Rehman S, Al-Hadhrami LM, Alam MM. Pumped hydro energy storage system: a technological review. Renew Sustain Energy Rev. 2015;44:586–98.
Arabkoohsar A, Andresen GBB. Design and analysis of the novel concept of high temperature heat and power storage. Energy. 2017;126:21–33. https://doi.org/10.1016/j.energy.2017.03.001.
Bagherzadeh SA, Ruhani B, Namar MM, Alamian R, Rostami S. Compression ratio energy and exergy analysis of a developed Brayton-based power cycle employing CAES and ORC. J Therm Anal Calorim. 2020;139:2781–90.
Nouri M, Namar MM, Jahanian O. Analysis of a developed Brayton cycled CHP system using ORC and CAES based on first and second law of thermodynamics. J Therm Anal Calorim. 2019;135:1743–52.
Romanelli F. Strategies for the integration of intermittent renewable energy sources in the electrical system. Eur Phys J Plus. 2016;131:53.
Akbari Vakilabadi M, Bidi M, Najafi AF, Ahmadi MH. Energy, Exergy analysis and performance evaluation of a vacuum evaporator for solar thermal power plant zero liquid discharge systems. J Therm Anal Calorim. 2019;20:1–6.
Achour L, Bouharkat M, Behar O. Performance assessment of an integrated solar combined cycle in the southern of Algeria. Energy Rep. 2018;4:207–17.
Liu T, Liu Q, Wang X, Sui J, Jin H. Performance investigation of a new solar-hybrid fuel-fired distributed energy system integrated with a thermochemical process. Energy Procedia. 2017;142:815–21.
Pärisch P, Mercker O, Warmuth J, Tepe R, Bertram E, Rockendorf G. Investigations and model validation of a ground-coupled heat pump for the combination with solar collectors. Appl Therm Eng. 2014;62:375–81.
Basir Khan MR, Jidin R, Pasupuleti J, Shaaya SA. Optimal combination of solar, wind, micro-hydro and diesel systems based on actual seasonal load profiles for a resort island in the South China Sea. Energy. 2015;82:80–97.
Li P, Li J, Pei G, Munir A, Ji J. A cascade organic Rankine cycle power generation system using hybrid solar energy and liquefied natural gas. Sol Energy. 2016;127:136–46.
Kasaeian A, Nouri G, Ranjbaran P, Wen D. Solar collectors and photovoltaics as combined heat and power systems: a critical review. Energy Convers Manag. 2018;156:688–705.
Haddad A, Ramadan M, Khaled M, Ramadan H, Becherif M. Study of hybrid energy system coupling fuel cell, solar thermal system and photovoltaic cell. Int J Hydrogen Energy. 2018 (in press), https://doi.org/10.1016/j.ijhydene.2018.06.019.
Arabkoohsar A, Andresen GBB. Supporting district heating and cooling networks with a bifunctional solar assisted absorption chiller. Energy Convers Manag. 2017;148:184–96.
Liu M, Steven Tay NH, Bell S, Belusko M, Jacob R, Will G, et al. Review on concentrating solar power plants and new developments in high temperature thermal energy storage technologies. Renew Sustain Energy Rev. 2016;53:1411–32.
Cavallaro F, Zavadskas EK, Streimikiene D. Concentrated solar power (CSP) hybridized systems. Ranking based on an intuitionistic fuzzy multi-criteria algorithm. J Clean Prod. 2018;179:407–16.
McTigue JD, Castro J, Mungas G, Kramer N, King J, Turchi C, et al. Hybridizing a geothermal power plant with concentrating solar power and thermal storage to increase power generation and dispatchability. Appl Energy. 2018;228:1837–52.
Jin HG, Hong H. 12—Hybridization of concentrating solar power (CSP) with fossil fuel power plants. In: Lovegrove K, Stein WBT-CSPT, editors. Woodhead Publishing Series Energy. Sawston: Woodhead Publishing; 2012.
Behar O. Solar thermal power plants—a review of configurations and performance comparison. Renew Sustain Energy Rev. 2018;92:608–27.
Arvelakis S, Frandsen FJ, Dam-Johansen K. Determining the melting behaviour of ashes from incineration plants via thermal analysis. J Therm Anal Calorim. 2003;72:1005–17.
Łach M, Mikuła J, Hebda M. Thermal analysis of the by-products of waste combustion. J Therm Anal Calorim. 2016;125:1035–45.
European Commission. The role of waste-to-energy in the circular economy. Commun From Comm To Eur Parliam Counc Eur Econ Soc Comm Comm Reg [Internet]. 2017;11. https://ec.europa.eu/environment/waste/waste-to-energy.pdf.
Münster M, Meibom P. Optimization of use of waste in the future energy system. Energy. 2011;36:1612–22.
Eriksson O, Finnveden G, Ekvall T, Björklund A. Life cycle assessment of fuels for district heating: a comparison of waste incineration, biomass- and natural gas combustion. Energy Policy. 2007;35:1346–62.
Udono K, Sitte R. Modeling seawater desalination powered by waste incineration using a dynamic systems approach. Desalination. 2008;229:302–17.
Hedberg E, Project D. Potential for Absorption Cooling Generated from Municipal Solid Waste in Bangkok, Degree Project, Department of Management and Engineering, Linkopings University.
Sadi M, Arabkoohsar A. Modelling and analysis of a hybrid solar concentrating-waste incineration power plant. J Clean Prod. 2019;216:570–84.
Arabkoohsar A, Sadi M. A hybrid solar concentrating-waste incineration power plant for cost-effective and dispatchable renewable energy production. In: 2018 IEEE 7th International Conference Power Energy, PECon 2018; 2019.
Dickes R, Lemort V, Quoilin S. Semi-empirical correlation to model heat losses along solar parabolic trough collectors; 2015, 28th international conference on efficiency, cost, optimization, simulation and environmental impact of energy systems, Pau, France.
Jack TA, Oko COC. Exergy and exergoeconomic analysis of a municipal waste-to-energy steam reheat power plant for Port Harcourt city. Int J Ambient Energy. 2018;39:352–9.
Song G, Shen L, Xiao J. Estimating specific chemical exergy of biomass from basic analysis data. Ind Eng Chem Res. 2011;50:9758–66.
Dudley VE, Kolb GJ, Mahoney AR, Mancini TR, Matthews CW, Sloan M, et al. Test results: SEGS LS-2 solar collector. Sandia Natl. Lab.; 1994.
Arabkoohsar A, Andresen GB. Thermodynamics and economic performance comparison of three high-temperature hot rock cavern based energy storage concepts. Energy. 2017;132:12–21.
Incropera FP, Bergman TL, Lavine AS, DeWitt DP. Fundamentals of heat and mass transfer, 8th Edition, ISBN: ES8-1-119-32042-5, [Internet]. Wiley. 2018. https://www.wiley.com/en-us/Fundamentals+of+Heat+and+Mass+Transfer%2C+8th+Edition-p-ES81119320425.
Touloukian YS, DeWitt DP. Thermal radiative properties. Nonmetallic solids; 1972. NASA, United States.
Yang H-C, Lee S-Y, Choi Y-C, Yang I-H, Chung DY. Thermokinetic analysis of spent ion-exchange resins for the optimization of carbonization reactor condition. J Therm Anal Calorim. 2017;127:587–95.
Javadi MA, Hoseinzadeh S, Ghasemiasl R, Heyns PS, Chamkha AJ. Sensitivity analysis of combined cycle parameters on exergy, economic, and environmental of a power plant. J Therm Anal Calorim. 2020;139:519–25.
Petela K, Szlek A. Energy and exergy analysis of solar heat driven chiller under wide system boundary conditions. Energy. 2019;168:440–9.
Padilla RV, Fontalvo A, Demirkaya G, Martinez A, Quiroga AG. Exergy analysis of parabolic trough solar receiver. Appl Therm Eng. 2014;67:579–86.
Carneiro MLNM, Gomes MSP. Energy, exergy, environmental and economic analysis of hybrid waste-to-energy plants. Energy Convers Manag. 2019;179:397–417.
US Environmental Protection Agency (USEPA) [Internet]. https://www.wbdg.org/FFC/EPA/EPACRIT/epa625_6_89_021.pdf.
Johnke B, Hoppaus R, Lee E, Irving B, Martinsen TMK. Good practice guidance and uncertainty management in national greenhouse gas inventories. 2000. Intergovernmental Panel on Climate Change.
Arabkoohsar A, Andresen GB. A smart combination of a solar assisted absorption chiller and a power productive gas expansion unit for cogeneration of power and cooling. Renew Energy. 2018;115:489–500.
Dudley V, Kolb G, Sloan M and KD. SEGS LS2 Solar Collector-Test Results. Rep. Sandia Natl. Lab. SSAN94-1884; 1996.
Author information
Authors and Affiliations
Corresponding author
Additional information
Publisher's Note
Springer Nature remains neutral with regard to jurisdictional claims in published maps and institutional affiliations.
Rights and permissions
About this article
Cite this article
Arabkoohsar, A., Sadi, M. Thermodynamics, economic and environmental analyses of a hybrid waste–solar thermal power plant. J Therm Anal Calorim 144, 917–940 (2021). https://doi.org/10.1007/s10973-020-09573-3
Received:
Accepted:
Published:
Issue Date:
DOI: https://doi.org/10.1007/s10973-020-09573-3