Highlights
-
Various synthetic methods for the synthesis of NiCo2O4 nano-/microstructures in bare, doped, and composite/hybrid forms are reviewed.
-
Currents status and development prospects of NiCo2O4 nano-/microstructure-based electrochemical biosensors for bioanalytes such as glucose, urea, and H2O2, along with condition governing the electrochemical biosensor parameters, are summarized.
-
Also provide an insight into the key challenges and future perspectives about point-of-care monitoring of bioanalytes using NiCo2O4 nano-/microstructure-based biosensors.
Abstract
Non-enzymatic biosensors based on mixed transition metal oxides are deemed as the most promising devices due to their high sensitivity, selectivity, wide concentration range, low detection limits, and excellent recyclability. Spinel NiCo2O4 mixed oxides have drawn considerable attention recently due to their outstanding advantages including large specific surface area, high permeability, short electron, and ion diffusion pathways. Because of the rapid development of non-enzyme biosensors, the current state of methods for synthesis of pure and composite/hybrid NiCo2O4 materials and their subsequent electrochemical biosensing applications are systematically and comprehensively reviewed herein. Comparative analysis reveals better electrochemical sensing of bioanalytes by one-dimensional and two-dimensional NiCo2O4 nano-/microstructures than other morphologies. Better biosensing efficiency of NiCo2O4 as compared to corresponding individual metal oxides, viz. NiO and Co3O4, is attributed to the close intrinsic-state redox couples of Ni3+/Ni2+ (0.58 V/0.49 V) and Co3+/Co2+ (0.53 V/0.51 V). Biosensing performance of NiCo2O4 is also significantly improved by making the composites of NiCo2O4 with conducting carbonaceous materials like graphene, reduced graphene oxide, carbon nanotubes (single and multi-walled), carbon nanofibers; conducting polymers like polypyrrole (PPy), polyaniline (PANI); metal oxides NiO, Co3O4, SnO2, MnO2; and metals like Au, Pd, etc. Various factors affecting the morphologies and biosensing parameters of the nano-/micro-structured NiCo2O4 are also highlighted. Finally, some drawbacks and future perspectives related to this promising field are outlined.

Similar content being viewed by others
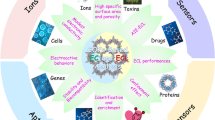
1 Introduction
Recently, spinel single-phase binary metal oxides containing two metal cations such as manganese cobaltate (MnCo2O4) [1], zinc cobaltate (ZnCo2O4) [2, 3], nickel ferrite (NiFe2O4) [4], copper manganate (CuMn2O4) [5], copper cobaltate (CuCo2O4) [6], cobalt manganate (CoMn2O4) [7], nickel cobaltate (NiCo2O4) [8] have attracted widespread attention from researchers worldwide due to their invariably better electrochemical properties as compared to individual metal oxides or a mixture of metal oxides. The excellent electrochemical performances of these single-phase binary metal oxides are attributed to the synergetic effects of properties of the individual metal oxide components [9]. Among various such single-phase binary metal oxides, NiCo2O4 is considered to be the best one as it possesses at least two times higher electronic conductivity as compared to corresponding individual metal oxides, viz. NiO and Co3O4 along with intrinsic-state redox couples of Ni3+/Ni2+ (0.58 V/0.49 V) and Co3+/Co2+ (0.53 V/0.51 V) [10,11,12]. Other key features are the exhibition of variable but sufficiently stable oxidation states by Ni (Ni2+, Ni3+) and Co (Co2+, Co3+, Co4+) and very high conductivity of 500 S cm−1 [13, 14].
Many transition metals, rare earth metals, non-metal-doped NiCo2O4, and conjugated polymer-modified NiCo2O4 materials have been reported in the literature with versatile applications. N- and P-doped NiCo2O4 with oxygen vacancies have been explored for electrochemical performance for supercapacitors, electro-catalyst for O2 and H2 evolution reaction [15,16,17,18], and anodic material for lithium-ion batteries [19]. Lin et al. [20] explored S-doped NiCo2O4 nanosheet arrays as the efficient and bifunctional electrode for overall water-splitting reactions. Compared with non-metal-doped NiCo2O4, transition metal and rare earth metal-doped NiCo2O4 are considered superior due to the latter’s excellent electrical conductivity. Zn- and Fe-doped NiCo2O4 showed electrocatalytic properties for oxygen evolution reactions and remarkable capacitive properties in asymmetric supercapacitors [21,22,23]. Ma et al. [24] synthesized highly porous hierarchical spinel Mn-doped NiCo2O4 nanosheets for high-performance anodes in lithium-ion batteries. Xia et al. [25] used Au–NiCo2O4 nanomaterials supported on 3D hierarchical porous graphene-like material as electro-catalyst for oxygen evolution reaction. Among the rare earth metal oxides, CeO2 is reported to be an excellent dopant for NiCo2O4 nanomaterials [26, 27]. Carbonaceous and polymer composite/hybrid NiCo2O4 nano-/microstructures are also found suitable for their potential applications in supercapacitors [28], fuel cells [29], Li-ion batteries [30], electro-catalyst for oxygen reduction reaction and oxygen evolution reaction [31], photo-detector [32], optoelectronic devices [33], perovskite solar cells [34], gas sensors [35,36,37] and biosensors [38, 39].
Facile, low-cost and eco-friendly synthetic methods lead to varieties of low dimensional nano-/micro-structured morphologies with excellent porosity and specifically large surface area, opportunities to synthesize composite/hybrid and ease of electrode fabrications for end-user applications. Spinel NiCo2O4 is a p-type semiconductor in which Ni occupies octahedral sites while Co is distributed in both octahedral and tetrahedral sites [13] (Fig. 1a, b). It shows a face-centered cubic arrangement and belongs to Fd3m space group with lattice constant ao = 8.269 Å [40].
Electrochemical sensing through miniaturized sensors based on nano-/micro-structured materials has taken over the conventional, expensive, laborious sensing techniques like lateral flow immunoassay, liquid chromatography, capillary electrophoresis, enzyme-linked immunosorbent assay, chemiluminescence, sequential injection analysis, gas chromatography–mass spectrometry and fluorescent methods [43,44,45,46,47,48]. Electrochemical biosensors can be categorized into amperometric and potentiometric sensors [49]. The amperometric biosensing involves a change in current response due to electrochemical redox reactions of the analytes when a potential is applied between the working and reference electrodes while the potentiometric biosensing makes use of ion-selective electrodes to transduce the biological reactions into a measurable electrical signal [43, 50].
Among the main classes of biosensors, the non-enzymatic biosensor is considered to be better, faster, and more convenient as compared to an enzymatic biosensor that involves complicated and multi-step enzyme immobilization processes and high specificity of the enzymes. Also, due to pH and temperature sensitiveness, the enzyme-based biosensors are highly unstable as enzymes undergo denaturation leading to biological inactivity beyond physiological conditions [51,52,53]. Nanomaterials not only provide high-density catalytic sites for the electro-oxidation or electro-reduction in the biomarkers but also provide large surface area for adsorption of biomarkers and facilitate an appropriate path for electron transport for electrochemical activity [54,55,56]. Since the crucial part in electrochemical biosensors is the modified electrode, much attention has been devoted to modulate the electrocatalytic behavior of the NiCo2O4 as electron mediator by engineering its composition, structure, specific surface area, and redox properties.
To date, many reviews have been reported for the applications of NiCo2O4 nano-/micro-structured materials including Li-ion batteries [10], supercapacitors [11, 57], fuel cells [58], and electro-catalyst for oxygen reduction, oxygen and hydrogen evolution reactions [59, 60]. The applications of the NiCo2O4-based non-enzymatic biosensors are aimed not only at the extension of the spectrum of target bioanalytes but also at the improvement in the biosensor performance in terms of sensitivity, selectivity, detection limits, long-term stability as well as reusability. Many new synthetic strategies and techniques have been developed for the fabrication of NiCo2O4-based non-enzymatic biosensors, but they are rarely summarized. Hence, it is an appropriate time to go through the periodical progress of NiCo2O4-based non-enzymatic biosensors. This review covers the crystal structure of the spinel NiCo2O4, various synthetic strategies employed for the synthesis of nano-/micro-structured NiCo2O4, electrochemical biosensing toward biomarkers such as glucose, H2O2, and urea, through the fabrication of modified electrodes. Various factors affecting the morphologies and biosensing parameters of the nano-/micro-structured NiCo2O4 are also reviewed.
2 General Biosensing Mechanism
Two types of strategies are generally involved in the electrochemical biosensing of biomarkers, i.e., enzyme based and enzyme-free [61, 62]. An enzymatic biosensor operates on three main components which include sensitive recognition element, signal transducer element, and data evaluation component [63,64,65,66]. Enzymes, antibodies, and nucleic acid are generally used as recognition components. Glucose oxidase and glucose dehydrogenase for glucose [67, 68], horseradish peroxidase for H2O2 [69], urease for urea [70], laccase and polyphenol oxidase for rutin [71], tryptophan oxidase for tryptophan [72], etc. act as sensitive recognition elements. The function of the signal transducer is to convert chemical changes into detectable and readable electronic signals which are finally transferred to the data evaluation component. Recent developments in the field of nanotechnology and nanoscience reveal the excellent efficiencies of the nanostructured materials as signal transducers. Biosensors based on nanostructured materials as artificial bioreceptors are used for early detection and diagnosis of diseases through the estimation of the levels of biomarkers [73,74,75]. The signal transducer behavior of the nanomaterials mainly depends upon the electrochemical redox properties, surface-to-volume ratio, crystal structure and phase, morphology, and the presence of some other conducting matrices along with the nanostructured materials [76,77,78]. In contrast, in enzyme-free biosensors, nanostructured materials are used as signal transducers as well as sensitive recognition elements.
Electrochemical biosensors are mainly based on the output electrical signals changes incurred from either the oxidation or the reduction of the target bioanalyte on the surface of the transducer (Fig. 2) [79,80,81]. These redox reactions are catalyzed by signal transducer enzymes and nanostructured materials in enzyme-based and enzyme-free biosensors, respectively. The strength of the electrical signals is significantly affected by the concentrations of target bioanalytes, temperature, pH, and the presence of the interfering species [82,83,84,85].
3 Synthesis of Nano-/Micro-Structured NiCo2O4
3.1 Hydrothermal/Solvothermal Method
Hydrothermal synthesis involves heterogeneous reactions in an aqueous medium within a temperature range of 100–200 °C and high pressure. To achieve these conditions, the reaction is usually carried out in Teflon-lined sealed steel autoclaves. Alkali metal hydroxide or NH3 is added to convert the precursor metal salts into their respective hydroxides at basic pH conditions [86, 87]. An initial nucleation phase is followed by the directed crystal growth along appropriate crystal planes. The morphology, surface, and the structural features of the materials synthesized through hydrothermal method depend upon the conditions like temperature, pH of the solution, concentration of the precursor, nature of the solvent, and the presence of the templates [88]. NiCo2O4 nano-/microstructures of various shapes and morphologies have been prepared hydrothermally. Nano-/micro-structured NiCo2O4 of morphologies such as urchin shaped [89], coral-like [90], core–ring-structured nanoplatelets [91], porous coral-like nanospheres [36], hollow nanospheres [92], nanospheres [93], urchin-like spheres [94], mesoporous nanoparticles [95], mesoporous nanoneedles [96, 97], 3D network-like mesoporous nanostructures [98], 3D hierarchical tremella-like, flower-like, urchin-like and pine needle-like [99], nanoflakes [100], nanowalls [101], etc. are reported.
Ni and Co precursor salt solutions with molar atomic ratio of 1:2 are taken during hydrothermal growth since Ni and Co atoms are present in the 1:2 atomic ratio. Liu et al. [94] used 1 mmol Ni(NO3)2·6H2O and 2 mmol Co(NO3)2·6H2O solution to prepare urchin-like NiCo2O4 spheres. Yang et al. [102] mixed 1 mmol of Ni(CH3COO)2·4H2O and 2 mmol of Co(CH3COO)2·4H2O for the preparation of NiCo2O4 nanospheres. Yu et al. [96] used 0.5 mmol Ni(NO3)2·6H2O, 1 mmol Co(NO3)2·6H2O for the synthesis of NiCo2O4 mesoporous nanoneedles. Zhu et al. [98] mixed 0.225 mmol of Ni(CH3COO)2·4H2O and 0.45 mmol of Co(CH3COO)2·4H2O for the synthesis of 3-D network-like mesoporous nanostructures. For the initial formation of binary metal hydroxides or metal carbonate hydroxides, reagents like NH3, urea, NaOH, NH4HCO3, NH4F, hexamethylenetetramine (HMTA) [103], diethylene glycol (DEG), cetyltrimethylammonium bromide (CTAB) [104], sodium dodecyl sulfate (SDS) [105], poly(diallyldimethylammonium chloride) (PDDA) [106], glycine [107], methyl glycerate [108], and ethylene glycol are added in the reaction mixture. The combination of some polar solvents such as ethanol, ethanol, propanol, ethylene glycol, and acetone along with water has also been found to facilitate the morphological characteristics [109]. Water:polar solvent ratio also significantly affects the growth mechanism. In Fig. 3a–d, different morphologies for the NiCo2O4 nanostructures are shown for water:ethanol ratios 1:0, 3:1, 1:1, and 1:3. More porous, denser, and thinner sheets were formed for the synthesized 3D flower-like NiCo2O4 nanostructures as the composition of ethanol was increased.

Reproduced with permission from Ref. [109]. Copyright © 2017 Elsevier B.V.
FESEM image of NiCo2O4 samples using water: ethanol ratios a 1:0, b 3:1, c 1:1, and d 1:3.
In the hydrothermal growth, the temperature is also a key factor in controlling the morphology of the nanostructures. Urchin- and sheaf-like NiCo2O4 nanostructures were synthesized by Umeshbabu et al. [104] using CTAB as a surfactant under hydrothermal conditions at 120 °C and 200 °C temperatures, respectively. Different morphologies were attributed to different degrees of crystal splitting and anisotropic crystal growth at different growth temperatures [110]. Further, the temperature also affects the magnitude of the van der Waals forces, hydrogen bonding, hydrophobic attraction, crystal field attraction, and intrinsic crystal contraction which subsequently control the Ostwald ripening process [111, 112].
Nayak et al. [89] mixed Ni(NO3)2·6H2O and Co(NO3)2·6H2O salts in a 1:2 atomic ratio along with urea which produced OH− ions in the reaction mixture according to Eqs. 1–3.
Ni2+ and Co2+ on reaction with these OH− ions formed Ni–Co bimetallic hydroxide [NiCo2(OH)6] which were finally converted into NiCo2O4 nanoneedles after crystal growth and calcinations. However, according to some reports, in the presence of urea, metal carbonate hydroxides are initially formed instead of bimetallic hydroxides (Eqs. 4–7) [113].
Even ethanol as the solvent can also initiate the formation of metal carbonate hydroxides. Two-dimensional porous NiCo2O4 nanodisks were synthesized by a low-temperature hydrothermal method by Jain et al. [114] (Eqs. 8, 9). Figure 4 proposes the initial formation of Ni2(CO3)(OH)2 and Co2(CO3)(OH)2. Subsequent hydrothermal treatment in basic medium followed by calcination at 500 oC formed two-dimensional porous NiCo2O4 nanodisks.

Reproduced with permission from Ref. [114], Copyright © 2018 Elsevier B.V.
Schematic diagram for the synthesis of two-dimensional porous nanodisks of NiCo2O4.
The nature of alkali source, capping agent, and other additives significantly affects the morphology of the NiCo2O4 nanostructures. Wang et al. [99] reported tremella-like NiCo2O4 nanostructures in the presence of HMTA, which transformed into flower-like nanostructures when NH4F was also added along with HMTA. However, when HMTA was replaced with urea, urchin-like and pine needle-like NiCo2O4 nanostructures were formed, respectively, in the absence and presence of NH4F additive [99]. HMTA is hydrolyzed to produce NH3 which finally produces OH− ions as stated earlier in this section (Eq. 10).
It was suggested that the F− ions released from NH4F stimulate the initially formed nanosheets and nanoneedles to produce more active sites to further activate nucleation, more mass loading of active material per unit area, firm binding between the active material, and hence more crystal growth [115,116,117]. The possible set of reactions elaborating the role of F− ions released from NH4F is shown as follows [118] (Eqs. 11–13).
Further, different concentrations of the NH4F also stimulated the initially formed nanostructures to acquire more versatile morphologies. For 3, 9, and 12 mmol concentrations of NH4F, various morphologies of the NiCo2O4 nanostructures are shown in Fig. 5. With an increase in concentration from 3 to 9 mmol, aggregation of the neighboring nanosheets occured. Further increase in concentration to 12 mmol, rhombus-shaped architectures were formed [117].

Reproduced with permission from Ref. [117]. Copyright © 2014 Elsevier Ltd.
FESEM images representing the effect of concentration of NH4F on the morphologies of NiCo2O4 nanostructures: a–c 3 mmol NH4F; d–f 9 mmol NH4F; g–i 12 mmol NH4F.
Deng et al. [119] prepared novel urchin-like peapoded NiCo2O4@C nanostructures as a bifunctional catalyst for the water-splitting reaction. A three-phase process was proposed which included the initial hydrothermal synthesis of nanoneedles self-assembled microsphere followed by coating with polymerized glucose as green carbon source onto NiCo2O4 microsphere. The final stage was the calcination of the coated NiCo2O4 microsphere under N2 atmosphere to give urchin-like peapoded NiCo2O4@C. The fabrication process of urchin-like peapoded NiCo2O4@C is pictorially demonstrated in Fig. 6.

Reproduced with permission from Ref. [119], Copyright © 2017 Elsevier B.V.
Schematic diagram of the process of urchin-like peapoded NiCo2O4@C.
Still another way of engineering the morphology, porosity, and growth of the crystals along the particular oriented crystal planes of the nanomaterials, is the use of non-aqueous solvents. The modified method is named as solvothermal instead of hydrothermal. Solvents with different solubilities and polarities can significantly affect the degree of supersaturation, the diffusion rates of the chemical species to the surface of the growing crystals, the interfacial surface energy, etc. [120, 121]. Fu et al. [122] synthesized 1D porous NiCo2O4 microrods (using metal acetate salts) (Fig. 7a) and microspheres (using metal nitrate salts) (Fig. 7b) in aqueous and isopropanol media, respectively, under similar conditions of temperature and reaction time. In 1:1 ethanol:water medium, spindle-like hierarchical architectures composed of closely packed microplates aligned along one direction with sizes of 3–5 μm were formed (Fig. 7c). In pure ethanol microspheres composed of nanosheets, interweave together with an average diameter of 8 μm were formed (Fig. 7d). However, in diethylene glycol, irregular aggregates with sheet-like structures were synthesized (Fig. 7e).

Reproduced with permission from Ref. [122]. Copyright © 2017 American Chemical Society
FESEM images of NiCo2O4 architectures prepared solvothermally using different solvents a water, b isopropanol, c 1:1 ethanol: water, d pure ethanol, and e diethylene glycol.
Wang et al. [123] in an interesting stepwise hydrothermal growth synthesized layers of NiCo2O4 nanosheets on the surface of NiCo2O4 nanocones precursor to give highly ordered 3D hierarchical NiCo2O4@NiCo2O4 core–shell nanocone arrays on nickel foams (Fig. 8). Different morphologies were engineered by controlling the reaction time and the temperature during stepwise hydrothermal growth. Further, NiCo2O4 nanocones arrays on Ni foam were synthesized in the absence of HMTA while the NiCo2O4 nanosheets growth on NiCo2O4 nanocones was guided by the presence of HMTA.

Reproduced with permission from Ref. [123], Copyright © 2018 Elsevier B.V.
Schematic illustration for the formation of highly ordered 3D hierarchical NiCo2O4@NiCo2O4 core–shell nanocones arrays on nickel foams.
3.2 Templated Solution Growth Method
The morphology, size, shape, and surface area of nanostructures can be designed through template-based synthesis to produce nanostructures with controlled physical, chemical, electrical, and electronic properties essential in notable applications and are also quite different from those of the bulk materials. Generally, three stages, viz., template preparation, directed synthesis of the desired material using the template, and the template removal, are described in the overall growth process of nanostructures [124]. The chemical nature, structure, concentration, and growth temperature are some of the important environmental factors affecting the growth of nanomaterials. Template-based methodologies are reported in the literature which govern the synthesis of NiCo2O4 nanomaterials with versatile morphologies including nanospheres, hollow spheres, nanocages, hollow submicron spheres, hollow irregular octahedra-like cages, flower-like nanostructure, microspheres with highly ordered mesoporous structures, nanowires, etc. With the development of new methods for synthesizing mesoporous binary NiCo2O4 metal oxides, the combination of template method with other methods such as hydrothermal/solvothermal, sol–gel has been widely used. In one such study, Ren et al. [125] prepared mesoporous NiCo2O4 microspheres using a mesoporous silica (KIT-6) template. The KIT-6 template was added into the metal nitrate precursor solution prepared in ethanol. The schematic illustration of the formation of mesoporous NiCo2O4 microspheres is shown in Fig. 9a. The high porosity of the synthesized mesospheres was ascertained by FESEM and TEM images (Fig. 9b, c). The template was finally removed by etching with 2 M NaOH solution [125].

Reproduced with permission from Ref. [125]. Copyright © Authors
a Schematic illustration of the formation of mesoporous NiCo2O4 microspheres, b high-magnification FESEM image, and c TEM image of the mesoporous NiCo2O4 microspheres.
Yuan et al. [126] utilized silica spheres as hard templates prepared by the modified Stöber method [127], for the synthesis of hierarchical mesoporous hollow NiCo2O4 submicron spheres with uniform size and mesoporous textual property. These submicron spheres were composed of ultrathin nanosheets with a thickness of a few nanometers. The NaOH solution was used for the in situ removal of silica spheres. Dopamine—a biomolecule containing amine functional groups is capable of self-polymerize under alkaline conditions. It forms a layer of the polydopamine which attracts various metal ions including Co2+ and Ni2+ cations due to strong electrostatic interactions. Further, the alkalinity of the medium results in the formation of –OH–Ni–OH–Co–OH– complex networks. This property has been explored for the synthesis of NiCo2O4 nanostructures by Veeramani et al. [128]. FESEM images shown in Fig. 10 are demonstrating the effect of dopamine on the morphology of the NiCo2O4 nanostructures. Flower-like dopamine derived NiCo2O4 nanostructures were formed.

Reproduced with permission from Ref. [128], Copyright © 2016 American Chemical Society
Schematic illustration of the synthesis of dopamine-free and dopamine-NiCo2O4 nanostructures.
In another significant strategy, Xiong et al. [129] used mollusk shell-based macroporous carbon material (MSBPC), as a template to grow NiCo2O4 nanowires hydrothermally (Fig. 11a, b). The MSBPC was obtained from mollusc shells by removing calcium carbonate crystal and other biomacromolecules by acid treatment and carbonization. It was observed that there was uniform and dense growth of the NiCo2O4 nanowires on the inner walls of MSBPC channels. The average length of the NiCo2O4 nanowires was about 1.5 µm. Li et al. [130] reported the synthesis of composite C@NiCo2O4 hollow microspheres via a two-step strategy of hard template-induced hydrothermal synthesis followed by calcination. SiO2@RF (resorcinol–formaldehyde resin, RF) sphere was used as a hard template, whereas HMTA was used as precipitant. The template SiO2@RF was synthesized via a one-pot sol–gel process under alkaline condition using an alcohol–water mixed solvent [131]. The SiO2 core was removed by treating the prepared material with 2 M NaOH at room temperature for 12 h. The SiO2@RF template was having a core–shell structure with an average diameter of 350 nm (Fig. 11c). The NiCo2O4 nanoflakes were grown and assembled on the carbon surface of the SiO2@RF spheres (Fig. 11d). Recently, novel micron-sized NiCo2O4 pompon was prepared by templated growth using polyvinylpyrrolidone (PVP) non-ionic polymer and cationic surfactant CTAB as co-template [132]. Columbic and coordinative forces between template, co-template, and the metal ions help to form a stable “hairball” structure which finally was converted into a micron-sized pompon-like product on annealing (Fig. 11e). In contrast, in the absence of co-template CTAB, mesoporous NiCo2O4 hollow submicron spheres with a uniform diameter of 400–500 nm were obtained through a soft template method assisted by PVP (Fig. 11f). Further, in the absence of even PVP, solid submicron spheres were obtained [133].
FESEM images of a MSBPC, b NiCo2O4 nanowires grown on MSBPC. Reproduced with permission from Ref. [129]. Copyright © 2014 American Chemical Society. c TEM image of SiO2@RF spheres, d TEM image of NiCo2O4 nanoflakes grown on SiO2@RF spheres. Reproduced with permission from Ref. [130]. Copyright © 2018 Elsevier B.V. e FESEM image of micron-sized NiCo2O4 pompon. Reproduced with permission from Ref. [132]. Copyright © 2019 Elsevier B.V. f FESEM image of NiCo2O4 hollow submicron spheres. Reproduced with permission from Ref. [133]. Copyright © 2015 Elsevier B.V.
Qi et al. [131] also used RF microspheres as templates for the synthesis of NiCo2O4 hollow microspheres with tunable shell numbers and shell thickness. The shell numbers were controlled by adjusting the solvent ratio (DI water: ethylene glycol) and heating ramp rate, whereas the shell thickness and porosity were controlled by adjusting the metal ion concentrations (Fig. 12). For total molar concentrations of Ni2+ and Co2+ of 0.05 and 0.1 M, thin and thick shells, respectively, were formed. NiCo2O4 hollow microspheres with double and triple shells were formed at a heating ramp rate of 2 and 5 °C min−1, respectively, in EG as a solvent. With the increase in the ramp rate, the increased temperature gradient of the infused RF microspheres along the radial direction favors the separation of adjacent NiCo2O4 layers and the infused RF cores, thereby transforming double shell to triple shells [134]. Furthermore, EG prevents the formation of the metal aqua ions, and thus, the penetration of the metal ions into RF microspheres is accelerated which is essential for the formation of multi-shell NiCo2O4 hollow microspheres [135, 136]. Additionally, the final calcination process also results in some adhesion force in the outward direction and the contraction force by decomposition of the inner core which segregates the outer NiCo2O4 shell and the inner infused RF [131].

Reproduced with permission from Ref. [131]. Copyright © 2016 Elsevier B.V.
Pictorial representation for the formation of NiCo2O4 hollow microspheres with tunable numbers and shell thickness.
In addition to templates of organic origin, inorganic metal oxides have also been reported as template materials for the synthesis of NiCo2O4 nano-/microarchitectures [137]. Lv et al. [138] synthesized hollow NiCo2O4 octahedral nanocages via a Cu2O-templated strategy in combination with a coordination reaction. Uniform Cu2O octahedral crystals were prepared by reducing a copper-citrate complex solution with ascorbic acid in the presence of PVP. Initially, amorphous (NiCox)O(OH) was deposited onto the Cu2O octahedral crystals through a precipitation method. Cu2O octahedral crystals were etched according to a “coordinating etching and precipitating” (CEP) using Na2S2O3 as coordinating etchant [139] (Eqs. 14, 15). After that, the product was annealed at 400 °C for 2 h to get the hollow NiCo2O4 nanocages. In a similar study, Huang et al. [140] reported the synthesis of highly porous NiCo2O4 hollow nanospheres through a polycrystalline Cu2O-templated route based on “coordinating etching and precipitating” process. The excellent electron transfer capability, large specific surface area, and intrinsic redox couples of Ni2+/Ni3+ and Co2+/Co3+ ions, and superior electrocatalytic activity of NiCo2O4 hollow nanospheres were explored for glucose sensing by cyclic voltammetry and electrochemical impedance spectroscopy. NiCo2O4 hollow nanosphere-modified glassy carbon electrode (GCE) exhibited a high sensitivity of 1917 μA mM−1 cm−2, linear dynamic ranges of 0.01–0.30 mM and 0.30–2.24 mM, and very low detection limit of 0.6 μM (S/N = 3). Solid CuO octahedral is also reported as template materials for the synthesis of hollow octahedra-like NiCo2O4 cages. However, CuO templates can be simply removed by dissolving with a diluted NH4OH solution [141].
Yang et al. [142] reported NiCo2O4 hollow nanorods prepared by the sacrificial template-accelerated acid hydrolysis of ZnO (Eq. 16).
3.3 Sol–gel Method
The sol–gel process represents the chemical conversion of the liquid “sol” to the network “gel” phase, subsequently post-treatment into solid metal oxides with microcrystalline ultrafine particles. It is superior to other methods because it can better control the texture and surface properties of synthesized nanomaterials. The sol–gel method for the synthesis of nanomaterials is affected by numerous factors including pH, temperature, nature of solvent, growth time, agitations time, presence of capping agents, template, etc. With the consideration of these factors and potential applications, many protocols have been used to design materials of different sizes and features, including nano-, micro-, meso-, and macro-materials. To get excellent porosity and conductivity for potential electrochemical applications, the addition of polymers stuffs such as PVP [143], organic solvents/additives like propionic acid [144], citric acid [145, 146], N,N-dimethylformamide (DMF) [147], and epoxides like propylene oxide [148, 149], during the post-annealing process is suggested. Significantly the additive/metal ion molar ratio is very important in controlling the pore size and pore volume. Traditional use of SiO2 is avoided as its addition decreases the conductivity and limits the connection of the film with conducting substrate in thin film forms of NiCo2O4 [143]. In a typical sol–gel method, the NiCo2O4 spinel oxide was prepared by mixing appropriate amounts of metal salt precursors along with citric acid. The resulting solution was magnetically stirred at 80 °C for 2 h to get a gelatinous matrix. Finally, the matrix was calcined at 550 °C for 5 h to get the desired product [146]. Citric acid was also used as a chelating ligand for the synthesis of highly porous coral-like crystalline NiCo2O4 nanoparticles with submicron sizes via a facile sol–gel method in H2O-DMF mixture as solvent [147]. Liu et al. prepared nanoporous NiCo2O4 thin films deposited on ITO glass. The precursor solutions for NiCo2O4 nanospheres were prepared via a sol–gel method in glacial acetic acid and ethanol as solvents, and ethylene glycol and CTAB were used as a viscosity modifier template, respectively [150]. Thus, the sol–gel process is a proven and important method for preparing NiCo2O4 nanoparticles.
3.4 Co-precipitation Method
Better stoichiometric control and high purity of the metal oxide nanomaterials can be easily achieved through the coprecipitation method which involves simultaneous precipitation from a homogeneous solution of two or more cations. Simultaneous occurrence of nucleation, growth, coarsening, Ostwald ripening, and aggregation dramatically affect the size, morphology, and properties of the metal oxide nanoparticles. The technique has been applied for the synthesis of NiCo2O4 nanomaterials. NiCo2O4 hexagonal nanostructures were prepared by Bhagwan et al. [151] using Ni and Co chlorides and 6 M KOH as the precipitating agent. The schematic illustration for the formation of NiCo2O4 hexagonal is shown in Fig. 13a. It was suggested that the strong alkaline environment in the growth solution caused nickel and cobalt ions to precipitate and nucleate together, forming nickel–cobalt hydroxide which was subsequently converted into NiCo2O4 hexagonal after calcination at 300 °C. Liang et al. [152] reported hierarchical NiCo2O4 nanosheets@halloysite nanotubes (Fig. 13b). The initial formation of NiCo precursor@halloysite nanotubes was assisted by HMTA and dehydrated citric acid trisodium salt.
a Schematic representation for the synthesis of hexagonal NiCo2O4 nanosheets, Reproduced with permission from Ref. [151]. Copyright © 2019 Elsevier Ltd. and b hierarchical NiCo2O4 nanosheets@halloysite nanotubes via co-precipitation method. Reproduced with permission from Ref. [152]. Copyright © 2014 American Chemical Society
A stepwise co-precipitation template free method was designed by Chen et al. [153] for the synthesis of hierarchical urchin-like NiCo2O4 hollow nanospheres. Urea-assisted mesoporous urchin-like NiCo2O4 nanostructures were prepared by Jadhav et al. [154] by an easy, viable, and cost-effective co-precipitation method. Yu et al. [155] explored the structure-stabilizing properties of PVP, which can bind the metal ions through electrostatic interaction with the –N and/or C=O functional groups, for the formation of Ni–Co precursor particles with tetragonal prism-like shapes by a modified coprecipitation method. The yolk–shell Ni–Co oxide nanoprisms with a highly porous interior core structure consisting of numerous polycrystalline primary particles were obtained finally after annealing. Other stabilizing and precipitating agents like ethylene glycol (EG) [156], urea [157], NaOH, NH4OH, NH4HCO3, H2C2O4 [158, 159] and NaHCO3 [153] are reported in the literature. Organic stabilizers such as EG are supposed to form a protective layer around the particle surface through interactions with hydroxyl groups preventing the aggregation. Moreover, EG also acts as a bidentate chelating ligand for solvated metal ions [160]. Another important factor that controls the morphology, shape, and size of the nanoparticles is the pH of the reaction medium during coprecipitation. Wan et al. [159] observed the change in morphology of the NiCo2O4 precursors from the cubic to the fibrous along the axial direction. The fibrous morphology was maintained at a still higher pH value of 8.4; however, the aspect ratio was increased (Fig. 14a–d). A dynamic equilibrium was suggested to exist between metal ammoniated complexes and the coprecipitation of Ni2+ and Co2+ as their oxalates.

Reproduced with permission from Ref. [159]. Copyright © 2018 Elsevier Ltd.
FESEM images NiCo2O4 precursor powders prepared at a pH = 3, b pH = 7, c pH = 8 and d pH = 8.4.
The post-annealing temperature is also an important factor for controlling the morphology of the NiCo2O4 spinel structures. The homogeneous dark blue-colored suspension which was obtained by mixing the metal nitrates and NaOH solution was initially evaporated under rotation and reduced pressure conditions by a cost-effective rotary evaporation method. Hexagonal column-like mesoporous loose architectures and hexagonal dense blocks were obtained at 200 and 400 °C calcination temperatures, respectively (Fig. 15) [161].

Reproduced with permission from Ref. [161]. Copyright © 2018 Elsevier Ltd and Techna Group S.r.l.
Schematic illustration of the synthesis of hexagonal mesoporous structured NiCo2O4 (HMS-NCO) and NiCo2O4 calcined at 400 °C (NCO-400).
3.5 Electro-Deposition
Electro-deposition is considered a very useful, versatile, and flexible tool for the deposition of dendritic hierarchical structures, thin and thick films, nanosheet, nanofoil, nanotubes, nanowires, and many well-ordered transition metal oxides on conducting surfaces. Potentiostatic, galvanostatic, and pulse plating are the three main techniques employed for electro-deposition [162, 163]. The basic principle of electro-deposition involves three steps, viz. preparation of a metal ions precursor solution, co-electro-deposition, and final thermal decomposition [164]. Recently, this technique has also been used for the preparation of NiCo2O4 spinel structures for various applications, including supercapacitors, anode materials for Li-ion batteries, gas sensors, biosensors, etc. Wu et al. [165] deposited nanostructured cauliflower-like NiCo2O4 film through galvanostatic electro-deposition combined with annealing treatment (Fig. 16). Galvanostatic electro-deposition was performed using a three-electrode compartment comprising a stainless steel disk as a working electrode. An Ag/AgCl saturated with KCl and a platinum plate were used as the reference and counter electrodes, respectively. Hydroxide-SiO2 template transformed nanoflakes to cauliflower-like NiCo2O4 nanoparticles. Under cathodic potential, the generated OH− ions catalyzed the sol–gel process for the formation of SiO2. The generated OH− ions facilitated the formation of Ni(OH)2 and Co(OH)2. Heat treatment of the deposited at 250 °C in air for 2 h converts the metal hydroxides into NiCo2O4 films.

Reproduced with permission from Ref. [165]. Copyright © 2017 Hydrogen Energy Publications LLC. Published by Elsevier Ltd
Schematic illustration of the formation of cauliflower-like NiCo2O4 film.
Wang et al. [166] reported the electro-deposition of the nickel/cobalt/zinc ternary alloy layer on ultrafine nickel wire. Removal of the zinc by dealloying with NaOH solution followed by oxidation at the atmospheric environment resulted in mesoporous NiCo2O4 film on the surface of ultrafine nickel wire. Zhao et al. [167] grew NiCo2O4 nanosheet networks on carbon cloth through a simple cathodic electro-deposition process followed by post-annealing at 300 °C in an air atmosphere for 120 min. The average mass loadings for NiCo2O4 nanosheet networks grown on carbon cloth at different electro-deposition times 200, 400, and 600 s were 0.4, 0.6, and 0.9 mg cm−2, respectively. The \({\text{NO}}_{ 3}^{ - }\) ions from the metal salts were reduced to \({\text{NO}}_{ 2}^{ - }\) and \({\text{NH}}_{ 4}^{ + }\) ions at the cathode. This reduction also resulted in the formation of OH− ions which combined with the Ni2+ and Co2+ to form amorphous binary metal hydroxide NiCo2(OH)6 nanosheet networks [168]. Post-annealing transforms the NiCo2(OH)6 into NiCo2O4 nanosheet networks [57, 169] (Eqs. 17–20).
The dissolution of the ions decreases near the electrode due to the formation of the OH− ions and an increase in pH near the electrode is observed. Since the solubility constants of Ni(OH)2 (8.2 × 10−16) and Co(OH)2/3 (2.5 × 10−16) are very low and comparable, their simultaneous precipitations occur which finally gives NiCO2(OH)6 [170, 171]. Ramadoss et al. [169] electrodeposited highly porous and binder-free 3D flower-like NiCo2O4/Ni nanostructures on Ni wire and explored their supercapacitor applications (Fig. 17a). The high porosity of the nanostructures was attributed to the presence of H2 bubbles produced by hydrogen evolution reaction during electro-deposition. Furthermore, H2 bubbles also acted as a template for the construction of a 3D flower-like NiCo2O4/Ni with dendritic walls on the Ni wire. Nanoforest hierarchical composites Co3O4@NiCo2O4 nanowire arrays were synthesized by Zhang et al. [172]. Co3O4 nanowires were initially grown on Ni foam through a facile hydrothermal method. After that, NiCo2O4 was electrochemically deposited in the Co3O4 nanowires to avoid the conventional aggregation (Fig. 17b). Mirzaee et al. [173] proposed a two-step method involving initial electro-deposition followed by thermal treatment at 300 °C with a ramping rate of 1 °C min−1 to form flower-like arrays of NiCo2O4 on electrochemically reduced graphene oxide (ERGO) which itself was deposited on nickel–nickel oxide foam.
a Electrodeposited 3D flower-like NiCo2O4/Ni nanostructures on Ni-wire. Reproduced with permission from Ref. [169], Copyright © 2016 The Royal Society of Chemistry. b Schematic representation of the formation of nanoforest hierarchical composites Co3O4@NiCo2O4 nanowire arrays. Reproduced with permission from Ref. [172]. Copyright © 2013 Elsevier Ltd.
In addition to these, NiCo2O4 architectures of versatile morphologies have been electrochemically deposited on a variety of conducting surfaces. Some of these include honeycomb-shaped NiCo2O4 on carbon cloth [174], ultrathin NiCo2O4 nanosheets on three-dimensional interwoven nitrogen-doped carbon nanotubes [175], ultrathin porous NiCo2O4 nanosheet arrays on flexible carbon fabric, 3D vertically aligned carbon nanotubes/NiCo2O4 core/shell structures [176], hybrid composite Ni(OH)2@NiCo2O4 on carbon fiber paper [177], 3D hierarchical NiCo2O4@MnO2 hybrid nanomaterial on stainless steel mesh [178], freestanding bowl-like NiCo2O4 on carbon fiber paper [179], network-like holey NiCo2O4 nanosheet arrays on Ni foam [180], NiCo2O4@ carbon nanofibers [181], and many more.
3.6 Combustion Method
Combustion synthesis, also referred to as self-propagating high-temperature synthesis is one of the most versatile, convinced, convenient, cost-effective, and fast method for the synthesis of nanomaterials. It involves a thermally induced redox reaction between precursor salt as oxidizers and an organic fuel [182,183,184]. Glucose, fructose, tartaric acid, sucrose, glycine, citric acid, hydrazine, urea, and oxalic acid are generally used as organic fuels. However, if metal oxalate or acetate salts are used, the combustion process can be directly conducted in the absence of fuel [185]. Byproduct gases like CO2, H2O, N2, oxides of N (NOx) and S (SOx), etc. are evolved during the combustion process [186]. The release of these gases promotes the expansion of the product and rapid fall in temperature after the reaction ceases. This provides a solid product with a high degree of porosity and good dispersibility [187]. As compared to solid-state combustion, liquid phase combustion synthesis has proved to be the most suitable one as oxidizers and fuel are well dissolved in aqueous or alcoholic solutions [188]. Ni(NO3)2·6H2O, Co(NO3)2·6H2O (in 1:2 molar ratio) as oxidizers and tartaric acid as fuel were dissolved in acidified 2-methoxy ethanol solution. The resulting solution was combusted at 250 °C for 1 h to prepare NiCo2O4 nanoparticles [189]. Sucrose assisted combustion of the Ni and Co nitrates also resulted in NiCo2O4 nanoparticles when the combustion process was carried out at 350 °C for 6 h [190]. The oxalate precursors were directly decomposed into NiCo2O4 powders by heating in an air ambient atmosphere at 320 °C for 10 h [185]. Citric acid assisted combustion at 400 °C for 4 h resulted in highly porous NiCo2O4 nanomaterials [191]. Urea-assisted combustion was processed at 400 °C for 2 h in ethyl acetate as a solvent [192]. In each case, a viscous gel is obtained initially by heating the reaction solution at low temperature followed by auto-ignition resulting in the formation of highly fluffy mass which is finally calcined at high temperature. Direct calcination of the metal nitrate salts in the presence of alkalis without any fuel has also been reported for the synthesis of the NiCo2O4 nanorods [41].
Though it is a fast and low-cost method for the synthesis of NiCo2O4 powders, it suffers from some major drawbacks including less control over morphological uniformity and particle size, the simultaneous formation of a variety of crystal phases, the formation of highly agglomerated structures, complex and uncertain growth mechanism, and critically very low possibilities of formation of a versatile and wide range of morphological structures as those of in hydrothermal and other solution methods.
3.7 Electro-Spinning Method
Many electrospun carbonaceous materials such as carbon nanofibers, single-walled carbon nanotubes, multi-walled carbon nanotubes, etc. prepared from oxidation and carbonization of polymers like PVP, PAN, PVA have been used as templates for the growth and deposition of NiCo2O4 nanostructures with versatile morphologies. In one synthetic way, there is simultaneous growth of NiCo2O4 nanostructures and electro-spinning of template material [193, 194]. In another strategy, NiCo2O4 nanostructures are grown through other synthetic methods like hydrothermal, sol–gel, coprecipitation, etc. on pre-electrospun carbonaceous templates [39]. Electro-spinning setup comprises a high-voltage system, spinneret, and collector which results in the formation of continuous nanofibers with diameters ranging from nanometer to micrometer [195,196,197]. The deposition of NiCo2O4 nanostructures on these carbonaceous materials not only improves the electrical and electronic properties but also enhances the thermal, mechanical and chemical stabilities which are the important prerequisite characteristics for the biosensing and other applications. The composition of the precursor solution, presence of additives like templates and capping agents, modification in the electro-spinning setup, post-annealing, electrospun voltage are some of the major factors which control the thickness, porosity, and morphology of the deposited NiCo2O4 films. Lai et al. [198] through electro-spinning, co-deposition, redox deposition fabricated NiCo2O4-doped carbon nanofiber@MnO2 nanosheet and nanorod hybrid membranes. Busacca et al. [199] prepared NiCo2O4/carbon nanofibers composites and investigated their oxygen evolution reaction in alkaline electrolyte. Metal acetate salt precursor in a molar ratio 1:2 was mixed in PAN (as carbon source) and DMF. The electrospun layer was thermally oxidized at 270 °C in air for 30 min followed by subsequent carbonization at 900 °C for 1 h under a helium gas flow. Li et al. [193] fabricated porous one-dimensional NiCo2O4 nanostructures via a single-spinneret electro-spinning method. Stoichiometric amounts of Ni and Co nitrates were homogeneously mixed in a solution prepared by dissolving PVP in ethanol and N,N-dimethylformamide. Metallic precursor concentration: PVP (M: PVP) ratio was significant in determining the morphologies of the electrospun one-dimensional NiCo2O4 nanostructures. For 0.44:1, 0.61:1, and 0.87:1 M: PVP ratios, NiCo2O4 nanofibers, nanotubes, and nanobelts were formed. The versatility in morphologies was attributed to the fast water evaporation and burning off of PVP during annealing. Guan et al. [194] synthesized spinel NiCo2O4 nanofibers with diameters of 50–100 nm through electro-spinning of the PVA/cobalt acetate/nickel acetate composite precursor followed by annealing at high temperatures ranging from 400 to 800 °C. Liu et al. [39] demonstrated the surfactant-assisted hydrothermal uniform growth NiCo2O4 nanoneedle on electrospun carbon nanofiber (ECF) and explored their glucose sensing properties non-enzymatically. ECF film was prepared through initial electro-spinning and subsequent oxidation and carbonization of PAN (Fig. 18a–c). Xu et al. [200] instead of PAN used PVP as a carbon source to produce NiCo2O4 nanotubes. These nanotubes were used as scaffolds for hydrothermal growth of MnO2 nanosheets for the additional improvement in electronic conductivity and electrochemical activity for supercapacitor applications (Fig. 18d–f). Copolymers like poly (acrylonitrile-co-methylhydrogen itaconate) [201] and biobased polymer composites such as PAN/lignin [202] are also reported in the literature for the formation of flexible carbon nanofibers. The hollow carbon nanofibers were used as a template for the hydrothermal growth of NiCo2O4 with uniform dandelion-like morphology consisting of densely grown nanoneedle (Fig. 18g, h) [203]. The above discussion thus reveals that the proper combination and the composition of the polymers can result in the formation of carbonaceous materials with versatile structural features with high surface area necessary for potential applications.
a Fabrication procedure of NiCo2O4/ECF nanohybrids, b FESEM image of ECF and c FESEM image of NiCo2O4 nanoneedles grown on ECF. Reproduced with permission from Ref. [39]. Copyright © 2017 Elsevier B.V. d The fabrication procedure for NiCo2O4@MnO2 composites, e FESEM image of NiCo2O4 nanotubes and f FESEM image of MnO2 nanosheets grown of NiCo2O4 nanotubes. Reproduced with permission from Ref. [200]. Copyright © 2016 Elsevier B.V. g, h Low- and high-magnification FESEM images, respectively, of NiCo2O4 with uniform dandelion-like morphologies. Reproduced with permission from Ref. [203]. Copyright © 2019 Elsevier Ltd.
3.8 Microwave-Assisted Method
Microwaves are the electromagnetic radiations having a frequency range between 300 MHz and 300 GHz and a wavelength range of 1 m–1 mm. Microwave-assisted synthesis of nano-/microstructures is superior to the conventional methods described above because it requires a very short reaction duration, is energy efficiency, cost-effectiveness, and gives an excellent yield of highly porous materials. Microwaves result in volumetric heating as they can penetrate throughout the volume of reactants [204]. This volumetric heating is caused by various types of polarization in the medium, including electron polarization, atomic polarization, directional polarization, and space charge polarization [205]. To obtain better morphological results, microwave-assisted synthesis of nanomaterials is usually combined with other synthetic methods such as sol–gel, co-precipitation, and hydro/solvothermal, etc. Recently, the improvement in the hydrothermal method in harmony with microwave assistance has been studied to synthesize NiCo2O4 nano-/microstructures. Other ways of engineering the structural aspects of the NiCo2O4 are the use of a template, capping agents, organic solvents, ionic solvents, and addition of other growth additives. The microwave-assisted hydrothermal method was applied by Zhang et al. [206] to prepare NiCo2O4 double-shelled hollow spheres with an outer and inner shell thickness of ~ 20 and ~ 70 nm, respectively. A mixture of isopropanol and glycerol was used to prepare a reaction solution (Fig. 19a). Glycerol molecules were supposed to form a self-assembled quasi-emulsions in isopropanol that serve as a soft template for the growth of Ni–Co double hydroxides. In the absence of glycerol, solid microspheres with diameters of ~ 1 µm were formed, demonstrating the templated role of glycerol in the synthesis of a double-shelled hollow nanostructure (Fig. 19b–d). In the presence of microwaves, the reaction mixture is heated due to dielectric loss, which significantly accelerates the reaction kinetics. Additionally, the presence of microwaves improves uniformity in terms of dispersion and size distributions.

Reproduced with permission from Ref. [206]. Copyright © 2017 Springer Nature
a Pictorial representation of the microwave-assisted hydrothermal synthesis of NiCo2O4 double-shelled hollow spheres. b FESEM and c TEM images of NiCo2O4 hollow spheres prepared in the presence of glycerol and d FESEM image of the NiCo2O4 structures prepared in the absence of glycerol.
Shanmugavani et al. [207] analyzed the effect of reaction times on the morphology of the NiCo2O4/NiO nanocomposites. The reaction was carried out in the presence of oxalic acid at an operating frequency of 2.45 GHz and 800 W output power. It was proposed that the initially formed nanoparticles are converted into bundled-like structures as the reaction time was increased. Recently, Sun et al. [103] reported novel porous nanoscale NiO/NiCo2O4 heterostructure through two-stage calcination of nickel–cobalt bimetallic hydroxide precursors (NiCo precursors) which were initially synthesized using a microwave-assisted hydrothermal method in the presence of HMTA and NH4F. Notably, F− ions were supposed to act as functional template agents. Prolonged irradiation significantly affects the morphology of NiCo2O4 materials. When the irradiation time was increased from 5 to 40 min, the incompletely self-assembled and non-uniform 2D nanosheets are converted into more optimized and thickened 3D frameworks with large open spaces (Fig. 20a–i).

Reproduced with permission from Ref. [103]. Copyright © 2019 Elsevier Inc.
FESEM images of bimetal Ni-Co-precursors obtained under various microwave-assisted hydrothermal reaction times. a 5 min, b, c 10 min, d 15 min, e 20 min, f 25 min, g 30 min, h 35 min, and i 40 min.
Nakate et al. [208] prepared nanocrystalline NiCo2O4 nanoplates in the surfactant-free environment using metal chloride salts precursors through microwave irradiation. Gu et al. [209] reported 3D nanosphere-like NiCo2O4 nanostructure composed of intertwined 2D ultrathin mesoporous nanosheets having large specific surface area 146.5 m2 g−1. The reaction solution was exposed to microwaves (power 560 W) for 6 min. Su et al. [210] reported highly crystalline NiCo2O4 supported on carbon black via a simple, one step intermittent microwave heating method avoiding the calcination process. However, in a contrary study, Tao et al. [211] analyzed the effect of post-annealing temperature on the morphologies of the NiCo2O4. Ni–Co double hydroxide was initially prepared through a microwave-assisted method using a tertbutanol solution (98%). Flower-shaped morphology of the Ni–Co double hydroxide was completely converted into unique coral-like morphology on calcination. As the post-annealing temperature was increased from 400 to 700 °C, individual ultrathin nanosheets shrink to smaller nano-sized crystal grains which finally self-assembled to form coral-like NiCo2O4 architectures.
For greener perspectives, ionic solvents like [1-butyl-3-methylimidazolium][BF4] {[Bmim][BF4]}, [Bmim]FeCl4, [Bmim]Cl [212], and non-ionic glucose-based polymeric surfactant, β-C10Alkyl Poly Glucoside [213] are also reported in the literature for the synthesis of NiCo2O4 architectures with versatile morphologies.
3.9 Spray Pyrolysis Method
In spray pyrolysis technique, an aerosol of various precursor components is prepared in suitable solvent and is sprayed on the substrate. After that, sequential evaporation of the solvent from the surface of the substrate, heating to precipitate out the solute, high-temperature annealing, formation of microporous particles, and finally, sintering of solid particles is carried out [214]. NiCo2O4 nanostructures with morphologies hollow nanosphere [215], hollow microspheres [216], dried plum-like particles [217], yolk–shell microspheres [218], nanoaggregates [219], thin films with uniform particle distribution size 20–30 nm [220], etc. are reported (Fig. 21a–e).
Morphologies of various NiCo2O4 nanostructures a hollow nanosphere. Reproduced with permission from Ref. [215]. Copyright © 2017 The Korean Society of Industrial and Engineering Chemistry, Published by Elsevier B.V. b hollow microspheres. Reproduced with permission from Ref. [216]. Copyright © 2019 Elsevier Ltd and Techna Group S.r.l. c yolk–shell microspheres. Reproduced with permission from Ref. [218]. Copyright © 2017 Elsevier Ltd. d nanoaggregates. Reproduced with permission from Ref. [219]. Copyright © 2015 Elsevier Inc. and e thin films with uniform particle distribution size 20–30 nm. Reproduced with permission from Ref. [220]. Copyright © 2016 Elsevier Ltd.
Similar to the electro-spinning method, carbonaceous materials such as reduced graphene oxide, carbon nanotubes, carbon nanofibers are also mixed in the precursor solution to improve the electrochemical properties of NiCo2O4. Park et al. [221] synthesized three-dimensional macroporous multi-walled carbon nanotubes microspheres densely loaded with NiCo2O4 hollow nanospheres via spray pyrolysis process. The schematic illustration depicting the formation mechanism is shown in Fig. 22a. The polystyrene nanobeads added in the solution improved the structural uniformity and the dispersion of CNT microspheres. The similarity in the atomic radii of the Ni and Co ions resulted in the Kirkendall diffusion into the outer surface of the where they were oxidized to form NiCo2O4 (Fig. 22b).

Reproduced with permission from Ref. [221]. Copyright © 2017 Elsevier Ltd.
Formation mechanism of 3D macroporous multi-walled carbon nanotubes microspheres densely loaded with NiCo2O4 hollow nanospheres.
4 Biosensor Applications of Nano-/Micro-structured NiCo2O4
4.1 Glucose Biosensors
Non-enzymatic glucose sensing is considered to be a better, fast, and convenient way as compared to the enzymatic method since the later is a complicated and multi-step process involving immobilization of enzyme bioreceptor such as glucose oxidase, glucose dehydrogenase, and quinoprotein glucose dehydrogenase onto the electrode surface [51, 52]. Furthermore, maintaining the enzyme stability under non-physiological conditions of observations is another major issue related to enzymatic glucose biosensing. Most of the biosensing measurements are based on cyclic voltammetry (CV) and amperometric analysis. Better biosensing behavior and electrochemical activity using NiCo2O4 nano-/microstructure-modified electrodes are adjudged by broader redox peaks with larger area coverage in the CV curves. Since the spinel NiCo2O4 comprises binary intrinsic-state redox couples of Ni3+/Ni2+ (0.58 V/0.49 V) and Co3+/Co2+ (0.53 V/0.51 V), only a pair of redox peaks in the CV curves is generally observed due to almost similar redox potential values for NiO and Co3O4 [142, 222, 223]. In alkaline medium, NiCo2O4 is oxidized to Ni and Co perhydroxides which finally convert glucose into gluconolactone (Eqs. 21–26) [224].
Since the rates of oxidation of Ni2+ and Co2+ ions on the electrode surface during anodic scan determine the rate of sensing of glucose, NiCo2O4 nano-/microstructures with versatile morphologies having large specific surface area, permeability, and most importantly short electron and ion diffusion pathways are synthesized. Ni3+ and Co3+ ions are reduced back to Ni2+ and Co2+ ions by the electrons lost by the oxidation of glucose to gluconolactone. According to Hussain et al. [225], H2O2 is formed as one of the products along with gluconolactone if the electrochemical sensing is performed in the presence of oxygen. Glucose undergoes a spontaneous reaction with water and O2 to form gluconolactone which is further oxidized into gluconic acid (Eqs. 27, 28). In a slightly basic medium (pH = 7.4), gluconic acid ionizes to gluconate ions which act as mobile charge carriers on the surface of the NiCo2O4 nanostructures producing a strong electrical signal (Eq. 29). Elakkiya et al. [226] reported highly porous flower-like NiCo2O4 nanostructures synthesized via a facile hydrothermal method for excellent electrocatalytic activity in alkaline electrolyte for the oxidation of glucose and lactic acid.



The binary spinel NiCo2O4 architecture exhibits better intrinsic electronic conductivity as compared to pure NiO and Co3O4 which is attributed to the doping of Ni3+ ions in the octahedral sites of the Co3O4 crystal lattice which accelerates the electron hopping process [227]. Huang et al. [140] compared the electron transfer resistance (Ret) through electrochemical impedance spectroscopy for GCE modified with NiCo2O4, NiO, and Co3O4. Nyquist plots for all the modified GCE consisted of two portions; an inclined line at low frequencies and a semicircular portion at high frequencies. However, the lowest Ret of NiCo2O4/GCE was an indication of the enhanced conductivity for NiCo2O4 (Fig. 23a). Broader redox peaks NiCo2O4/GCE as compared to NiO/GCE and Co3O4/GCE confirmed the better biosensing behavior of the NiCo2O4 as compared to Co3O4 and NiO (Fig. 23b).

Reproduced with permission from Ref. [140]. Copyright © 2016 Elsevier B.V.
a Nyquist plots of NiCo2O4, Co3O4, and NiO-modified GCE in 0.1 M NaOH. b CV curves for NiCo2O4, Co3O4, and NiO-modified GCE in 0.2 M NaOH without glucose.
Spinel NiCo2O4 hollow nanocages were prepared by using Co-based zeolite imidazole frameworks (ZIF-67) as a template and precursor by Feng et al. [228]. Morphological characterization revealed that the thickness of the cage shell was about 30 nm. The outer surface of the nanocages was covered with small nanosheets. A wide linear dynamic range 0.18 μΜ–5.1 mM, high sensitivity 1306 μA mM−1 cm−2, a fast response time of 1 s, and limit of detection 27 nM were observed for NiCo2O4 hollow nanocage-based modified GCE.
NiCo2O4 nanoplates interconnected through MoS2 nanosheets performed excellent electrocatalytic behavior toward glucose. NiCo2O4 nanoplates and MoS2 nanosheets illustrated a significance synergic effect. Though not an active catalyst for the oxidation of glucose, the highly active edge of vein-like MoS2 nanosheets inhibited the agglomeration of NiCo2O4 nanoplates and formed long conducting chains which provide an alternative pathway with lower electrical resistance [229] (Fig. 24a, b). The fabricated glucose biosensor exhibited a high sensitivity of 1748.58 μA mM−1 cm−2 and a very low detection limit of 0.152 μM. MoS2 nanosheets have also been reported as support material for the fabrication of NiCo2O4/MoS2 nanocomposites through a simple ionothermal method in deep eutectic solvent (choline chloride (ChCl)-urea mixture) [230]. Deep eutectic solvents consist of simple eutectic-based ionic liquids prepared by eutectic mixing of ChCl and some hydrogen bond donors like acids, amides, alcohols, etc. [231]. These solvents have excellent thermal stability, high surface tensions, negligible vapor pressure, and most importantly biodegradability [232,233,234,235,236]. The NiCo2O4-MoS2/chitosan/GCE-modified electrode was used as an electrochemical sensor for glucose in red wine and honey [230].

Reproduced with permission from Ref. [229]. Copyright © 2017 Elsevier B.V.
a Electrocatalytic activities of MoS2-NiCo2O4/GCE in 0.1 M NaOH at a scan rate of 50 mV s−1. b Amperometric response curves for MoS2-NiCo2O4/GCE.
Analysis of non-enzymatic glucose sensing properties of NiCo2O4 nanosheets showed linear response with respect to the change in glucose concentration varying from 5 to 65 μM. The high sensitivity of 6.69 μA μM−1 cm−2 with a LOD value of 0.38 μM and liquid of quantification of 1.27 μM was observed. During CV measurements, scan rates increased the oxidation and reduction peak currents as well as peak-to-peak separations [224]. The electrochemical kinetics of the NiCo2O4 hollow nanorods grown on stainless steel via a sacrificial template showed similar trends during glucose sensing in 0.1 M NaOH solution with scan rates ranging from 5 to 100 mV s−1 (Fig. 25a). Amperometric studies revealed a steady-state current optimization within 2 s of glucose addition. Calculated sensitivity, linear detection range, and detection limit were 1685.1 µA mM−1 cm−2, 0.0003–1.0 mM, and 0.16 µM (S/N = 3), respectively (Fig. 25b) [142]. Cui et al. [237] prepared rectangular flake-like mesoporous NiCo2O4 via a facile hydrothermal method and observed glucose biosensing sensitivity of 662.31 µA mM−1 cm−2 and very low detection limit of 0.3 nM at S/N = 3. The other optimized operational parameters were: 0.2 M KOH, + 0.5 V applied potential and 1.0 mg mL−1 loading of meso-NiCo2O4 in the suspensions. Dry rod-like NiCo2O4 synthesized through a facile hydrothermal reaction followed by subsequently microwave treatment. The non-enzymatic glucose sensor fabricated using these rod-like features showed a high sensitivity of 431.29 µA mM−1 cm−2 [238]. The microwave treatment completely removed the water and made the material highly porous for exhibiting excellent biosensing applications. One-dimensional porous NiCo2O4 nanowires array grown on nickel foam (NiCo2O4 NWs/NF) via a facile hydrothermal method exhibit highly efficient glucose sensitivity of 5916 μA mM−1 cm−2, a detection limit of 1 μM–3.987 mM and LOD of 0.94 μM (S/N = 3) [239]. As conducting substrate, nickel foam not only provides the large electrochemically active surface area due to three-dimensional interconnected features, but also directs the growth of one-dimensional NiCo2O4 porous nanowires [240]. Besides, the one-dimensional porous NiCo2O4 nanowires array provided sufficient transport channels for ions and abundant active sites for redox reactions. Carbon cloth has also been used as a potential conducting surface for the growth of porous NiCo2O4 nanowires. As fabricated enzyme-free NiCo2O4 porous nanowire arrays supported on carbon cloth-based electrode for glucose sensing exhibited a linear dynamic range of 1 μM–0.63 mM, the sensitivity of 4.12 mA mM−1 cm−2, and low detection limit of 0.5 μM [241].

Reproduced with permission from Ref. [142]. Copyright © 2015 Elsevier B.V.
a CV curves of NiCo2O4 hollow nanorods with various scan rates. Inset: redox peak currents as a function of the scan rates, b variation in response current for NiO (curve a), Co3O4 (curve b), and NiCo2O4 hollow nanorods (curve c) as a function of glucose concentrations. Inset: calibration curve of NiCo2O4 hollow nanorods.
One of the main disadvantages of using bare NiCo2O4 is its poor electrical conductivity. However, this limitation can be overcome by forming its composite/hybrid materials. It has been reported that the electrical conductivity and hence the electrochemical biosensing performance of NiCo2O4 can be improved by making its composites with conducting carbonaceous materials like graphene, reduced graphene oxide, carbon nanotubes (single and multi-walled), carbon nanofibers; conducting polymers like polypyrrole (PPy), polyaniline (PANI); metal oxides NiO, Co3O4, SnO2, MnO2; and metals like Au, Pd, etc. Among these, the carbonaceous materials are considered to be potential candidates as compared to others due to their excellent electrical conductivities, good mechanical strength, thermal and chemical stabilities, and resistance to oxidation–reduction reactions. Besides, these carbonaceous materials provide a large specific surface area for better adsorption of analytes, which ultimately results in very high sensitivity and very low detection limits.
The two-dimensional one-atom-thick layered structure of graphene has been extensively used for making composites with NiCo2O4 due to its high specific surface area of 2670 m2 g−1 and excellent conductivity [242, 243]. Studies have revealed a higher specific surface area for the NiCo2O4/reduced graphene oxide composites as compared to bare NiCo2O4 nanoparticles (Fig. 26a) [244]. Even the pore width was less in the case of NiCo2O4/reduced graphene oxide composites. Various glucose-sensing scans are given in Fig. 26b–d. The enhanced redox peak current density for NiCo2O4/reduced graphene oxide composites as compared to pure NiCo2O4 was attributed to the lesser extent of aggregation of graphene sheets due to the interception of the NiCo2O4 nanoparticles on graphene surface causing weakening of π–π interaction between individual graphene sheets, faster diffusion rates and electron transfer between the glucose molecules and the electrode surface [245].

Reproduced with permission from Ref. [244]. Copyright © 2016 Elsevier B.V.
a Adsorption–desorption hysteresis loop, specific surface area (SSA), average pore width, and total pore volume of the synthesized pure NiCo2O4 and NiCo2O4/graphene nanohybrids. b CV for NiCo2O4/graphene hybrid-modified electrode. c Effect of scan rates scan rate for the solution with 0.1 mM glucose in 0.1 M NaOH. d Linear sweep voltammetric curves for glucose in the concentration of 0–0.14 mM and calibration plot (Inset).
Ma et al. [246] developed NiCo2O4 nanowrinkles/reduced graphene oxide hybrid-based modified GCE for non-enzymatic glucose detection at the physiological level. As far as the concentration of the glucose is concerned, the oxidation potential of glucose decreased while oxidation peak current increased proportionally to a greater extent for NiCo2O4 nanowrinkles/reduced graphene oxide hybrid-based modified GCE as compared to single component Co3O4, NiO and bare NiCo2O4 at a scan rate of 100 mV s−1 in 0.1 M NaOH (Fig. 27a–d). The results confirmed the crucial role of reduced graphene oxide in improving the electrocatalytic biosensing performance of the NiCo2O4 spinel for different concentrations of glucose.

Reproduced with permission from Ref. [246]. Copyright © 2016 Elsevier Ltd.
CV plots for different glucose concentrations (1–4 mM) in 0.1 M NaOH a Co3O4, b NiO, c NiCo2O4, and d NiCo2O4 nanowrinkles/reduced graphene oxide-modified GCE.
In addition to two-dimensional graphene, Wu et al. [245] reported the synthesis of three-dimensional graphene foam (3DGF) through a chemical vapor deposition technique. The 3DGF provides additional stability and large porous surface as well as high conductivity to the hierarchical NiCo2O4 composites. NiCo2O4 hierarchical nanoneedles were deposited onto the surface of 3DGF via a hydrothermal method. The synergism between hierarchical NiCo2O4 nanoneedles and 3DGF exhibited a high sensitivity of 2524 μA mM−1 cm−2 and a limit of detection 0.38 μM (S/N = 3). Further, as fabricated electrode showed excellent selectivity for glucose even in the presence of interfering compounds like dopamine, ascorbic acid, lactose, d-Fructose, and urea as negligible current responses were observed on their additions as compared to glucose. NiCo2O4 nanospheres/reduced graphene oxide composite prepared by a template-based method using the Cu2O/GO template achieved a high sensitivity of 2082.57 μA mM−1 cm−2, the detection range of 0.04–1.28 mM, and low detection limit of 0.7 μM [137]. Ni et al. [247] reported a reduced graphene oxide supported NiCo2O4 nanorods composite prepared via an ionothermal method using deep eutectic solvents. The modified GCE exhibited superior electrocatalytic biosensing of glucose with a wide double-linear range from 1 μM to 25 mM and a very low detection limit of 0.35 μM (S/N = 3). The presence of a large number of small interconnected nanoparticles on the surface of the NiCo2O4 nanorods provided the dense electrocatalytic active site in coordination with reduced graphene oxide which provided large surface area and excellent electrical conductivity (Fig. 28a).
a Proposed mechanism of glucose sensing using NiCo2O4 nanorods/rGO/Nafion composite-modified GCE. Reproduced with permission from Ref. [247]. Copyright © 2018 Elsevier Ltd. b Schematic presentation of the synthesis of the NHGH/NiCo2O4 electro-catalyst for non-enzymatic glucose sensing, Reproduced with permission from Ref. [38]. Copyright © 2019 Elsevier B.V.
Another way of preventing the aggregation of graphene sheets, which reduces the specific surface area and inhibits the fast mass transfer, is the nitrogen doping. This nitrogen doping is not only supposed to facilitates the charge transfers between adjacent carbon atoms but also suppresses the electrons and holes recombination necessary for better electrical conductivity and electrocatalytic oxidation of glucose [248, 249]. Detailed characterization revealed that in the course of hydrothermal reactions, the graphene was reduced to nitrogen-doped reduced graphene oxide when glycine acted as a source of nitrogen. Further, the nitrogen-doped reduced graphene was self-assembled into hydrogels with interconnected 3D porous network structure resulted from an increased extent of π–π stacking interactions. This 3D form provides a sufficiently large surface area and active sites for the better adsorption of the analyte species. To ascertain this, Lu et al. [38] explored the interactions of flower-like NiCo2O4 and 3D nitrogen-doped holey graphene hydrogel (NHGH)-modified GCE for electrochemical biosensing of glucose (Fig. 28b).
Similar to graphene, carbon nanofibers also possess excellent dimensional, thermal and chemical stability as well as good electrical conductivity. Recently, these fibers have attracted wide attention and have been widely explored in fields such as electrochemical cells, catalysis, adsorption, structure enhancement, biosensors, gas sensors, and nanodevices [250, 251]. Among various synthetic methods, electro-spinning is considered to be the most suitable low-cost and simple method for synthesizing carbon nanofibers [252, 253]. Liu et al. [39] explored the glucose-sensing behavior of NiCo2O4 nanoneedle-decorated electrospun carbon nanofiber nanohybrids. Faster electrocatalytic oxidation of glucose was reported for nanohybrids as compared to bare NiCo2O4 nanoneedle and electrospun carbon nanofiber-modified GCEs. The fact was supported by a large increase in the anode peak current and a positive shift in the anode peak potential.
Novel metals such as Au, Ag, and Pd, have also been used to prepare NiCo2O4 composites to improve the biosensing capabilities. Recently, dealloying has been used as a convenient method for preparing nanoporous metals with a 3D bicontinuous structure, which is characterized by open nanopores with adjustable sizes [254,255,256]. These 3D nanoporous metals act as conductive surfaces for the deposition of biosensors electrocatalytic materials such as NiCo2O4 since they provide high conductivity and large surface area. Disposable needle-type hybrid electrode comprising a stainless steel core modified with a 3D nanoporous Au/NiCo2O4 nanowall hybrid structure-modified electrochemical non-enzymatic glucose sensor showed a linear response of 0.01–21 mM glucose, high sensitivity of 0.3871 μA μM−1 cm−2, detection limit of 1 μM within a response time of < 1 s [257]. Naik et al. [258] compared the bare NiCo2O4/Ni foam, NiCo2O4–Ag/Ni foam and NiCo2O4–Au/Ni foam nanosheets electrodes. The calculated sensitivity for pure NiCo2O4, NiCo2O4–Ag, and NiCo2O4–Au nanosheets electrodes in the linear range 5–45 μM and 45–465 μM were 20.8, 29.86, and 44.86 μA μM−1 cm−2 and 6.2, 11.5, and 13.96 μA μM−1 cm−2, respectively. The respective limits of detection were 9.33, 5.82, and 2.64 μM. DFT studies confirmed strong binding between Au and NiCo2O4 as compared to Ag. Further, the binding energy of glucose was more for the NiCo2O4–Au surface compared to the NiCo2O4–Ag surface. The enhanced density of states near the Fermi level improved the conductivity of the NiCo2O4–Au nanosheet than NiCo2O4–Ag that caused superior glucose sensing performance. In a similar type of report, the sensitivities for pure NiCo2O4 and NiCo2O4–Pd nanosheets electrodes in the linear range 5–90 μM and 70–450 μM were 27.5 and 40.03 μA μM−1 cm−2 and 8.53 and 8.23 μA μM−1 cm−2, respectively [259].
Similar to metals, conducting polymers also possess the electronic, electrical, and optical properties, easy synthesis, excellent mechanical stabilities and most importantly the low toxicity and biodegradability, the issues which are generally associated with metals. Moreover, the noble metals are easily poisoned by some intermediates produced during the oxidation of glucose. Among various conducting polymers, polyaniline and polypyrrole have gained much attention due to their superior thermal and oxidative stabilities [260, 261]. Constructing a core–shell nanostructure comprising conductive polymer coating as the outer walls of metal oxides is the most important strategy for enhancing the conductivities [262]. NiCo2O4@PANI nanoparticles with an average particle size 25 nm shortened the ion transport pathway and the modified GCE exhibited a sensitivity of 4.55 mA mM−1 cm−2, a detection limit of 0.3833 μM and linear dynamic range of 0.0150–4.7350 mM (Fig. 29a, b) [263]. The PANI core–shell provided more effective electrical contact between redox-active centers and the electrolyte resulting in good contact and small diffusion distances for electron transports which subsequently improved the sensor activity. NiCo2O4@Ppy nanowires grown on Ni foam were synthesized via hydrothermal growth and oxidant-induced polymerization process (Fig. 29c–e). The fabricated glucose sensor showed high sensitivity 3059 μA mM−1 cm−2, low detection limit 0.22 μM, and wide linear dynamic range 0.001–20 mM. The excellent electrocatalytic behavior was attributed to the synergism due to bimetallic oxide, the significant role of Ppy in transmitting charges among electrode material due to its excellent conductivity, non-collapsing and non-agglomeration of the NiCo2O4 due to Ppy coating, and absence of any adhesive or conductive agent during electrode fabrication [264].
a HRTEM image of NiCo2O4@PANI nanocomposite and b CV plots for different GCE electrodes in the absence and presence of 4 mM glucose in 0.1 M NaOH solution at scan rate = 50 mV s−1. Reproduced with permission from Ref. [263]. Copyright © 2015 Elsevier B.V. c Schematic illustration of the preparation of core–shell NiCo2O4@Ppy on Ni foam substrate. d FESEM image of bare NiCo2O4. e FESEM image of NiCo2O4@Ppy composite, Reproduced with permission from Ref. [264]. Copyright © 2019 Elsevier B.V.
NiCo2O4 nano-/microstructures combined with smarter nano-architectured metal oxides (Co3O4, SnO2, NiO, and MnO2, etc.) have many synergistic multifunctional properties of nanostructured components including dense and easily accessible electroactive sites, altered bandgap energies, faster charge transfer processes, and reduced internal resistance. The p-type semiconductor nanostructured NiCo2O4 [32], Co3O4 [265], and n-type materials SnO2 [266] and MnO2 [267] have the bandgap energies of 2.1, 2.2, 3.6, and 1.3 eV, respectively. Due to slightly different bandgap energies, the semiconductor metal oxides introduce in situ impurity bands in NiCo2O4 which increase the electron conductivity to extract excellent electrocatalytic efficiencies [268]. Chen et al. [269] reported porous Co3O4 nanosheets synthesized via a simple hydrothermal method. The Co3O4 nanosheets provided the growth sites for the hydrothermal synthesis of NiCo2O4 nanorods. At the optimized conditions, porous Co3O4–NiCo2O4 nanosheet-modified GCE exhibited a preeminent sensitivity of 1463.13 μA mM−1 cm−2, a low detection limit of 0.112 μM and linear dynamic range 0.001–2.1 mM with excellent selectivity and reproducibility. The amperometric current–time plot showed a successive increase in current with the concentration of glucose (1 μM–6.1 mM) at an applied voltage of + 0.55 V using porous Co3O4–NiCo2O4 nanosheet-modified GCE. The current–concentration calibration plot displayed two linear portions with concentration ranges 1 μM–2.1 mM and 2.1–6.1 mM. Further, the incorporation of graphene into Co3O4/NiCo2O4 double-shelled nanocages was explored by Xue et al. [270]. The Co3O4/NiCo2O4 double-shelled nanocages were prepared by using zeolite imidazole frameworks-67 as a template. The amperometric studies revealed a sensitivity of 0.196 mA mM−1 cm−2 with detection limit 0.744 μM in linearized concentration range 0.01–3.52 mM, whereas, in linearized concentrations range of 0.01–3.52 mM, the sensitivity was 0.304 mA mM−1 cm−2 with detection limit 0.384 μM.
The introduction of n-type semiconductors stuffs like SnO2 in p-type NiCo2O4 semiconductors results in the formations of n–p junctions that facilitate the photo-induced electrochemical changes by altering the bandgap energies. Cai et al. [118] observed a prompt photocurrent reduction with the addition of the 100 µL–20 mM glucose solutions into the electrolyte solution. It was proposed that under sunlight stimulation, electron–hole (e−–h+) pairs are generated by the excitation of the electrons from the valence band of the n-type SnO2 semiconductor after the absorption of light of suitable energy (more than bandgap energy). The OH− of the solid electrolyte trap these h+ holes and form \({\text{OH}}^{ \cdot }\) radicals (Eq. 30). The \({\text{OH}}^{ \cdot }\) radicals are then transferred to the counter electrode to oxidize NiCo2O4 to NiOOH and CoOOH (Eq. 31). However, in the presence of glucose, positively charged h+ causes oxidation of glucose to gluconolactone. The electrons released during the oxidation process are transferred back to the valence band, so the photocurrent is decreased.
Chen et al. [271] synthesized bionics-inspired streptococcus-like mixed oxide NiCo2O4 coated on needle-like MnO2 architectures. Initially, MnO2 nanowires were synthesized via a quick precipitation method, while NiCo2O4 were grown on pre-synthesized MnO2 nanowires via a facile hydrothermal method. MnO2 nanowires prevented the agglomeration of NiCo2O4 by acting as nucleation sites and electrocatalytic centers for the uniform growth of NiCo2O4. The synergism between NiCo2O4 and MnO2 was explored for the non-enzymatic electrochemical sensing of glucose. NiCo2O4–MnO2/GCE exhibited high sensitivity, wide concentration ranges, very low detection limit, and long-term stability as compared to NiCo2O4/GCE and MnO2/GCE.
Numerous studies have been conducted to verify the selectivity of the NiCo2O4-based modified sensors as ascorbic acid, dopamine, and uric acid coexist along with glucose in human blood serum [272]. Therefore, for practical applications, these components should not affect the amperometric parameters and the positive results have been reported [230, 237, 238, 241, 247, 272, 273]. Additionally, reproducibility and stability of the modified NiCo2O4-based electrodes have been analyzed. The results have shown acceptable reproducibility with a very low relative standard deviations in many studies [123, 142, 161, 193, 199, 200, 227, 274]. The electrochemical sensing parameters such as sensitivity, linear dynamic range, and detection limits for various NiCo2O4-based modified electrodes toward glucose are compared in Table 1.
In addition to electrochemical sensing of glucose using NiCo2O4 nano-/microstructure-modified electrodes, colorimetric sensing has also been reported by Huang et al. [274]. They explored the peroxidase-like activity of the hierarchical NiCo2O4 hollow sphere which was directly dependent on the concentration of H2O2 produced by the oxidation of glucose to gluconic acid in the presence of glucose oxidase (Gox). Hence, a colorimetric method for the detection of glucose can be designed using NiCo2O4. The higher the concentration of the glucose, the more was the production of H2O2 and hence the greater was the oxidation of the 3,3,5,5-tetramethylbenzidine (TMB) to oxidized TMB. Absorbance at λmax = 652 nm for oxidized TMB was increased linearly with the concentration of glucose. The linear range was observed between 0.1 and 4.5 mM with a low detection limit of 5.31 μM (Fig. 30a, b). The corresponding reaction mechanism is shown in Fig. 30c. Intrinsic peroxidase and oxidase-like activities of the NiCo2O4 architectures were also confirmed by Su et al. [275] by analyzing the electron spin resonance spectra for the oxidation of TMB by NiCo2O4 mesoporous spheres. The oxidation was accompanied without the production of 1O2 and OH· radicals. Additionally, these peroxidase-like activities were feasible even over a broad temperature range.

Reproduced with permission from Ref. [274], Copyright © 2017 by the authors; licensee MDPI, Basel, Switzerland
a Effect of glucose concentrations on absorption. b Linearized calibration curve. c Proposed mechanism for colorimetric detection of glucose using the hierarchical NiCo2O4 hollow sphere.
4.2 H2O2 Biosensors
H2O2 is the most important byproduct produced from some enzyme-catalyzed biochemical reactions. In addition to its importance as a regulator of immune cell activation, vascular remodeling, and stomatal closure during metabolic processes, it also has pharmaceutical, clinical, environmental, textile, and food manufacturing applications [278]. Further, the concentration of H2O2 in urine is a direct indicator of the whole-body oxidative stress which is the common cause of renal failure, arteriosclerosis, myalgic, encephalomyelitis, Parkinson’s disease, diabetes mellitus, cancer and cardiovascular diseases [279]. Similar to glucose, the literature reports enzymatic as well as non-enzymatic biosensors for the detection of H2O2. Horseradish peroxidase and heme protein-based enzymatic biosensor are the most researched H2O2 biosensor due to their high sensitivity, selectivity, and biodegradability. In recent years, non-enzymatic/enzymeless H2O2 biosensors based on metal oxides have become a new class of biosensors due to fast, low-cost, and easy-to-fabrication processes [280].
In this section of the review, some non-enzymatic H2O2 biosensors based on NiCo2O4 spinel nano-/microstructures are discussed. The current–time amperometric H2O2 biosensing using modified Co3O4/NiCo2O4 nanosheets/GCE at an applied potential of − 0.35 V exhibited high sensitivity and low limit of detection of 303.42 μA mM−1 cm−2 and 0.596 μM, respectively [269]. The Co3+ ions of Co3O4 were supposed to play an important role in the sensing of H2O2. In alkaline medium, Co3+ ions reduce H2O2 to H2O (Eq. 32) [281].
The electro-reduction in H2O2 by NiCo2O4 spinel-based electrodes occurs according to Eqs. 33–36 [95, 282, 283].



Since H2O2 is reduced to H2O (O2− ions) or OH− ions as opposed to oxidation of glucose to gluconolactone, it was found that the current response during electrochemical sensing of H2O2 was reduced. Similar trends have been reported by other sensor materials such as ZnFe2O4/reduced graphene oxide [284], nickel phosphide (Ni2P) nanosheets array on titanium mesh [285], cobalt nitride (Co3N) nanowire array on Ti mesh [286], nanoporous carbon nanofibers/Pt nanoparticles [287], Ag decorated hierarchical Sn3O4 [288] and many more for the electrochemical sensing of H2O2 amperometrically.
Xiao et al. [289] reported that NiCo2O4 mixed oxide-based electrodes in the alkaline medium can cause electro-reduction as well as electro-oxidation toward H2O2. The extraordinary variety of inter-convertible oxidation states of Co and Ni in spinel NiCo2O4 is the key factor for its oxidizing and reducing nature. High valence Co3+ and Ni3+ ions of NiCo2O4 can be reduced to lower + 2 oxidation states, i.e., into Co2+ and Ni2+ ions. Similarly, lower + 2 oxidation states can also be oxidized to higher valencies including Co3+, Co4+, and Ni3+ ions. Other factors which decide the electro-reduction and electro-oxidation behavior of the NiCo2O4 toward H2O2 are the pH and the concentration of the H2O2 in the medium. At a scan rate of 10 mV s−1, H2O2 electro-reduction and electro-oxidation were observed for 0.4 M H2O2 in 3.0 M and 0.75 M H2O2 in 3.0 M KOH, respectively. Equation 37 represents the overall electro-oxidation of H2O2 in an alkaline medium.
The electrons lost during the oxidation of H2O2 reduce the trivalent cations (Co3+ and Ni3+) ions to their divalent states.
Xue et al. [290] grew ZnO nanowires on Ni foam via a galvanostatic electro-deposition technique. After that, the Ni foam-supported ZnO nanowires and Co3O4/NiCo2O4 double-shelled nanocages were prepared by coprecipitation and annealing processes. The ZnO/Co3O4/NiCo2O4/Ni foam-based electrochemical H2O2 sensor exhibited a high sensitivity of 388 μA mM−1 cm−2, the low detection limit of 0.163 μM, and a dynamic linear range concentration of 0.2 μM–2.4 mM with a fast response time of 5 s. The fast and high response was attributed to the fast electron transport and short electrical pathway provided by ZnO nanowires. Additionally, Co3O4/NiCo2O4 double-shelled nanocages provided sufficient mesopores and large specific surface area for improved H2O2 sensing [290]. Sakthivel et al. [291] compared the electrochemical kinetics of modified NiCo2O4/GCE, NiCo2S4/GCE, and NiCoSe2/GCE toward H2O2. The modified NiCoSe2/GCE showed better electrochemical sensing behavior for H2O2 than modified NiCo2O4/GCE and NiCo2S4/GCE. The greater electrocatalytic efficiency of modified NiCoSe2/GCE was attributed to the large electrochemically active surface area for hydrothermally synthesized NiCoSe2.
4.3 Urea Biosensors
Urea (carbamide or carbonyl diamide) is one of the main end products of protein metabolism in humans and animals. Urea is exclusively formed in the liver, and is transported by the bloodstream to the kidneys for excretion in the human body. The normal level of urea in human blood serum is 2.5–7.5 mM [292,293,294,295]. Amount of urea above or below the permissible level in the serum results in chronic renal and hepatic failure, gastrointestinal bleeding, and nephritic syndrome [296]. Similar to other metabolically important biomolecules, the literature reports enzymatic as well as non-enzymatic biosensors for the selective and highly sensitive urea sensors. Enzyme-based urea biosensors explore the use of urease enzyme which facilitates the hydrolysis of urea into ammonium (\({\text{NH}}_{ 4}^{ + }\)) and bicarbonate (\({\text{HCO}}_{ 3}^{ - }\)) ions (Eq. 38) [297].
However, in this section, some non-enzymatic-modified urea sensor electrodes based on spinel NiCo2O4 nano-/microstructures are reviewed. Research has proved that urea can be electrochemically oxidized by NiCo2O4 nano-/microstructures (Eqs. 39–41).
The overall reaction can be written as:
Recently, Amin et al. [298] explored the urea sensing behavior of NiCo2O4 nanoneedle-modified GCE which showed high sensitivity with a linear response concentration range of 0.01–5 mM and low detection limit of 1.0 µM. It was proposed that initially Ni2+ ions are oxidized to Ni3+ ions to form NiOOH in an alkaline medium which is reduced back to give Ni(OH)2 at the time of urea electro-oxidation [299]. Mesoporous spinel NiCo2O4 nanostructures prepared via facile chemical deposition method showed higher catalytic activities, lower overpotential, and more tolerance toward urea electro-oxidation as compared to Co3O4 [227]. NiCo2O4/3D graphene/ITO exhibited high sensitivity of 166 μA mM−1 cm−2, a linear range of 0.06–0.30 mM, and a low detection limit of 5.0 µM with a very small response time of 1 s through non-enzymatic detection method [300]. Further, a higher oxidation peak for NiCo2O4/3D graphene in the CV as compared to NiCo2O4/CNT-modified electrode confirmed the superiority of 3D graphene as a matrix material for electrode fabrication. The higher oxidation current potential was attributed to the highly porous nature and excellent conductivity of the interconnected 3D graphene matrix [301]. Since oxidation of urea is in alkaline medium, higher electrocatalytic oxidation of urea is recorded at higher pH conditions. However, beyond an optimum pH the electro-oxidation decreases due to blockage of the active sites by OH− ions [302].
4.4 Electrochemical Determination of Some Other Bioanalytes
Some other bioanalytes such as rutin, trypsin, ascorbic acid, dopamine, uric acid, and tryptophan have also been electrochemically analyzed using nano-/micro-structured hybrid NiCo2O4-modified electrodes. Rutin, a flavonoid substance, is used as anti-bacterial, anti-viral, antiproliferative, antioxidants, antagonists, and anti-inflammatory. It also controls the blood pressure and vascular fragility including hereditary hemorrhagic telangiectasia, haemangiomas, vitamin C deficiency, etc. [303, 304]. Cui et al. [305] reported the fabrication of GCE modified with mesoporous NiCo2O4-decorated reduced graphene oxide for the electrochemical sensing of rutin using differential pulse voltammetric (DPV) technique. Flake-like NiCo2O4 sheets anchored on the wrinkled reduced graphene oxide sheets through electrostatic interaction prevented the self-agglomerations. The wide linear range of 0.1–150 μM and a low detection limit of 0.01 μM were observed along with excellent anti-interference capabilities. The strong synergism between reduced graphene sheets and mesoporous NiCo2O4 resulted in increased redox peak current and decreased potential difference. During electro-oxidation, rutin is converted into 3′,4′-diquinone with the release of two H+ ions and two electrons (Eq. 42) [306, 307].

Trypsin, a serine protease secreted from the pancreas, has also been widely studied recently as it used for identifying and determining the amino acid sequence in protein and peptide, particularly at the C-terminus and as a specific biomarker for diseases like chronic cystic fibrosis, chronic pancreatitis, cancer, and many pathological changes [308, 309]. Lin et al. [310] reported a large and prompt rise in electrochemical signal in the presence of trypsin by NiCo2O4- poly(amidoamine)/peptide@g-C3N4 nanocomposite-modified GCE. 3,4,9,10-perylene tetracarboxylic acid (PTCA) was used to connect the peptides and g-C3N4. The modified GCE exhibited increased DPVs peak currents when the trypsin concentration was increased from 10−10 to 10−4 mg mL−1. Kaur et al. [311] studied the simultaneous electrochemical sensing of ascorbic acid, dopamine, uric acid, and tryptophan using NiCo2O4/Nano-ZSM-5 nanocomposite-modified GCE. Wide linear ranges were 1–1200, 0.6–900, 0.9–1000, and 0.9–1000 μM, while the corresponding detection limits were 0.8, 0.5, 0.7, and 0.7 μM for ascorbic acid, dopamine, uric acid, and tryptophan, respectively. Simultaneous detection was possible as the anodic oxidative peak currents were observed at different applied potentials, i.e., 0.158, 0.394, 0.561, and 0.820 V, respectively, for ascorbic acid, dopamine, uric acid, and tryptophan in DVP plots at a scan rate of 20 mV s−1.
Detailed comparison from Tables 1 and 2 indicates that the morphology of the NiCo2O4 nano-/microstructures and the presence of any other component along with NiCo2O4 significantly affect the biosensing efficiency. Comparative analysis revealed better electrochemical sensing of glucose by one-dimensional nanofibres and nanorods and two-dimensional nanosheets like morphologies of NiCo2O4 than other morphologies. Doped and composites/hybrid NiCo2O4 nano-/microstructures exhibit superior sensing parameters that bare NiCo2O4. In particular, graphenic nanomaterials due to their excellent conductivity and large surface area significantly elevate the I–V characteristics to many folds. These materials also accelerate the rate of heterogeneous electron transfer, i.e., the transfer of electrons from/to electrode to/from bioanalyte molecules [312].
5 Conclusion
Herein, various strategies for the synthesis of spinel NiCo2O4 nano-/microstructures with versatile morphologies and their subsequent use for the development of biosensors for efficient non-enzymatic sensing and detection of biomolecules such as glucose, H2O2 and urea are comprehensively reviewed. As compared to NiO and Co3O4, the NiCo2O4 nanomaterials showed better electrochemical sensing as adjudged by broader redox peaks with larger area coverage in the CV curves. The biosensing efficiency of the NiCo2O4 nano-/microstructures can be improved by engineering the morphology, specific surface area, porosity, doping and by making composite/hybrids with various carbonaceous materials, conducting polymers, metal oxides, non-metals and metals. These materials not only improve the mechanical, thermal, and chemical stability but also modulate the bandgap energies, electronic and ionic conductivities, dispersion behavior, avoid aggregation of the NiCo2O4 nanomaterials and provide short electron and ion diffusion pathways. All these factors contribute to better electrocatalytic behavior with excellent sensitivity, selectivity, and long-term stability of the spinel NiCo2O4 nano-/microstructure-based biosensors. It is hoped that this review will provide basic ideas as well as new insights for future research and progress in this field.
6 Challenges and Future Perspectives
Despite extensive research in this area, many issues that impede the practical application of NiCo2O4nano-/microstructures need to be addressed for further improvement. Some of these issues have been identified herein.
Structural features of the NiCo2O4 nanomaterials are controlled by factors like temperature, pH of the reaction solution, precursor concentration, solvent nature and quantity, presence of the growth directing agents and templates, etc. It is, therefore, one of the major challenges to design large-scale and low-cost morphology controlled synthesis of the NiCo2O4 nanomaterials for next-generation biosensors.
Rational combination of NiCo2O4 nano-/microstructures with other hybrid materials or conductive substrates to designing NiCo2O4 composite/hybrids is found to improve the intrinsic characteristics like low electronic conductivity and wide bandgap and hence the biosensing behavior of NiCo2O4. However, still, more in-depth understanding is required to correlate the synergism between the components of the composite/hybrid materials.
Cost-effectiveness, easy to manufacture, recyclability, sensor disposal, and biocompatibility of NiCo2O4 nano-/microstructure-based biosensors are other issues that need to be addressed and solved. The high cost of electrochemical work stations restricts the practical applications of these sensors. In this regard, portable and wearable sensing devices will be promising. The toxicity issues of the NiCo2O4 nano-/microstructures and other components are very rarely discussed in the literature. Future research thus should also focus on studying this important issue.
The biosensing behavior of the NiCo2O4 nano-/microstructure-based sensors is affected by factors like working temperature, pH of the medium, scan rates, and applied potential. The optimization of these parameters is rarely addressed. Studying the specificity of NiCo2O4-based sensors from competitive assays requires an appropriate protocol because it is reported that the sensor can detect glucose, H2O2, urea, trypsin, etc.
Due to complex structures of the biomolecules, the interface and mechanistic studies at the surface of the nanomaterials are still undefined. The redox transformation of the molecules during electrocatalytic biosensing is also a debatable issue. Therefore, future work should focus on elucidating the interaction mechanism between nanomaterials and biomolecules on the electrode surface, to fabricate a new generation of biosensors.
References
C. Shenghai, S. Liping, K. Fanhao, H. Lihua, Z. Hui, Carbon-coated MnCo2O4 nanowire as bifunctional oxygen catalysts for rechargeable Zn–air batteries. J. Power Sources 430, 25–31 (2019). https://doi.org/10.1016/j.jpowsour.2019.05.029
H. Hou, H. Shao, X. Zhang, G. Liu, S. Hussain, G. Qiao, RGO-loaded flower-like ZnCo2O4 nanohybrid as counter electrode for dye-sensitized solar cells. Mater. Lett. 225, 5–8 (2018). https://doi.org/10.1016/j.matlet.2018.04.103
R. Shi, Y. Zhang, Z. Wang, Facile synthesis of a ZnCo2O4 electrocatalyst with three-dimensional architecture for methanol oxidation. J. Alloys Compd. 810, 151879 (2019). https://doi.org/10.1016/j.jallcom.2019.151879
Z. Li, M. Ye, A. Han, H. Du, Preparation, characterization and microwave absorption properties of NiFe2O4 and its composites with conductive polymer. J. Mater. Sci. Mater. Electron. 27, 1031–1043 (2016). https://doi.org/10.1007/s10854-015-3848-8
L. Li, G. Jiang, J. Ma, CuMn2O4/graphene nanosheets as excellent anode for lithium-ion battery. Mater. Res. Bull. 104, 53–59 (2018). https://doi.org/10.1016/j.materresbull.2018.03.051
P. Li, W. Sun, Q. Yu, P. Yang, J. Qiao, Z. Wang, D. Rooney, K. Sun, An effective three-dimensional ordered mesoporous CuCo2O4 as electrocatalyst for Li–O2 batteries. Solid State Ion. 289, 17–22 (2016). https://doi.org/10.1016/j.ssi.2016.02.014
Y. Jin, L. Wang, Q. Jiang, X. Du, C. Ji, X. He, Morphology controllable synthesis of CoMn2O4 structures by adjusting the urea concentration: from microflowers to microspheres. Mater. Lett. 168, 166–170 (2016). https://doi.org/10.1016/j.matlet.2016.01.077
F. Du, X. Zuo, Q. Yang, B. Yang, G. Li et al., The stabilization of NiCo2O4 nanobelts used for catalyzing triiodides in dye-sensitized solar cells by the presence of RGO sheets. Sol. Energy Mater. Sol. Cells 149, 9–14 (2016). https://doi.org/10.1016/j.solmat.2015.11.025
L. Manjakkal, D. Szwagierczak, R. Dahiya, Metal oxides based electrochemical pH sensors: current progress and future perspectives. Prog. Mater. Sci. 109, 100635 (2020). https://doi.org/10.1016/j.pmatsci.2019.100635
X. Han, X. Gui, T.F. Yi, Y. Li, C. Yue, Recent progress of NiCo2O4-based anodes for high-performance lithium-ion batteries. Curr. Opin. Solid State Mater. Sci. 22, 109–126 (2018). https://doi.org/10.1016/j.cossms.2018.05.005
Y. Li, X. Han, T. Yi, Y. He, X. Li, Review and prospect of NiCo2O4-based composite materials for supercapacitor electrodes. J. Energy Chem. 31, 54–78 (2019). https://doi.org/10.1016/j.jechem.2018.05.010
Q. Wang, B. Liu, X. Wang, S. Ran, L. Wang, D. Chen, G. Shen, Morphology evolution of urchin-like NiCo2O4 nanostructures and their applications as psuedocapacitors and photoelectrochemical cells. J. Mater. Chem. 22, 21647–21653 (2012). https://doi.org/10.1039/c2jm34705a
R.R. Owings, G.J. Exarhos, C.F. Windisch, P.H. Holloway, J.G. Wen, Process enhanced polaron conductivity of infrared transparent nickel–cobalt oxide. Thin Solid Films 483, 175–184 (2005). https://doi.org/10.1016/j.tsf.2005.01.011
X. Zhao, L. Mao, Q. Cheng, J. Li, F. Liao, G. Yang, L. Xie, C. Zhao, L. Chen, Two-dimensional spinel structured co-based materials for high performance supercapacitors: a critical review. Chem. Eng. J. 387, 124081 (2020). https://doi.org/10.1016/j.cej.2020.124081
K. Choi, I.K. Moon, J. Oh, An efficient amplification strategy for N-doped NiCo2O4 with oxygen vacancies and partial Ni/Co-nitrides as a dual-function electrode for both supercapatteries and hydrogen electrocatalysis. J. Mater. Chem. A 7, 1468–1478 (2019). https://doi.org/10.1039/c8ta07210h
Y. Ha, L. Shi, X. Yan, Z. Chen, Y. Li, W. Xu, R. Wu, Multifunctional electrocatalysis on a porous N-doped NiCo2O4@C nanonetwork. ACS Appl. Mater. Interfaces 11, 45546–45553 (2019). https://doi.org/10.1021/acsami.9b13580
W. Chu, Z. Shi, Y. Hou, D. Ma, X. Bai, Y. Gao, N. Yang, Trifunctional of phosphorus-doped NiCo2O4 nanowire materials for asymmetric supercapacitor, oxygen evolution reaction, and hydrogen evolution reaction. ACS Appl. Mater. Interfaces 12, 2763–2772 (2020). https://doi.org/10.1021/acsami.9b13182
X. Hu, M. Huang, X. Meng, X. Ju, Fabrication of uniform urchin-like N-doped NiCo2O4@C hollow nanostructures for high performance supercapacitors. RSC Adv. 9, 42110–42119 (2019). https://doi.org/10.1039/c9ra07678f
J. Zhang, Y. Chen, R. Chu, H. Jiang, Y. Zeng, Y. Zhang, N.M. Huang, H. Guo, Pseudocapacitive P-doped NiCo2O4 microspheres as stable anode for lithium ion batteries. J. Alloys Compd. 787, 1051–1062 (2019). https://doi.org/10.1016/j.jallcom.2018.12.275
J.H. Lin, Y.T. Yan, T.X. Xu, C.Q. Qu, J. Li, J. Cao, J.C. Feng, J.L. Qi, S doped NiCo2O4 nanosheet arrays by Ar plasma: an efficient and bifunctional electrode for overall water splitting. J. Colloid Interface Sci. 560, 34–39 (2020). https://doi.org/10.1016/j.jcis.2019.10.056
M. Yang, Y. Li, Y. Yu, X. Liu, Z. Shi, Y. Xing, Self-assembly of three-dimensional zinc-doped NiCo2O4 as efficient electrocatalysts for oxygen evolution reaction. Chem. A Eur. J. 24, 13002–13008 (2018). https://doi.org/10.1002/chem.201802325
K.L. Yan, X. Shang, Z. Li, B. Dong, X. Li et al., Ternary mixed metal Fe-doped NiCo2O4 nanowires as efficient electrocatalysts for oxygen evolution reaction. Appl. Surf. Sci. 416, 371–378 (2017). https://doi.org/10.1016/j.apsusc.2017.04.204
L. Liu, H. Zhang, L. Fang, Y. Mu, Y. Wang, Facile preparation of novel dandelion-like Fe-doped NiCo2O4 microspheres@nanomeshes for excellent capacitive property in asymmetric supercapacitors. J. Power Sources 327, 135–144 (2016). https://doi.org/10.1016/j.jpowsour.2016.07.054
J. Ma, E. Guo, L. Yin, Porous hierarchical spinel Mn-doped NiCo2O4 nanosheet architectures as high-performance anodes for lithium-ion batteries and electrochemical reaction mechanism. J. Mater. Sci. Mater. Electron. 30, 8555–8567 (2019). https://doi.org/10.1007/s10854-019-01176-5
W.Y. Xia, N. Li, Q.Y. Li, K.H. Ye, C.W. Xu, Au–NiCo2O4 supported on three-dimensional hierarchical porous graphene-like material for highly effective oxygen evolution reaction. Sci. Rep. 6, 23398 (2016). https://doi.org/10.1038/srep23398
C. Wei, X. Zheng, R. Li, X. Wang, Z. Xaio, L. Wang, Mesoporous hybrid NiCo2O4/CeO2 hierarchical hollow spheres for enhanced supercapacitors. ChemistrySelect 4, 11149–11155 (2019). https://doi.org/10.1002/slct.201902778
C. Wei, R. Zhang, X. Zheng, Q. Ru, Q. Chen, C. Cui, G. Li, D. Zhang, Hierarchical porous NiCo2O4/CeO2 hybrid materials for high performance supercapacitors. Inorg. Chem. Front. 5, 3126–3134 (2018). https://doi.org/10.1039/c8qi01010b
S.K. Shinde, M.B. Jalak, G.S. Ghodake, N.C. Maile, H.M. Yadav et al., Flower-like NiCo2O4/NiCo2S4 electrodes on Ni mesh for higher supercapacitor applications. Ceram. Int. 45, 17192–17203 (2019). https://doi.org/10.1016/j.ceramint.2019.05.274
B. Ge, K. Li, Z. Fu, L. Pu, X. Zhang, Z. Liu, K. Huang, The performance of nano urchin-like NiCo2O4 modified activated carbon as air cathode for microbial fuel cell. J. Power Sources 303, 325–332 (2016). https://doi.org/10.1016/j.jpowsour.2015.11.003
W. Wang, X. Song, C. Gu, D. Liu, J. Liu, J. Huang, A high-capacity NiCo2O4@reduced graphene oxide nanocomposite Li-ion battery anode. J. Alloys Compd. 741, 223–230 (2018). https://doi.org/10.1016/j.jallcom.2018.01.097
Y.Q. Zhang, M. Li, B. Hua, Y. Wang, Y.F. Sun, J.L. Luo, A strongly cooperative spinel nanohybrid as an efficient bifunctional oxygen electrocatalyst for oxygen reduction reaction and oxygen evolution reaction. Appl. Catal. B Environ. 236, 413–419 (2018). https://doi.org/10.1016/j.apcatb.2018.05.047
L. Hu, L. Wu, M. Liao, X. Fang, High-performance NiCo2O4 nanofilm photodetectors fabricated by an interfacial self-assembly strategy. Adv. Mater. 23, 1988–1992 (2011). https://doi.org/10.1002/adma.201004109
M. Silambarasan, P.S. Ramesh, D. Geetha, Spinel NiCo2O4 nanostructures: synthesis, morphological, optical and electrochemical properties, in Recent Trends in Materials Science and Applications. Springer Proceedings in Physics, vol. 189, ed. by J. Ebenezar (Springer, Cham, 2017), pp. 219–231. https://doi.org/10.1007/978-3-319-44890-9_21
A. Bashir, S. Shukla, R. Bashir, R. Patidar, A. Bruno, D. Gupta, M.S. Satti, Z. Akhter, Low temperature, solution processed spinel NiCo2O4 nanoparticles as efficient hole transporting material for mesoscopic n-i-p perovskite solar cells. Sol. Energy 196, 367–378 (2020). https://doi.org/10.1016/j.solener.2019.12.031
H. Zhang, Y. Chen, RGO loaded porous NiCo2O4 nanoplates as alcohol gas sensors. Nanosci. Nanotechnol. Lett. 9, 374–379 (2017). https://doi.org/10.1166/nnl.2017.2341
B. Zhang, F. Qu, X. Zhou, S. Zhang, T. Thomas, M. Yang, Porous coral-like NiCo2O4 nanospheres with promising xylene gas sensing properties. Sens. Actuators B Chem. 261, 203–209 (2018). https://doi.org/10.1016/j.snb.2018.01.125
N. Joshi, L.F. Da Silva, H. Jadhav, J.C. M’Peko, B.B. Millan Torres, K. Aguir, V.R. Mastelaro, O.N. Oliveira, One-step approach for preparing ozone gas sensors based on hierarchical NiCo2O4 structures. RSC Adv. 6, 92655–92662 (2016). https://doi.org/10.1039/c6ra18384k
Z. Lu, L. Wu, J. Zhang, W. Dai, G. Mo, J. Ye, Bifunctional and highly sensitive electrochemical non-enzymatic glucose and hydrogen peroxide biosensor based on NiCo2O4 nanoflowers decorated 3D nitrogen doped holey graphene hydrogel. Mater. Sci. Eng. C 102, 708–717 (2019). https://doi.org/10.1016/j.msec.2019.04.072
L. Liu, Z. Wang, J. Yang, G. Liu, J. Li, L. Guo, S. Chen, Q. Guo, NiCo2O4 nanoneedle-decorated electrospun carbon nanofiber nanohybrids for sensitive non-enzymatic glucose sensors. Sens. Actuators. B Chem. 258, 920–928 (2018). https://doi.org/10.1016/j.snb.2017.11.118
B. Li, J. Feng, Y. Qian, S. Xiong, Mesoporous quasi-single-crystalline NiCo2O4 superlattice nanoribbons with optimizable lithium storage properties. J. Mater. Chem. A 3, 10336–10344 (2015). https://doi.org/10.1039/c5ta01229e
Y. Zhu, X. Pu, W. Song, Z. Wu, Z. Zhou et al., High capacity NiCo2O4 nanorods as electrode materials for supercapacitor. J. Alloys Compd. 617, 988–993 (2014). https://doi.org/10.1016/j.jallcom.2014.08.064
J. Li, S. Xiong, Y. Liu, Z. Ju, Y. Qian, High electrochemical performance of monodisperse NiCo2O4 mesoporous microspheres as an anode material for Li-ion batteries. ACS Appl. Mater. Interfaces 5, 981–988 (2013). https://doi.org/10.1021/am3026294
R. Batool, A. Rhouati, M.H. Nawaz, A. Hayat, J.L. Marty, A review of the construction of nano-hybrids for electrochemical biosensing of glucose. Biosensors 9(1), 46 (2019). https://doi.org/10.3390/bios9010046
P. Mehrotra, Biosensors and their applications—a review. J. Oral Biol. Craniofac. Res. 6, 153–159 (2016). https://doi.org/10.1016/j.jobcr.2015.12.002
H. Lu, S. Zhang, L. Guo, W. Li, Applications of graphene-based composite hydrogels: a review. RSC Adv. 7, 51008–51020 (2017). https://doi.org/10.1039/c7ra09634h
R. Bhadoria, H.S. Chaudhary, Recent advances of biosensors in biomedical sciences. Int. J. Drug Deliv. 3, 571 (2011). https://doi.org/10.3389/fbioe.2016.00011
J. Peña-Bahamonde, H.N. Nguyen, S.K. Fanourakis, D.F. Rodrigues, Recent advances in graphene-based biosensor technology with applications in life sciences. J. Nanobiotechnol. 16, 75 (2018). https://doi.org/10.1186/s12951-018-0400-z
S. Yin, Z. Jin, T. Miyake, Wearable high-powered biofuel cells using enzyme/carbon nanotube composite fibers on textile cloth. Biosens. Bioelectron. 141, 111471 (2019). https://doi.org/10.1016/j.bios.2019.111471
B.S. Dakshayini, K.R. Reddy, A. Mishra, N.P. Shetti, S.J. Malode, S. Basu, S. Naveen, A.V. Raghu, Role of conducting polymer and metal oxide-based hybrids for applications in ampereometric sensors and biosensors. Microchem. J. 147, 7–24 (2019). https://doi.org/10.1016/j.microc.2019.02.061
N.P. Shetti, S.J. Malode, D. Ilager, K. Raghava Reddy, S.S. Shukla, T.M. Aminabhavi, A novel electrochemical sensor for detection of molinate using ZnO nanoparticles loaded carbon electrode. Electroanalysis 31, 1040–1049 (2019). https://doi.org/10.1002/elan.201800775
G. Wang, X. He, L. Wang, A. Gu, Y. Huang, B. Fang, B. Geng, X. Zhang, Non-enzymatic electrochemical sensing of glucose. Microchim. Acta 180, 161–186 (2013). https://doi.org/10.1007/s00604-012-0923-1
S. Park, H. Boo, T.D. Chung, Electrochemical non-enzymatic glucose sensors. Anal. Chim. Acta 556, 46–57 (2006). https://doi.org/10.1016/j.aca.2005.05.080
X. Hui, X. Xuan, J. Kim, J.Y. Park, A highly flexible and selective dopamine sensor based on Pt–Au nanoparticle-modified laser-induced graphene. Electrochim. Acta 328, 135066 (2019). https://doi.org/10.1016/j.electacta.2019.135066
R. Ahmad, N. Tripathy, M.S. Ahn, K.S. Bhat, T. Mahmoudi et al., Highly efficient non-enzymatic glucose sensor based on CuO modified vertically-grown ZnO nanorods on electrode. Sci. Rep. 7, 5715 (2017). https://doi.org/10.1038/s41598-017-06064-8
R.J. Chung, A.N. Wang, Q.L. Liao, K.Y. Chuang, Non-enzymatic glucose sensor composed of carbon-coated nano-zinc oxide. Nanomaterials 7(2), 36 (2017). https://doi.org/10.3390/nano7020036
N.P. Shetti, S.J. Malode, D.S. Nayak, R.R. Naik, G.T. Kuchinad, K.R. Reddy, S.S. Shukla, T.M. Aminabhavi, Hetero nanostructured iron oxide and bentonite clay composite assembly for the determination of an antiviral drug acyclovir. Microchem. J. 155, 104727 (2020). https://doi.org/10.1016/j.microc.2020.104727
C. Wang, E. Zhou, W. He, X. Deng, J. Huang et al., NiCo2O4-based supercapacitor nanomaterials. Nanomaterials 7(2), 41 (2017). https://doi.org/10.3390/nano7020041
J.P. Cheng, W.D. Wang, X.C. Wang, F. Liu, Recent research of core–shell structured composites with NiCo2O4 as scaffolds for electrochemical capacitors. Chem. Eng. J. 393, 124747 (2020). https://doi.org/10.1016/j.cej.2020.124747
H. Osgood, S.V. Devaguptapu, H. Xu, J. Cho, G. Wu, Transition metal (Fe Co, Ni, and Mn) oxides for oxygen reduction and evolution bifunctional catalysts in alkaline media. Nano Today 11, 601–625 (2016). https://doi.org/10.1016/j.nantod.2016.09.001
S.S. Tong, X.J. Wang, Q.C. Li, X.J. Han, Progress on electrocatalysts of hydrogen evolution reaction based on carbon fiber materials. Chin. J. Anal. Chem. 44, 1447–1457 (2016). https://doi.org/10.1016/S1872-2040(16)60958-1
N.P. Shetti, D.S. Nayak, S.J. Malode, R.R. Kakarla, S.S. Shukla, T.M. Aminabhavi, Sensors based on ruthenium-doped TiO2 nanoparticles loaded into multi-walled carbon nanotubes for the detection of flufenamic acid and mefenamic acid. Anal. Chim. Acta 1051, 58–72 (2019). https://doi.org/10.1016/j.aca.2018.11.041
S.D. Bukkitgar, N.P. Shetti, R.M. Kulkarni, K.R. Reddy, S.S. Shukla, V.S. Saji, T.M. Aminabhavi, Electro-catalytic behavior of Mg-doped ZnO nano-flakes for oxidation of anti-inflammatory drug. J. Electrochem. Soc. 166, B3072 (2019). https://doi.org/10.1149/2.0131909jes
C. Sabu, T.K. Henna, V.R. Raphey, K.P. Nivitha, K. Pramod, Advanced biosensors for glucose and insulin. Biosens. Bioelectron. 141, 111201 (2019). https://doi.org/10.1016/j.bios.2019.03.034
C.V. Reddy, I.N. Reddy, K.R. Reddy, S. Jaesool, K. Yoo, Template-free synthesis of tetragonal Co-doped ZrO2 nanoparticles for applications in electrochemical energy storage and water treatment. Electrochim. Acta 317, 416–426 (2019). https://doi.org/10.1016/j.electacta.2019.06.010
P.S. Basavarajappa, S.B. Patil, N. Ganganagappa, K.R. Reddy, A.V. Raghu, C.V. Reddy, Recent progress in metal-doped TiO2, non-metal doped/codoped TiO2 and TiO2 nanostructured hybrids for enhanced photocatalysis. Int. J. Hydrogen Energy 45, 7764–7778 (2020). https://doi.org/10.1016/j.ijhydene.2019.07.241
S.B. Patil, P.S. Basavarajappa, N. Ganganagappa, M.S. Jyothi, A.V. Raghu, K.R. Reddy, Recent advances in non-metals-doped TiO2 nanostructured photocatalysts for visible-light driven hydrogen production, CO2 reduction and air purification. Int. J. Hydrogen Energy 44, 13022–13039 (2019). https://doi.org/10.1016/j.ijhydene.2019.03.164
C. Chen, Q. Xie, D. Yang, H. Xiao, Y. Fu, Y. Tan, S. Yao, Recent advances in electrochemical glucose biosensors: a review. RSC Adv. 3, 4473–4491 (2013). https://doi.org/10.1039/c2ra22351a
D.W. Hwang, S. Lee, M. Seo, T.D. Chung, Recent advances in electrochemical non-enzymatic glucose sensors—a review. Anal. Chim. Acta 1033, 1–34 (2018). https://doi.org/10.1016/j.aca.2018.05.051
A. Jing, G. Liang, H. Shi, Y. Yuan, Q. Zhan, W. Feng, Three-dimensional holey-graphene architectures for highly sensitive enzymatic electrochemical determination of hydrogen peroxide. J. Nanosci. Nanotechnol. 19, 7404–7409 (2019). https://doi.org/10.1166/jnn.2019.16613
J.C. Chou, C.Y. Wu, Y.H. Liao, C.H. Lai, S.J. Yan, Y.X. Wu, S.H. Lin, Enzymatic urea sensor based on graphene oxide/titanium dioxide films modified by urease-magnetic beads. IEEE Trans. Nanotechnol. 18, 336–344 (2019). https://doi.org/10.1109/TNANO.2019.2907225
E. Zapp, D. Brondani, I.C. Vieira, J. Dupont, C.W. Scheeren, Bioelectroanalytical determination of rutin based on bi-enzymatic sensor containing iridium nanoparticles in ionic liquid phase supported in clay. Electroanalysis 23, 764–776 (2011). https://doi.org/10.1002/elan.201000619
M. Kameya, H. Onaka, Y. Asano, Selective tryptophan determination using tryptophan oxidases involved in bis-indole antibiotic biosynthesis. Anal. Biochem. 438, 124–132 (2013). https://doi.org/10.1016/j.ab.2013.03.024
K.R. Reddy, B.C. Sin, C.H. Yoo, W. Park, K.S. Ryu, J.S. Lee, D. Sohn, Y. Lee, A new one-step synthesis method for coating multi-walled carbon nanotubes with cuprous oxide nanoparticles. Scr. Mater. 58, 1010–1013 (2008). https://doi.org/10.1016/j.scriptamat.2008.01.047
N.L. Reddy, V.N. Rao, M. Vijayakumar, R. Santhosh, S. Anandan et al., A review on frontiers in plasmonic nano-photocatalysts for hydrogen production. Int. J. Hydrogen Energy 44, 10453–10472 (2019). https://doi.org/10.1016/j.ijhydene.2019.02.120
C. Venkata Reddy, I.N. Reddy, B. Akkinepally, K.R. Reddy, J. Shim, Synthesis and photoelectrochemical water oxidation of (Y, Cu) codoped α-Fe2O3 nanostructure photoanode. J. Alloys Compd. 814, 152349 (2020). https://doi.org/10.1016/j.jallcom.2019.152349
N.P. Shetti, S.D. Bukkitgar, K.R. Reddy, C.V. Reddy, T.M. Aminabhavi, Nanostructured titanium oxide hybrids-based electrochemical biosensors for healthcare applications. Colloids Surf. B: Biointerfaces 178, 385–394 (2019). https://doi.org/10.1016/j.colsurfb.2019.03.013
S. Kumar, S.D. Bukkitgar, S. Singh, Pratibha, V. Singh et al., Electrochemical sensors and biosensors based on graphene functionalized with metal oxide nanostructures for healthcare applications. ChemistrySelect 4, 5322–5337 (2019). https://doi.org/10.1002/slct.201803871
N.P. Shetti, D.S. Nayak, S.J. Malode, K.R. Reddy, S.S. Shukla, T.M. Aminabhavi, Electrochemical behavior of flufenamic acid at amberlite XAD-4 resin and silver-doped titanium dioxide/amberlite XAD-4 resin modified carbon electrodes. Colloids Surf. B: Biointerfaces 177, 407–415 (2019). https://doi.org/10.1016/j.colsurfb.2019.02.022
K.R. Reddy, V.G. Gomes, M. Hassan, Carbon functionalized TiO2 nanofibers for high efficiency photocatalysis. Mater. Res. Express 1, 15012 (2014). https://doi.org/10.1088/2053-1591/1/1/015012
D. Kishore Kumar, J. Loskot, J. Kříž, N. Bennett, H.M. Upadhyaya, V. Sadhu, C. Venkata Reddy, K.R. Reddy, Synthesis of SnSe quantum dots by successive ionic layer adsorption and reaction (SILAR) method for efficient solar cells applications. Sol. Energy 199, 570–574 (2020). https://doi.org/10.1016/j.solener.2020.02.050
N.R. Reddy, U. Bhargav, M.M. Kumari, K.K. Cheralathan, M.V. Shankar, K.R. Reddy, T.A. Saleh, T.M. Aminabhavi, Highly efficient solar light-driven photocatalytic hydrogen production over Cu/FCNTs-titania quantum dots-based heterostructures. J. Environ. Manag. 254, 109747 (2020). https://doi.org/10.1016/j.jenvman.2019.109747
C.V. Reddy, I.N. Reddy, V.V.N. Harish, K.R. Reddy, N.P. Shetti, J. Shim, T.M. Aminabhavi, Efficient removal of toxic organic dyes and photoelectrochemical properties of iron-doped zirconia nanoparticles. Chemosphere 239, 124766 (2020). https://doi.org/10.1016/j.chemosphere.2019.124766
V. Navakoteswara Rao, N. Lakshmana Reddy, M. Mamatha Kumari, P. Ravi, M. Sathish et al., Photocatalytic recovery of H2 from H2S containing wastewater: surface and interface control of photo-excitons in Cu2S@TiO2 core–shell nanostructure. Appl. Catal. B Environ. 254, 174–185 (2019). https://doi.org/10.1016/j.apcatb.2019.04.090
P.S. Basavarajappa, B.N.H. Seethya, N. Ganganagappa, K.B. Eshwaraswamy, R.R. Kakarla, Enhanced photocatalytic activity and biosensing of gadolinium substituted BiFeO3 nanoparticles. ChemistrySelect 3, 9025–9033 (2018). https://doi.org/10.1002/slct.201801198
N.P. Shetti, S.J. Malode, D.S. Nayak, G.B. Bagihalli, S.S. Kalanur et al., Fabrication of ZnO nanoparticles modified sensor for electrochemical oxidation of methdilazine. Appl. Surf. Sci. 496, 143656 (2019). https://doi.org/10.1016/j.apsusc.2019.143656
S. Feng, L. Guanghua, Hydrothermal and solvothermal syntheses, in Modern Inorganic Synthetic Chemistry, 2nd edn., ed. by R. Xu, Y. Xu (Elsevier, Amsterdam, 2011), pp. 73–104. https://doi.org/10.1016/B978-0-444-63591-4.00004-5
G.W. Morey, Hydrothermal synthesis. J. Am. Ceram. Soc. 36, 279–285 (1953). https://doi.org/10.1111/j.1151-2916.1953.tb12883.x
D. Ohare, Hydrothermal Synthesis. Encyclopedia of Materials: Science and Technology, 2nd edn. (Elsevier, Amsterdam, 2001), pp. 3989–3992. https://doi.org/10.1016/B0-08-043152-6/00701-4
P. Nayak, M. Sahoo, S.K. Nayak, Urchin-like NiCo2O4 microsphere by hydrothermal route: structural, electrochemical, optical and magnetic properties. Ceram. Int. 46(3), 3818–3826 (2020). https://doi.org/10.1016/j.ceramint.2019.10.105
S. Debata, S. Patra, S. Banerjee, R. Madhuri, P.K. Sharma, Controlled hydrothermal synthesis of graphene supported NiCo2O4 coral-like nanostructures: an efficient electrocatalyst for overall water splitting. Appl. Surf. Sci. 449, 203–212 (2018). https://doi.org/10.1016/j.apsusc.2018.01.302
B. Cui, H. Lin, J.B. Li, X. Li, J. Yang, J. Tao, Core–ring structured NiCo2O4 nanoplatelets: synthesis, characterization, and electrocatalytic applications. Adv. Funct. Mater. 18, 1440–1447 (2008). https://doi.org/10.1002/adfm.200700982
K. Xu, J. Yang, J. Hu, Synthesis of hollow NiCo2O4 nanospheres with large specific surface area for asymmetric supercapacitors. J. Colloid Interface Sci. 511, 456–462 (2018). https://doi.org/10.1016/j.jcis.2017.09.113
X. Chang, W. Li, Y. Liu, M. He, X. Zheng, X. Lv, Z. Ren, Synthesis and characterization of NiCo2O4 nanospheres/nitrogen-doped graphene composites with enhanced electrochemical performance. J. Alloys Compd. 784, 293–300 (2019). https://doi.org/10.1016/j.jallcom.2019.01.036
Z.Q. Liu, Q.Z. Xu, J.Y. Wang, N. Li, S.H. Guo et al., Facile hydrothermal synthesis of urchin-like NiCo2O4 spheres as efficient electrocatalysts for oxygen reduction reaction. Int. J. Hydrogen Energy 38, 6657–6662 (2013). https://doi.org/10.1016/j.ijhydene.2013.03.092
R. Ding, L. Qi, M. Jia, H. Wang, Simple hydrothermal synthesis of mesoporous spinel NiCo2O4 nanoparticles and their catalytic behavior in CH3OH electro-oxidation and H2O2 electro-reduction. Catal. Sci. Technol. 3, 3207–3215 (2013). https://doi.org/10.1039/c3cy00590a
M. Yu, J. Chen, J. Liu, S. Li, Y. Ma, J. Zhang, J. An, Mesoporous NiCo2O4 nanoneedles grown on 3D graphene-nickel foam for supercapacitor and methanol electro-oxidation. Electrochim. Acta 151, 99–108 (2015). https://doi.org/10.1016/j.electacta.2014.10.156
Y. Liu, X. Chi, Q. Han, Y. Du, J. Yang, Y. Liu, Vertically self-standing C@ NiCo2O4 nanoneedle arrays as effective binder-free cathodes for rechargeable Na–O2 batteries. J. Alloys Compd. 772, 693–702 (2019). https://doi.org/10.1016/j.jallcom.2018.09.121
Y. Zhu, Z. Wu, M. Jing, W. Song, H. Hou, X. Yang, Q. Chen, X. Ji, 3D network-like mesoporous NiCo2O4 nanostructures as advanced electrode material for supercapacitors. Electrochim. Acta 149, 144–151 (2014). https://doi.org/10.1016/j.electacta.2014.10.064
J. Wang, Y. Zhang, J. Ye, H. Wei, J. Hao, J. Mu, S. Zhao, S. Hussain, Facile synthesis of three-dimensional NiCo2O4 with different morphology for supercapacitors. RSC Adv. 6, 70077–70084 (2016). https://doi.org/10.1039/c6ra14242g
Q. Zheng, X. Zhang, Y. Shen, Fabrication of free-standing NiCo2O4 nanoarrays via a facile modified hydrothermal synthesis method and their applications for lithium ion batteries and high-rate alkaline batteries. Mater. Res. Bull. 63, 211–215 (2015). https://doi.org/10.1016/j.materresbull.2014.12.024
W. Jiang, F. Hu, S. Yao, Z. Sun, X. Wu, Hierarchical NiCo2O4 nanowalls composed of ultrathin nanosheets as electrode materials for supercapacitor and Li ion battery applications. Mater. Res. Bull. 93, 303–309 (2017). https://doi.org/10.1016/j.materresbull.2017.05.036
X. Yang, X. Yu, Q. Yang, D. Zhao, K. Zhang et al., Controllable synthesis and magnetic properties of hydrothermally synthesized NiCo2O4 nano-spheres. Ceram. Int. 43, 8585–8589 (2017). https://doi.org/10.1016/j.ceramint.2017.03.121
S. Sun, X. Jin, B. Cong, X. Zhou, W. Hong, G. Chen, Construction of porous nanoscale NiO/NiCo2O4 heterostructure for highly enhanced electrocatalytic oxygen evolution activity. J. Catal. 379, 1–9 (2019). https://doi.org/10.1016/j.jcat.2019.09.010
E. Umeshbabu, G. Rajeshkhanna, G.R. Rao, Urchin and sheaf-like NiCo2O4 nanostructures: synthesis and electrochemical energy storage application. Int. J. Hydrogen Energy 39, 15627–15638 (2014). https://doi.org/10.1016/j.ijhydene.2014.07.168
W. Zhang, W. Xin, T. Hu, Q. Gong, T. Gao, G. Zhou, One-step synthesis of NiCo2O4 nanorods and firework-shaped microspheres formed with necklace-like structure for supercapacitor materials. Ceram. Int. 45, 8406–8413 (2019). https://doi.org/10.1016/j.ceramint.2019.01.149
J. Shen, X. Li, N. Li, M. Ye, Facile synthesis of NiCo2O4-reduced graphene oxide nanocomposites with improved electrochemical properties. Electrochim. Acta 141, 126–133 (2014). https://doi.org/10.1016/j.electacta.2014.07.063
A.K. Mondal, D. Su, S. Chen, X. Xie, G. Wang, Highly porous NiCo2O4 nanoflakes and nanobelts as anode materials for lithium-ion batteries with excellent rate capability. ACS Appl. Mater. Interfaces. 6, 14827–14835 (2014). https://doi.org/10.1021/am5036913
L. Shen, L. Yu, X.Y. Yu, X. Zhang, X.W.D. Lou, Self-templated formation of uniform NiCo2O4 hollow spheres with complex interior structures for lithium-Ion batteries and supercapacitors. Angew. Chem. Int. Ed. 54, 1868–1872 (2015). https://doi.org/10.1002/anie.201409776
H. Xin, Z. Xu, Y. Liu, W. Li, Z. Hu, 3D flower-like NiCo2O4 electrode material prepared by a modified solvothermal method for supercapacitor. J. Alloys Compd. 711, 670–676 (2017). https://doi.org/10.1016/j.jallcom.2017.03.208
J. Tang, A. Paul Alivisatos, Crystal splitting in the growth of Bi2S3. Nano Lett. 6, 2701–2706 (2006). https://doi.org/10.1021/nl0615930
G.R. Patzke, Y. Zhou, R. Kontic, F. Conrad, Oxide nanomaterials: synthetic developments, mechanistic studies, and technological innovations. Angew. Chem. Int. Ed. 50, 826–859 (2011). https://doi.org/10.1002/anie.201000235
S.K. Meher, P. Justin, G. Ranga-Rao, Nanoscale morphology dependent pseudocapacitance of NiO: influence of intercalating anions during synthesis. Nanoscale 3, 683–692 (2011). https://doi.org/10.1039/c0nr00555j
Z.Q. Liu, K. Xiao, Q.Z. Xu, N. Li, Y.Z. Su, H.J. Wang, S. Chen, Fabrication of hierarchical flower-like super-structures consisting of porous NiCo2O4 nanosheets and their electrochemical and magnetic properties. RSC Adv. 3, 4372–4380 (2013). https://doi.org/10.1039/c3ra23084h
A. Jain, B.J. Paul, S. Kim, V.K. Jain, J. Kim, A.K. Rai, Two-dimensional porous nanodisks of NiCo2O4 as anode material for high-performance rechargeable lithium-ion battery. J. Alloys Compd. 772, 72–79 (2019). https://doi.org/10.1016/j.jallcom.2018.09.051
Q. Wang, X. Wang, J. Xu, X. Ouyang, X. Hou, D. Chen, R. Wang, G. Shen, Flexible coaxial-type fiber supercapacitor based on NiCo2O4 nanosheets electrodes. Nano Energy 8, 44–51 (2014). https://doi.org/10.1016/j.nanoen.2014.05.014
Y. Chen, B. Qu, L. Hu, Z. Xu, Q. Li, T. Wang, High-performance supercapacitor and lithium-ion battery based on 3D hierarchical NH4F-induced nickel cobaltate nanosheet-nanowire cluster arrays as self-supported electrodes. Nanoscale 5, 9812–9820 (2013). https://doi.org/10.1039/c3nr02972g
D. Cai, S. Xiao, D. Wang, B. Liu, L. Wang et al., Morphology controlled synthesis of NiCo2O4 nanosheet array nanostructures on nickel foam and their application for pseudocapacitors. Electrochim. Acta 142, 118–124 (2014). https://doi.org/10.1016/j.electacta.2014.06.119
B. Cai, W. Mao, Z. Ye, J. Huang, Facile fabrication of all-solid-state SnO2/NiCo2O4 biosensor for self-powered glucose detection. Appl. Phys. A Mater. Sci. Process. 122, 806 (2016). https://doi.org/10.1007/s00339-016-0263-9
J. Deng, H. Zhang, Y. Zhang, P. Luo, L. Liu, Y. Wang, Striking hierarchical urchin-like peapoded NiCo2O4@C as advanced bifunctional electrocatalyst for overall water splitting. J. Power Sources 372, 46–53 (2017). https://doi.org/10.1016/j.jpowsour.2017.10.062
J. Yang, C. Li, Z. Quan, D. Kong, X. Zhang, P. Yang, J. Lin, One-step aqueous solvothermal synthesis of In2O3 nanocrystals. Cryst. Growth Des. 8, 695–699 (2008). https://doi.org/10.1021/cg070340x
S.M. Saleh, A.M. Soliman, M.A. Sharaf, V. Kale, B. Gadgil, Influence of solvent in the synthesis of nano-structured ZnO by hydrothermal method and their application in solar-still. J. Environ. Chem. Eng. B (2017). https://doi.org/10.1016/j.jece.2017.02.004
F. Fu, J. Li, Y. Yao, X. Qin, Y. Dou, H. Wang, J. Tsui, K.Y. Chan, M. Shao, Hierarchical NiCo2O4 micro- and nanostructures with tunable morphologies as anode materials for lithium- and sodium-ion batteries. ACS Appl. Mater. Interfaces 9, 16194–16201 (2017). https://doi.org/10.1021/acsami.7b02175
X. Wang, Y. Fang, B. Shi, F. Huang, F. Rong, R. Que, Three-dimensional NiCo2O4@ NiCo2O4 core–shell nanocones arrays for high-performance supercapacitors. Chem. Eng. J. 344, 311–319 (2018). https://doi.org/10.1016/j.cej.2018.03.061
Y. Liu, J. Goebl, Y. Yin, Templated synthesis of nanostructured materials. Chem. Soc. Rev. 42, 2610–2653 (2013). https://doi.org/10.1039/c2cs35369e
Q. Ren, G. Wu, W. Xing, J. Han, P. Li et al., Highly ordered mesoporous NiCo2O4 as a high performance anode material for Li-ion batteries. Front. Chem. 7, 521 (2019). https://doi.org/10.3389/fchem.2019.00521
C. Yuan, J. Li, L. Hou, J. Lin, G. Pang, L. Zhang, L. Lian, X. Zhang, Template-engaged synthesis of uniform mesoporous hollow NiCo2O4 sub-microspheres towards high-performance electrochemical capacitors. RSC Adv. 3, 18573–18578 (2013). https://doi.org/10.1039/c3ra42828a
W. Stöber, A. Fink, E. Bohn, Controlled growth of monodisperse silica spheres in the micron size range. J. Colloid Interface Sci. 26, 62–69 (1968). https://doi.org/10.1016/0021-9797(68)90272-5
V. Veeramani, R. Madhu, S.M. Chen, M. Sivakumar, Flower-like nickel–cobalt oxide decorated dopamine-derived carbon nanocomposite for high performance supercapacitor applications. ACS Sustain. Chem. Eng. 4, 5013–5020 (2016). https://doi.org/10.1021/acssuschemeng.6b01391
W. Xiong, Y. Gao, X. Wu, X. Hu, D. Lan et al., Composite of macroporous carbon with honeycomb-like structure from mollusc shell and NiCo2O4 nanowires for high-performance supercapacitor. ACS Appl. Mater. Interfaces 6, 19416–19423 (2014). https://doi.org/10.1021/am5055228
W. Li, F. Yang, Z. Hu, Y. Liu, Template synthesis of C@ NiCo2O4 hollow microsphere as electrode material for supercapacitor. J. Alloys Compd. 749, 305–312 (2018). https://doi.org/10.1016/j.jallcom.2018.03.046
X. Qi, W. Zheng, G. He, T. Tian, N. Du, L. Wang, NiCo2O4 hollow microspheres with tunable numbers and thickness of shell for supercapacitors. Chem. Eng. J. 309, 426–434 (2017). https://doi.org/10.1016/j.cej.2016.10.060
C. Li, X.Y. Gao, S.D. Li, Y. Zhao, K. Gao, Novel micron-sized NiCo2O4 pompon directed by a co-template method as capacitor electrode. Mater. Lett. 244, 74–77 (2019). https://doi.org/10.1016/j.matlet.2019.02.048
Y. Zhu, J. Wang, Z. Wu, M. Jing, H. Hou, X. Jia, X. Ji, An electrochemical exploration of hollow NiCo2O4 submicrospheres and its capacitive performances. J. Power Sources 287, 307–315 (2015). https://doi.org/10.1016/j.jpowsour.2015.04.053
P. Xu, R. Yu, H. Ren, L. Zong, J. Chen, X. Xing, Hierarchical nanoscale multi-shell Au/CeO2 hollow spheres. Chem. Sci. 5, 4221–4226 (2014). https://doi.org/10.1039/c4sc01882f
J. Wang, N. Yang, H. Tang, Z. Dong, Q. Jin et al., Accurate control of multishelled Co3O4 hollow microspheres as high-performance anode materials in lithium-ion batteries. Angew. Chem. Int. Ed. 52, 6417–6420 (2013). https://doi.org/10.1002/anie.201301622
X. Zeng, J. Yang, L. Shi, L. Li, M. Gao, Synthesis of multi-shelled ZnO hollow microspheres and their improved photocatalytic activity. Nanoscale Res. Lett. 9, 468 (2014). https://doi.org/10.1186/1556-276X-9-468
B. Wang, Y. Cao, Y. Chen, X. Lai, J. Peng, J. Tu, X. Li, Rapid synthesis of rGO conjugated hierarchical NiCo2O4 hollow mesoporous nanospheres with enhanced glucose sensitivity. Nanotechnology 28, 025501 (2017). https://doi.org/10.1088/0957-4484/28/2/025501
X. Lv, Y. Zhu, H. Jiang, X. Yang, Y. Liu, Y. Su, J. Huang, Y. Yao, C. Li, Hollow mesoporous NiCo2O4 nanocages as efficient electrocatalysts for oxygen evolution reaction. Dalton Trans. 44, 4148–4154 (2015). https://doi.org/10.1039/c4dt03803g
J. Nai, Y. Tian, X. Guan, L. Guo, Pearson’s principle inspired generalized strategy for the fabrication of metal hydroxide and oxide nanocages. J. Am. Chem. Soc. 135, 16082–16091 (2013). https://doi.org/10.1021/ja402751r
W. Huang, Y. Cao, Y. Chen, J. Peng, X. Lai, J. Tu, Fast synthesis of porous NiCo2O4 hollow nanospheres for a high-sensitivity non-enzymatic glucose sensor. Appl. Surf. Sci. 396, 804–811 (2017). https://doi.org/10.1016/j.apsusc.2016.11.034
X. Hou, S. Xue, M. Liu, X. Shang, Y. Fu, D. He, Hollow irregular octahedra-like NiCo2O4 cages composed of mesoporous nanosheets as a superior anode material for lithium-ion batteries. Chem. Eng. J. 350, 29–36 (2018). https://doi.org/10.1016/j.cej.2018.05.164
J. Yang, M. Cho, Y. Lee, Synthesis of hierarchical NiCo2O4 hollow nanorods via sacrificial-template accelerate hydrolysis for electrochemical glucose oxidation. Biosens. Bioelectron. 75, 15–22 (2016). https://doi.org/10.1016/j.bios.2015.08.008
H. Behzad, F.E. Ghodsi, PVP-assisted enhancement in ion storage performance of sol–gel derived nano-structured NiCo2O4 thin films as battery-type electrode. Mater. Res. Bull. 99, 336–342 (2018). https://doi.org/10.1016/j.materresbull.2017.11.031
M. El Baydi, S.K. Tiwari, R.N. Singh, J.L. Rehspringer, P. Chartier, J.F. Koenig, G. Poillerat, High specific surface area nickel mixed oxide powders LaNiO3 (Perovskite) and NiCo2O4 (Spinel) via sol–gel type routes for oxygen electrocatalysis in alkaline media. J. Solid State Chem. 116, 157–169 (1995). https://doi.org/10.1006/jssc.1995.1197
B. Hua, W. Zhang, J. Wu, J. Pu, B. Chi, L. Jian, A promising NiCo2O4 protective coating for metallic interconnects of solid oxide fuel cells. J. Power Sources 195, 7375–7379 (2010). https://doi.org/10.1016/j.jpowsour.2010.05.031
L. Merabet, K. Rida, N. Boukmouche, Sol–gel synthesis, characterization, and supercapacitor applications of MCo2O4 (M = Ni, Mn, Cu, Zn) cobaltite spinels. Ceram. Int. 44, 11265–11273 (2018). https://doi.org/10.1016/j.ceramint.2018.03.171
Y.Q. Wu, X.Y. Chen, P.T. Ji, Q.Q. Zhou, Sol–gel approach for controllable synthesis and electrochemical properties of NiCo2O4 crystals as electrode materials for application in supercapacitors. Electrochim. Acta 56, 7517–7522 (2011). https://doi.org/10.1016/j.electacta.2011.06.101
T.Y. Wei, C.H. Chen, H.C. Chien, S.Y. Lu, C.C. Hu, A cost-effective supercapacitor material of ultrahigh specific capacitances: spinel nickel cobaltite aerogels from an epoxide-driven sol–gel process. Adv. Mater. 22, 347–351 (2010). https://doi.org/10.1002/adma.200902175
M.C. Liu, C. Lu, X.M. Li, L. Bin Kong, Y.C. Luo, L. Kang, X. Li, F.C. Walsh, A sol–gel process for the synthesis of NiCo2O4 having improved specific capacitance and cycle stability for electrochemical capacitors. J. Electrochem. Soc. 159, A1262 (2012). https://doi.org/10.1149/2.057208jes
Y. Liu, N. Wang, C. Yang, W. Hu, Sol–gel synthesis of nanoporous NiCo2O4 thin films on ITO glass as high-performance supercapacitor electrodes. Ceram. Int. 42, 11411–11416 (2016). https://doi.org/10.1016/j.ceramint.2016.04.071
J. Bhagwan, G. Nagaraju, B. Ramulu, S.C. Sekhar, J.S. Yu, Rapid synthesis of hexagonal NiCo2O4 nanostructures for high-performance asymmetric supercapacitors. Electrochim. Acta 299, 509–517 (2019). https://doi.org/10.1016/j.electacta.2018.12.174
J. Liang, Z. Fan, S. Chen, S. Ding, G. Yang, Hierarchical NiCo2O4 nanosheets@halloysite nanotubes with ultrahigh capacitance and long cycle stability as electrochemical pseudocapacitor materials. Chem. Mater. 26, 4354–4360 (2014). https://doi.org/10.1021/cm500786a
F. Chen, W. Zhang, F. Cheng, Z. Yang, X. Fan, M. Huang, Stepwise co-precipitation to the synthesis of urchin-like NiCo2O4 hollow nanospheres as high performance anode material. J. Appl. Electrochem. 48, 1095–1104 (2018). https://doi.org/10.1007/s10800-018-1213-3
H.S. Jadhav, R.S. Kalubarme, C.N. Park, J. Kim, C.J. Park, Facile and cost effective synthesis of mesoporous spinel NiCo2O4 as an anode for high lithium storage capacity. Nanoscale 6, 10071–10076 (2014). https://doi.org/10.1039/c4nr02183e
L. Yu, B. Guan, W. Xiao, X.W. Lou, Formation of yolk-shelled Ni–Co mixed oxide nanoprisms with enhanced electrochemical performance for hybrid supercapacitors and lithium ion batteries. Adv. Energy Mater. 5, 1500981 (2015). https://doi.org/10.1002/aenm.201500981
C. Wang, X. Zhang, D. Zhang, C. Yao, Y. Ma, Facile and low-cost fabrication of nanostructured NiCo2O4 spinel with high specific capacitance and excellent cycle stability. Electrochim. Acta 63, 220–227 (2012). https://doi.org/10.1016/j.electacta.2011.12.090
H.S. Jadhav, R.S. Kalubarme, J.W. Roh, K.N. Jung, K.H. Shin, C.N. Park, C.J. Park, Facile and cost effective synthesized mesoporous spinel NiCo2O4 as catalyst for non-aqueous lithium-oxygen batteries. J. Electrochem. Soc. 161, A2188 (2014). https://doi.org/10.1149/2.0771414jes
H. Fu, Y. Liu, L. Chen, Y. Shi, W. Kong et al., Designed formation of NiCo2O4 with different morphologies self-assembled from nanoparticles for asymmetric supercapacitors and electrocatalysts for oxygen evolution reaction. Electrochim. Acta 296, 719–729 (2019). https://doi.org/10.1016/j.electacta.2018.11.103
Y. Wan, J. Chen, J. Zhan, Y. Ma, Facile synthesis of mesoporous NiCo2O4 fibers with enhanced photocatalytic performance for the degradation of methyl red under visible light irradiation. J. Environ. Chem. Eng. 6, 6079–6087 (2018). https://doi.org/10.1016/j.jece.2018.09.023
K.T. Jeng, C.C. Chien, N.Y. Hsu, S.C. Yen, S. Du Chiou, S.H. Lin, W.M. Huang, Performance of direct methanol fuel cell using carbon nanotube-supported Pt–Ru anode catalyst with controlled composition. J. Power Sources 160, 97–104 (2006). https://doi.org/10.1016/j.jpowsour.2006.01.057
B. Zhang, J. Zhu, H. Liu, P. Shi, W. Wu, F. Wang, Y. Liu, Rapid synthesis of hexagonal mesoporous structured NiCo2O4 via rotary evaporation for high performance supercapacitors. Ceram. Int. 44, 8695–8699 (2018). https://doi.org/10.1016/j.ceramint.2018.01.204
A. Karatutlu, A. Barhoum, A. Sapelkin, Liquid-phase synthesis of nanoparticles and nanostructured materials, in Emerging Applications of Nanoparticles and Architecture Nanostructures, ed. by A. Barhoum, A.S.H. Makhlouf (Elsevier, Amsterdam, 2018), pp. 1–28. https://doi.org/10.1016/B978-0-323-51254-1.00001-4
M.T. Crespo, A review of electrodeposition methods for the preparation of alpha-radiation sources. Appl. Radiat. Isot. 70, 210–215 (2012). https://doi.org/10.1016/j.apradiso.2011.09.010
M. Musiani, Electrodeposition of composites: an expanding subject in electrochemical materials science. Electrochim. Acta 45, 3397–3402 (2000). https://doi.org/10.1016/S0013-4686(00)00438-2
L.K. Wu, J. Xia, H.Z. Cao, Y.P. Tang, G.Y. Hou, G.Q. Zheng, Highly active and durable cauliflower-like NiCo2O4 film for oxygen evolution with electrodeposited SiO2 as template. Int. J. Hydrogen Energy 42, 10813–10825 (2017). https://doi.org/10.1016/j.ijhydene.2017.03.076
N. Wang, B. Sun, P. Zhao, M. Yao, W. Hu, S. Komarneni, Electrodeposition preparation of NiCo2O4 mesoporous film on ultrafine nickel wire for flexible asymmetric supercapacitors. Chem. Eng. J. 345, 31–38 (2018). https://doi.org/10.1016/j.cej.2018.03.147
N. Zhao, H. Fan, M. Zhang, J. Ma, W. Zhang et al., Investigating the large potential window of NiCo2O4 supercapacitors in neutral aqueous electrolyte. Electrochim. Acta 321, 134681 (2019). https://doi.org/10.1016/j.electacta.2019.134681
C. Yuan, J. Li, L. Hou, X. Zhang, L. Shen, X.W. Lou, Ultrathin mesoporous NiCo2O4 nanosheets supported on Ni foam as advanced electrodes for supercapacitors. Adv. Funct. Mater. 22, 4592–4597 (2012). https://doi.org/10.1002/adfm.201200994
A. Ramadoss, K.N. Kang, H.J. Ahn, S.I. Kim, S.T. Ryu, J.H. Jang, Realization of high performance flexible wire supercapacitors based on 3-dimensional NiCo2O4/Ni fibers. J. Mater. Chem. A 4, 4718–4727 (2016). https://doi.org/10.1039/c5ta10781d
J. Xiao, S. Yang, Sequential crystallization of sea urchin-like bimetallic (Ni, Co) carbonate hydroxide and its morphology conserved conversion to porous NiCo2O4 spinel for pseudocapacitors. RSC Adv. 1, 588–595 (2011). https://doi.org/10.1039/c1ra00342a
B. Ash, R.K. Paramguru, B.K. Mishra, Electrode reactions during electrolytic preparation of nickel hydroxide. Electrochem. Commun. 12, 48–51 (2010). https://doi.org/10.1016/j.elecom.2009.10.033
G. Zhang, T. Wang, X. Yu, H. Zhang, H. Duan, B. Lu, Nanoforest of hierarchical Co3O4@ NiCo2O4 nanowire arrays for high-performance supercapacitors. Nano Energy 2, 586–594 (2013). https://doi.org/10.1016/j.nanoen.2013.07.008
M. Mirzaee, C. Dehghanian, Synthesis of flower-like NiCo2O4 via chronopotentiometric technique and its application as electrode materials for high-performance supercapacitors. Mater. Today Energy 10, 68–80 (2018). https://doi.org/10.1016/j.mtener.2018.08.011
Y. An, Z. Hu, B. Guo, N. An, Y. Zhang, Z. Li, Y. Yang, H. Wu, Electrodeposition of honeycomb-shaped NiCo2O4 on carbon cloth as binder-free electrode for asymmetric electrochemical capacitor with high energy density. RSC Adv. 6, 37562–37573 (2016). https://doi.org/10.1039/c6ra04788b
J. Wu, P. Guo, R. Mi, X. Liu, H. Zhang et al., Ultrathin NiCo2O4 nanosheets grown on three-dimensional interwoven nitrogen-doped carbon nanotubes as binder-free electrodes for high-performance supercapacitors. J. Mater. Chem. A 3, 15331–15338 (2015). https://doi.org/10.1039/c5ta02719e
W.W. Liu, C.X. Lu, K. Liang, B.K. Tay, A high-performance anode material for Li-ion batteries based on a vertically aligned CNTs/NiCo2O4 core/shell structure. Part. Part. Syst. Charact. 31, 1151–1157 (2014). https://doi.org/10.1002/ppsc.201400030
L. Huang, D. Chen, Y. Ding, Z.L. Wang, Z. Zeng, M. Liu, Hybrid composite Ni(OH)2@ NiCo2O4 grown on carbon fiber paper for high-performance supercapacitors. ACS Appl. Mater. Interfaces 5, 11159–11162 (2013). https://doi.org/10.1021/am403367u
Y. Zhang, B. Wang, F. Liu, J. Cheng, X.W. Zhang, L. Zhang, Full synergistic contribution of electrodeposited three-dimensional NiCo2O4@MnO2 nanosheet networks electrode for asymmetric supercapacitors. Nano Energy 27, 627–637 (2016). https://doi.org/10.1016/j.nanoen.2016.08.013
J. Wang, R. Zhan, Y. Fu, H.Y. Yu, C. Jiang et al., Design and synthesis of hierarchical, freestanding bowl-like NiCo2O4 as cathode for long-life Li–O2 batteries. Mater. Today Energy 5, 214–221 (2017). https://doi.org/10.1016/j.mtener.2017.07.002
W. Wang, Z. Li, A. Meng, Q. Li, Network-like holey NiCo2O4 nanosheet arrays on Ni foam synthesized by electrodeposition for high-performance supercapacitors. J. Solid State Electrochem. 23, 635–644 (2019). https://doi.org/10.1007/s10008-018-4149-y
J. Zhang, R. Chu, Y. Chen, H. Jiang, Y. Zhang, N.M. Huang, H. Guo, Electrodeposited binder-free NiCo2O4@carbon nanofiber as a high performance anode for lithium ion batteries. Nanotechnology 29, 125401 (2018). https://doi.org/10.1088/1361-6528/aaa94c
P. Dinka, A. Mukasyan, Novel approaches to solution–combustion synthesis of nanomaterials. Int. J Self Propag. High Temp. Synth. 16, 23–35 (2007). https://doi.org/10.3103/S1061386207010049
K.V. Manukyan, Combustion and materials synthesis. Int. J. Self Propag. High Temp. Synth. 26, 143–144 (2017). https://doi.org/10.3103/S1061386217030025
J. Xu, Q. Chen, X. Liu, H. Baoquan, Q. Qian, The combustion synthesis of nanoscale ceria-doped calcia solid solution. Adv. Mater. Res. 610–613, 547–550 (2012). https://doi.org/10.4028/www.scientific.net/amr.610-613.547
R. Alcántara, M. Jaraba, P. Lavela, J.L. Tirado, NiCo2O4 spinel: first report on a transition metal oxide for the negative electrode of sodium-ion batteries. Chem. Mater. 14, 2847–2848 (2002). https://doi.org/10.1021/cm025556v
S.T. Aruna, A.S. Mukasyan, Combustion synthesis and nanomaterials. Curr. Opin. Solid State Mater. Sci. 12, 44–50 (2008). https://doi.org/10.1016/j.cossms.2008.12.002
A. Varma, A.S. Mukasyan, A.S. Rogachev, K.V. Manukyan, Solution combustion synthesis of nanoscale materials. Chem. Rev. 116, 14493–14586 (2016). https://doi.org/10.1021/acs.chemrev.6b00279
J.C. Toniolo, A.S. Takimi, C.P. Bergmann, Nanostructured cobalt oxides (Co3O4 and CoO) and metallic Co powders synthesized by the solution combustion method. Mater. Res. Bull. 45, 672–676 (2010). https://doi.org/10.1016/j.materresbull.2010.03.001
I.T. Papadas, A. Ioakeimidis, G.S. Armatas, S.A. Choulis, Low-temperature combustion synthesis of a spinel NiCo2O4 hole transport layer for perovskite photovoltaics. Adv. Sci. 5, 1701029 (2018). https://doi.org/10.1002/advs.201701029
T. Huang, B. Liu, P. Yang, Z. Qiu, Z. Hu, Facilely synthesized NiCo2O4 nanoparticles as electrode material for supercapacitors. Int. J. Electrochem. Sci. 13, 6144 (2018). https://doi.org/10.20964/2018.06.60
A.N. Naveen, S. Selladurai, Novel synthesis of highly porous spinel cobaltite (NiCo2O4) electrode material for supercapacitor applications. AIP Conf. Proc. 1591, 246 (2014). https://doi.org/10.1063/1.4872560
V. Shanmugavalli, K. Vishista, Low-cost synthesis of cubic spinel structured high efficient NiCo2O4/polyaniline nanocomposite for supercapacitor application. Mater. Res. Express 6, 45021 (2019). https://doi.org/10.1088/2053-1591/aae8ee
L. Li, S. Peng, Y. Cheah, P. Teh, J. Wang, G. Wee, Y. Ko, C. Wong, M. Srinivasan, Electrospun porous NiCo2O4 nanotubes as advanced electrodes for electrochemical capacitors. Chem. A: Eur. J. 19, 5892–5898 (2013). https://doi.org/10.1002/chem.201204153
H. Guan, C. Shao, Y. Liu, N. Yu, X. Yang, Fabrication of NiCo2O4 nanofibers by electrospinning. Solid State Commun. 131, 107–109 (2004). https://doi.org/10.1016/j.ssc.2004.04.035
G. Sun, L. Sun, H. Xie, J. Liu, Electrospinning of nanofibers for energy applications. Nanomaterials 6(7), E129 (2016). https://doi.org/10.3390/nano6070129
Q. Liu, J. Zhu, L. Zhang, Y. Qiu, Recent advances in energy materials by electrospinning. Renew. Sustain. Energy Rev. 81, 1825–1858 (2018). https://doi.org/10.1016/j.rser.2017.05.281
Z. Dong, S.J. Kennedy, Y. Wu, Electrospinning materials for energy-related applications and devices. J. Power Sources 196, 4886–4904 (2011). https://doi.org/10.1016/j.jpowsour.2011.01.090
F. Lai, Y.E. Miao, Y. Huang, T.S. Chung, T. Liu, Flexible hybrid membranes of NiCo2O4-doped carbon nanofiber@MnO2 core–sheath nanostructures for high-performance supercapacitors. J. Phys. Chem. C 119, 13442–13450 (2015). https://doi.org/10.1021/acs.jpcc.5b02739
C. Busacca, S.C. Zignani, A. Di Blasi, O. Di Blasi, M. Lo Faro, V. Antonucci, A.S. Aricò, Electrospun NiMn2O4 and NiCo2O4 spinel oxides supported on carbon nanofibers as electrocatalysts for the oxygen evolution reaction in an anion exchange membrane-based electrolysis cell. Int. J. Hydrogen Energy 44, 20987–20996 (2019). https://doi.org/10.1016/j.ijhydene.2019.02.214
K. Xu, S. Li, J. Yang, H. Xu, J. Hu, Hierarchical MnO2 nanosheets on electrospun NiCo2O4 nanotubes as electrode materials for high rate capability and excellent cycling stability supercapacitors. J. Alloys Compd. 678, 120–125 (2016). https://doi.org/10.1016/j.jallcom.2016.03.255
J. Sun, W. Wang, D. Yu, NiCo2O4 nanosheet-decorated carbon nanofiber electrodes with high electrochemical performance for flexible supercapacitors. J. Electron. Mater. 48, 3833–3843 (2019). https://doi.org/10.1007/s11664-019-07135-4
D. Lei, X.D. Li, M.K. Seo, M.S. Khil, H.Y. Kim, B.S. Kim, NiCo2O4 nanostructure-decorated PAN/lignin based carbon nanofiber electrodes with excellent cyclability for flexible hybrid supercapacitors. Polymer (Guildf) 132, 31–40 (2017). https://doi.org/10.1016/j.polymer.2017.10.051
G.M. Tomboc, H. Kim, Derivation of both EDLC and pseudocapacitance characteristics based on synergistic mixture of NiCo2O4 and hollow carbon nanofiber: an efficient electrode towards high energy density supercapacitor. Electrochim. Acta 318, 392–404 (2019). https://doi.org/10.1016/j.electacta.2019.06.112
A. Mirzaei, G. Neri, Microwave-assisted synthesis of metal oxide nanostructures for gas sensing application: a review. Sens. Actuators B: Chem. 237, 749–775 (2016). https://doi.org/10.1016/j.snb.2016.06.114
L.Y. Meng, B. Wang, M.G. Ma, K.L. Lin, The progress of microwave-assisted hydrothermal method in the synthesis of functional nanomaterials. Mater. Today Chem. 1–2, 63–83 (2016). https://doi.org/10.1016/j.mtchem.2016.11.003
X. Zhang, Y. Zhou, B. Luo, H. Zhu, W. Chu, K. Huang, Microwave-assisted synthesis of NiCo2O4 double-shelled hollow spheres for high-performance sodium ion batteries. Nano-Micro Lett. 10, 13 (2018). https://doi.org/10.1007/s40820-017-0164-2
A. Shanmugavani, R.K. Selvan, Microwave assisted reflux synthesis of NiCo2O4/NiO composite: fabrication of high performance asymmetric supercapacitor with Fe2O3. Electrochim. Acta 189, 283–294 (2016). https://doi.org/10.1016/j.electacta.2015.12.043
U.T. Nakate, S.N. Kale, Microwave assisted synthesis and characterizations of NiCo2O4 nanoplates and electrical, magnetic properties. Mater. Today: Proc. 3(6), 1992–1998 (2016). https://doi.org/10.1016/j.matpr.2016.04.101
L. Gu, L. Qian, Y. Lei, Y. Wang, J. Li, H. Yuan, D. Xiao, Microwave-assisted synthesis of nanosphere-like NiCo2O4 consisting of porous nanosheets and its application in electro-catalytic oxidation of methanol. J. Power Sources 261, 317–323 (2014). https://doi.org/10.1016/j.jpowsour.2014.03.098
Y.Z. Su, Q.Z. Xu, Q.S. Zhong, S.T. Shi, C.J. Zhang, C.W. Xu, NiCo2O4/C prepared by one-step intermittent microwave heating method for oxygen evolution reaction in splitter. J. Alloys Compd. 617, 115–119 (2014). https://doi.org/10.1016/j.jallcom.2014.07.195
Y. Tao, L. Ruiyi, L. Zaijun, F. Yinjun, A facile and scalable strategy for synthesis of size-tunable NiCo2O4 with nanocoral-like architecture for high-performance. Electrochim. Acta 134, 384–392 (2014). https://doi.org/10.1016/j.electacta.2014.03.168
Y. Zhu, X. Ji, R. Yin, Z. Hu, X. Qiu, Z. Wu, Y. Liu, Nanorod-assembled NiCo2O4 hollow microspheres assisted by an ionic liquid as advanced electrode materials for supercapacitors. RSC Adv. 7, 11123–11128 (2017). https://doi.org/10.1039/c7ra00067g
N.S. Chaubal, V.Y. Joshi, Nonionic polymeric surfactant template for mesoporous NiCo2O4 Formation. J. Porous Mater. 18, 177–183 (2011). https://doi.org/10.1007/s10934-010-9368-2
T.V. Gavrilović, D.J. Jovanović, M.D. Dramićanin, Chapter 2—synthesis of multifunctional inorganic materials: from micrometer to nanometer dimensions. Nanomater. Green Energy 1, 55–81 (2018). https://doi.org/10.1016/B978-0-12-813731-4.00002-3
M. Kundu, G. Karunakaran, E. Kolesnikov, V.E. Sergeevna, S. Kumari, M.V. Gorshenkov, D. Kuznetsov, Hollow NiCo2O4 nano-spheres obtained by ultrasonic spray pyrolysis method with superior electrochemical performance for lithium-ion batteries and supercapacitors. J. Ind. Eng. Chem. 59, 90–98 (2018). https://doi.org/10.1016/j.jiec.2017.10.010
Y. Ma, Z. Yu, M. Liu, C. Song, X. Huang, M. Moliere, G. Song, H. Liao, Deposition of binder-free oxygen-vacancies NiCo2O4 based films with hollow microspheres via solution precursor thermal spray for supercapacitors. Ceram. Int. 45, 10722–10732 (2019). https://doi.org/10.1016/j.ceramint.2019.02.145
T. Li, X. Li, Z. Wang, H. Guo, Y. Li, A novel NiCo2O4 anode morphology for lithium-ion batteries. J. Mater. Chem. A 3, 11970–11975 (2015). https://doi.org/10.1039/c5ta01928a
J. Leng, Z. Wang, X. Li, H. Guo, T. Li, H. Liang, Self-templated formation of hierarchical NiCo2O4 yolk–shell microspheres with enhanced electrochemical properties. Electrochim. Acta 244, 154–161 (2017). https://doi.org/10.1016/j.electacta.2017.05.109
D.P. Lapham, J.L. Lapham, The porosity of NiCo2O4 films and powders by three common preparation techniques. Microporous Mesoporous Mater. 223, 35–45 (2016). https://doi.org/10.1016/j.micromeso.2015.10.025
R.J. Deokate, R.S. Kalubarme, C.J. Park, C.D. Lokhande, Simple synthesis of NiCo2O4 thin films using spray pyrolysis for electrochemical supercapacitor application: a novel approach. Electrochim. Acta 224, 378–385 (2017). https://doi.org/10.1016/j.electacta.2016.12.034
G.D. Park, J.K. Lee, Y.C. Kang, Three-dimensional macroporous CNTs microspheres highly loaded with NiCo2O4 hollow nanospheres showing excellent lithium-ion storage performances. Carbon 128, 191–200 (2018). https://doi.org/10.1016/j.carbon.2017.11.088
R.A. Soomro, Z.H. Ibupoto, Sirajuddin, M.I. Abro, M. Willander, Electrochemical sensing of glucose based on novel hedgehog-like NiO nanostructures. Sens. Actuators B: Chem. 209, 966–974 (2015). https://doi.org/10.1016/j.snb.2014.12.050
E. Zhang, Y. Xie, S. Ci, J. Jia, Z. Wen, Porous Co3O4 hollow nanododecahedra for nonenzymatic glucose biosensor and biofuel cell. Biosens. Bioelectron. 81, 46–53 (2016). https://doi.org/10.1016/j.bios.2016.02.027
K.K. Naik, S. Kumar, C.S. Rout, Electrodeposited spinel NiCo2O4 nanosheet arrays for glucose sensing application. RSC Adv. 5, 74585–74591 (2015). https://doi.org/10.1039/c5ra13833g
M. Hussain, Z.H. Ibupoto, M.A. Abbasi, X. Liu, O. Nur, M. Willander, Synthesis of three dimensional nickel cobalt oxide nanoneedles on nickel foam, their characterization and glucose sensing application. Sensors 14, 5415–5425 (2014). https://doi.org/10.3390/s140305415
R. Elakkiya, G. Maduraiveeran, A three-dimensional nickel–cobalt oxide nanomaterial as an enzyme-mimetic electrocatalyst for the glucose and lactic acid oxidation reaction. New J. Chem. 43, 14756–14762 (2019). https://doi.org/10.1039/c9nj01291e
R. Ding, L. Qi, M. Jia, H. Wang, Facile synthesis of mesoporous spinel NiCo2O4 nanostructures as highly efficient electrocatalysts for urea electro-oxidation. Nanoscale 6, 1369–1376 (2014). https://doi.org/10.1039/c3nr05359h
Y. Feng, D. Xiang, Y. Qiu, L. Li, Y. Li, K. Wu, L. Zhu, MOF-derived spinel NiCo2O4 hollow nanocages for the construction of non-enzymatic electrochemical glucose sensor. Electroanalysis 32(3), 571–580 (2020). https://doi.org/10.1002/elan.201900558
S. Wang, S. Zhang, M. Liu, H. Song, J. Gao, Y. Qian, MoS2 as connector inspired high electrocatalytic performance of NiCo2O4 nanoplates towards glucose. Sens. Actuators B: Chem. 254, 1101–1109 (2018). https://doi.org/10.1016/j.snb.2017.08.011
H. Zhao, J. Xu, Q. Sheng, J. Zheng, W. Cao, T. Yue, NiCo2O4 nanorods decorated MoS2 nanosheets synthesized from deep eutectic solvents and their application for electrochemical sensing of glucose in red wine and honey. J. Electrochem. Soc. 166, H404 (2019). https://doi.org/10.1149/2.0141910jes
A.P. Abbott, G. Capper, D.L. Davies, R.K. Rasheed, V. Tambyrajah, Novel solvent properties of choline chloride/urea mixtures. Chem. Commun. 9, 70–71 (2003). https://doi.org/10.1039/b210714g
M. Avalos, R. Babiano, P. Cintas, J.L. Jiménez, J.C. Palacios, Greener media in chemical synthesis and processing. Angew. Chem. Int. Ed. 45, 3904–3908 (2006). https://doi.org/10.1002/anie.200504285
A.P. Abbott, K. El Ttaib, G. Frisch, K.J. McKenzie, K.S. Ryder, Electrodeposition of copper composites from deep eutectic solvents based on choline chloride. Phys. Chem. Chem. Phys. 11, 4269–4277 (2009). https://doi.org/10.1039/b817881j
F.H. Aidoudi, P.J. Byrne, P.K. Allan, S.J. Teat, P. Lightfoot, R.E. Morris, Ionic liquids and deep eutectic mixtures as new solvents for the synthesis of vanadium fluorides and oxyfluorides. Dalton Trans. 40, 4324–4331 (2011). https://doi.org/10.1039/c0dt01765e
L. Wei, Z.Y. Zhou, S.P. Chen, C.D. Xu, D. Su, M.E. Schuster, S.G. Sun, Electrochemically shape-controlled synthesis in deep eutectic solvents: triambic icosahedral platinum nanocrystals with high-index facets and their enhanced catalytic activity. Chem. Commun. 49, 11152–11154 (2013). https://doi.org/10.1039/c3cc46473c
L. Wei, Y.J. Fan, N. Tian, Z.Y. Zhou, X.Q. Zhao, B.W. Mao, S.G. Sun, Electrochemically shape-controlled synthesis in deep eutectic solvents—a new route to prepare Pt nanocrystals enclosed by high-index facets with high catalytic activity. J. Phys. Chem. C 116, 2040–2044 (2012). https://doi.org/10.1021/jp209743h
S. Cui, J. Zhang, Y. Ding, S. Gu, P. Hu, Z. Hu, Rectangular flake-like mesoporous NiCo2O4 as enzyme mimic for glucose biosensing and biofuel cell. Sci. China Mater. 60, 766–776 (2017). https://doi.org/10.1007/s40843-017-9072-9
F.C. Sun, J.T. Zhang, H. Ren, S.T. Wang, Y. Zhou, J. Zhang, “Dry” NiCo2O4 nanorods for electrochemical non-enzymatic glucose sensing. Chin. J. Chem. Phys. 31, 799 (2018). https://doi.org/10.1063/1674-0068/31/cjcp1804061
Q. Guo, W. Zeng, Y. Li, Highly sensitive non-enzymatic glucose sensor based on porous NiCo2O4 nanowires grown on nickel foam. Mater. Lett. 256, 126603 (2019). https://doi.org/10.1016/j.matlet.2019.126603
P. Arul, S.A. John, Organic solvent free in situ growth of flower like Co-ZIF microstructures on nickel foam for glucose sensing and supercapacitor applications. Electrochim. Acta 306, 254–263 (2019). https://doi.org/10.1016/j.electacta.2019.03.117
X. Luo, M. Huang, D. He, M. Wang, Y. Zhang, P. Jiang, Porous NiCo2O4 nanoarray-integrated binder-free 3D open electrode offers a highly efficient sensing platform for enzyme-free glucose detection. Analyst 143, 2546–2554 (2018). https://doi.org/10.1039/c8an00668g
D. Zhang, J. Tong, B. Xia, Q. Xue, Ultrahigh performance humidity sensor based on layer-by-layer self-assembly of graphene oxide/polyelectrolyte nanocomposite film. Sens. Actuators B: Chem. 203, 263–270 (2014). https://doi.org/10.1016/j.snb.2014.06.116
D. Zhang, J. Tong, B. Xia, Humidity-sensing properties of chemically reduced graphene oxide/polymer nanocomposite film sensor based on layer-by-layer nano self-assembly. Sens. Actuators B: Chem. 197, 66–72 (2014). https://doi.org/10.1016/j.snb.2014.02.078
T.H. Ko, S. Radhakrishnan, M.K. Seo, M.S. Khil, H.Y. Kim, B.S. Kim, A green and scalable dry synthesis of NiCo2O4/graphene nanohybrids for high-performance supercapacitor and enzymeless glucose biosensor applications. J. Alloys Compd. 696, 193–200 (2017). https://doi.org/10.1016/j.jallcom.2016.11.234
M. Wu, S. Meng, Q. Wang, W. Si, W. Huang, X. Dong, Nickel–cobalt oxide decorated three-dimensional graphene as an enzyme mimic for glucose and calcium detection. ACS Appl. Mater. Interfaces 7, 21089–21094 (2015). https://doi.org/10.1021/acsami.5b06299
G. Ma, M. Yang, C. Li, H. Tan, L. Deng, S. Xie, F. Xu, L. Wang, Y. Song, Preparation of spinel nickel–cobalt oxide nanowrinkles/reduced graphene oxide hybrid for nonenzymatic glucose detection at physiological level. Electrochim. Acta 220, 545–553 (2016). https://doi.org/10.1016/j.electacta.2016.10.163
Y. Ni, J. Xu, H. Liu, S. Shao, Fabrication of RGO-NiCo2O4 nanorods composite from deep eutectic solvents for nonenzymatic amperometric sensing of glucose. Talanta 185, 335–343 (2018). https://doi.org/10.1016/j.talanta.2018.03.097
F. Meng, J. Li, S.K. Cushing, M. Zhi, N. Wu, Solar hydrogen generation by nanoscale p–n junction of p-type molybdenum disulfide/n-type nitrogen-doped reduced graphene oxide. J. Am. Chem. Soc. 135, 10286–10289 (2013). https://doi.org/10.1021/ja404851s
Y. Liu, K. Yan, O.K. Okoth, J. Zhang, A label-free photoelectrochemical aptasensor based on nitrogen-doped graphene quantum dots for chloramphenicol determination. Biosens. Bioelectron. 74, 1016–1021 (2015). https://doi.org/10.1016/j.bios.2015.07.067
L. Feng, N. Xie, J. Zhong, Carbon nanofibers and their composites: a review of synthesizing, properties and applications. Materials 7, 3919–3945 (2014). https://doi.org/10.3390/ma7053919
W. Lu, T. He, B. Xu, X. He, H. Adidharma, M. Radosz, K. Gasem, M. Fan, Progress in catalytic synthesis of advanced carbon nanofibers. J. Mater. Chem. A 5, 13863–13881 (2017). https://doi.org/10.1039/c7ta02007d
D.W. Kim, C.H. Kim, C.M. Yang, S. Ahn, Y.H. Kim et al., Deriving structural perfection in the structure of polyacrylonitrile based electrospun carbon nanofiber. Carbon 147, 612–615 (2019). https://doi.org/10.1016/j.carbon.2019.02.066
M. Naebe, T. Lin, X. Wang, Carbon nanotubes reinforced electrospun polymer nanoibres, in Nanofibres, ed. by A. Kumar (Intech, Croatia, 2010), pp. 309–328. https://doi.org/10.5772/8160
T. Fujita, Hierarchical nanoporous metals as a path toward the ultimate three-dimensional functionality. Sci. Technol. Adv. Mater. 18, 724–740 (2017). https://doi.org/10.1080/14686996.2017.1377047
T. Fujita, L.H. Qian, K. Inoke, J. Erlebacher, M.W. Chen, Three-dimensional morphology of nanoporous gold. Appl. Phys. Lett. 92, 251902 (2008). https://doi.org/10.1063/1.2948902
X.Y. Lang, H.Y. Fu, C. Hou, G.F. Han, P. Yang, Y.B. Liu, Q. Jiang, Nanoporous gold supported cobalt oxide microelectrodes as high-performance electrochemical biosensors. Nat. Commun. 4, 2169 (2013). https://doi.org/10.1038/ncomms3169
W. Li, H. Qi, B. Wang, Q. Wang, S. Wei, X. Zhang, Y. Wang, L. Zhang, X. Cui, Ultrathin NiCo2O4 nanowalls supported on a 3D nanoporous gold coated needle for non-enzymatic amperometric sensing of glucose. Microchim. Acta 185, 124 (2018). https://doi.org/10.1007/s00604-017-2663-8
K.K. Naik, A. Gangan, B. Chakraborty, C.S. Rout, Superior non-enzymatic glucose sensing properties of Ag–/Au–NiCo2O4 nanosheets with insight from electronic structure simulations. Analyst 143, 571–579 (2018). https://doi.org/10.1039/c7an01354j
K.K. Naik, A. Gangan, B. Chakraborty, S.K. Nayak, C.S. Rout, Enhanced nonenzymatic glucose-sensing properties of electrodeposited NiCo2O4–Pd nanosheets: experimental and DFT investigations. ACS Appl. Mater. Interfaces 9, 23894–23903 (2017). https://doi.org/10.1021/acsami.7b02217
G. Cakmak, Z. Kü̧ükyavuz, S. Kü̧ükyavuz, Conductive copolymers of polyaniline, polypyrrole and poly(dimethylsiloxane). Synth. Met. 151, 10–18 (2005). https://doi.org/10.1016/j.synthmet.2005.02.019
A.S. Hammad, H. Noby, M.F. Elkady, A.H. El-Shazly, In-situ polymerization of polyaniline/polypyrrole copolymer using different techniques, in IOP Conference Series: Materials Science and Engineering, vol. 290 (2018), p. 012001. https://doi.org/10.1088/1757-899X/290/1/012001
J.M. Jeong, B.G. Choi, S.C. Lee, K.G. Lee, S.J. Chang et al., Hierarchical hollow spheres of Fe2O3@polyaniline for lithium ion battery anodes. Adv. Mater. 25, 6250–6255 (2013). https://doi.org/10.1002/adma.201302710
Z. Yu, H. Li, X. Zhang, N. Liu, W. Tan, X. Zhang, L. Zhang, Facile synthesis of NiCo2O4@Polyaniline core–shell nanocomposite for sensitive determination of glucose. Biosens. Bioelectron. 75, 161–165 (2016). https://doi.org/10.1016/j.bios.2015.08.024
X. Duan, K. Liu, Y. Xu, M. Yuan, T. Gao, J. Wang, Nonenzymatic electrochemical glucose biosensor constructed by NiCo2O4@Ppy nanowires on nickel foam substrate. Sens. Actuators B: Chem. 292, 121–128 (2019). https://doi.org/10.1016/j.snb.2019.04.107
A.B. Vennela, D. Mangalaraj, N. Muthukumarasamy, S. Agilan, K.V. Hemalatha, Structural and optical properties of Co3O4 nanoparticles prepared by sol–gel technique for photocatalytic application. Int. J. Electrochem. Sci. 14, 3535–3552 (2019). https://doi.org/10.20964/2019.04.40
O. Mounkachi, E. Salmani, M. Lakhal, H. Ez-Zahraouy, M. Hamedoun, M. Benaissa, A. Kara, A. Ennaoui, A. Benyoussef, Band-gap engineering of SnO2. Sol. Energy Mater. Sol. Cells 148, 34–38 (2016). https://doi.org/10.1016/j.solmat.2015.09.062
E. Cockayne, L. Li, First-principles DFT + U studies of the atomic, electronic, and magnetic structure of α-MnO2 (cryptomelane). Chem. Phys. Lett. 544, 53–58 (2012). https://doi.org/10.1016/j.cplett.2012.06.061
M.C. Liu, L. Bin Kong, C. Lu, X.M. Li, Y.C. Luo, L. Kang, A sol–gel process for fabrication of NiO/NiCo2O4/Co3O4 composite with improved electrochemical behavior for electrochemical capacitors. ACS Appl. Mater. Interfaces 4, 4631–4636 (2012). https://doi.org/10.1021/am301010u
D. Chen, D. Pang, S. Zhang, H. Song, W. Zhu, J. Zhu, Synergistic coupling of NiCo2O4 nanorods onto porous Co3O4 nanosheet surface for tri-functional glucose, hydrogen-peroxide sensors and supercapacitor. Electrochim. Acta 330, 135326 (2020). https://doi.org/10.1016/j.electacta.2019.135326
B. Xue, K. Li, L. Feng, J. Lu, L. Zhang, Graphene wrapped porous Co3O4/NiCo2O4 double-shelled nanocages with enhanced electrocatalytic performance for glucose sensor. Electrochim. Acta 239, 36–44 (2017). https://doi.org/10.1016/j.electacta.2017.04.005
D. Chen, S. Wang, M. Liu, J. Gao, H. Song, S. Zhang, Bionics-inspired strong coupling of streptococcus-like NiCo2O4 to needle-like MnO2 for enhanced electrocatalytic determination of glucose. J. Electrochem. Soc. 166, B1653 (2019). https://doi.org/10.1149/2.1291915jes
Y. Su, B. Luo, J.Z. Zhang, Controllable cobalt oxide/Au hierarchically nanostructured electrode for nonenzymatic glucose sensing. Anal. Chem. 88, 1617–1624 (2016). https://doi.org/10.1021/acs.analchem.5b03396
Z. Qin, Q. Cheng, Y. Lu, J. Li, Facile synthesis of hierarchically mesoporous NiCo2O4 nanowires for sensitive nonenzymatic glucose detection. Appl. Phys. A Mater. Sci. Process. 123, 492 (2017). https://doi.org/10.1007/s00339-017-1108-x
W. Huang, T. Lin, Y. Cao, X. Lai, J. Peng, J. Tu, Hierarchical NiCo2O4 hollow sphere as a peroxidase mimetic for colorimetric detection of H2O2 and glucose. Sensors 17, 217 (2017). https://doi.org/10.3390/s17010217
L. Su, W. Dong, C. Wu, Y. Gong, Y. Zhang, L. Li, G. Mao, S. Feng, The peroxidase and oxidase-like activity of NiCo2O4 mesoporous spheres: mechanistic understanding and colorimetric biosensing. Anal. Chim. Acta 951, 124–132 (2017). https://doi.org/10.1016/j.aca.2016.11.035
M. Saraf, K. Natarajan, S.M. Mobin, Multifunctional porous NiCo2O4 nanorods: sensitive enzymeless glucose detection and supercapacitor properties with impedance spectroscopic investigations. New J. Chem. 41, 9299–9313 (2017). https://doi.org/10.1039/c7nj01519d
H. Yin, T. Zhan, D. Qin, X. He, Q. Nie, J. Gong, Self-assembly of dandelion-like NiCo2O4 hierarchical microspheres for non-enzymatic glucose sensor. Inorg. Nano Met. Chem. 47, 1560–1567 (2017). https://doi.org/10.1080/24701556.2017.1357610
W. Chen, S. Cai, Q.Q. Ren, W. Wen, Y. Di Zhao, Recent advances in electrochemical sensing for hydrogen peroxide: a review. Analyst 137, 49–58 (2012). https://doi.org/10.1039/c1an15738h
K. Dhara, D.R. Mahapatra, Recent advances in electrochemical nonenzymatic hydrogen peroxide sensors based on nanomaterials: a review. J. Mater. Sci. 54, 12319–12357 (2019). https://doi.org/10.1007/s10853-019-03750-y
R. Zhang, W. Chen, Recent advances in graphene-based nanomaterials for fabricating electrochemical hydrogen peroxide sensors. Biosens. Bioelectron. 89, 249–268 (2017). https://doi.org/10.1016/j.bios.2016.01.080
P. Kannan, T. Maiyalagan, A. Pandikumar, L. Guo, P. Veerakumar, P. Rameshkumar, Highly sensitive enzyme-free amperometric sensing of hydrogen peroxide in real samples based on Co3O4 nanocolumn structures. Anal. Methods 11, 2292–2302 (2019). https://doi.org/10.1039/c9ay00230h
R. Ding, L. Qi, M. Jia, H. Wang, Porous NiCo2O4 nanostructures as bi-functional electrocatalysts for CH3OH oxidation reaction and H2O2 reduction reaction. Electrochim. Acta 113, 290–301 (2013). https://doi.org/10.1016/j.electacta.2013.09.053
Y. Wang, K. Cheng, D. Cao, F. Yang, P. Yan, W. Zhang, G. Wang, Preparation of NiCo2O4 nanosheet arrays and its high catalytic performance for H2O2 electroreduction. Fuel Cells 15, 298–305 (2015). https://doi.org/10.1002/fuce.201300299
L. Ning, X. Guan, J. Ma, M. Wang, X. Fan, G. Zhang, F. Zhang, W. Peng, Y. Li, A highly sensitive nonenzymatic H2O2 sensor based on platinum, ZnFe2O4 functionalized reduced graphene oxide. J. Alloys Compd. 738, 317–322 (2018). https://doi.org/10.1016/j.jallcom.2017.12.161
X. Xiong, C. You, X. Cao, L. Pang, R. Kong, X. Sun, Ni2P nanosheets array as a novel electrochemical catalyst electrode for non-enzymatic H2O2 sensing. Electrochim. Acta 253, 517–521 (2017). https://doi.org/10.1016/j.electacta.2017.09.104
F. Xie, X. Cao, F. Qu, A.M. Asiri, X. Sun, Cobalt nitride nanowire array as an efficient electrochemical sensor for glucose and H2O2 detection. Sens. Actuators B: Chem. 255, 1254–1261 (2018). https://doi.org/10.1016/j.snb.2017.08.098
Y. Li, M. Zhang, X. Zhang, G. Xie, Z. Su, G. Wei, Nanoporous carbon nanofibers decorated with platinum nanoparticles for non-enzymatic electrochemical sensing of H2O2. Nanomaterials 5, 1891–1905 (2015). https://doi.org/10.3390/nano5041891
L. Tian, K. Xia, W. Hu, X. Zhong, Y. Chen et al., A wide linear range and stable H2O2 electrochemical sensor based on Ag decorated hierarchical Sn3O4. Electrochim. Acta 231, 190–199 (2017). https://doi.org/10.1016/j.electacta.2017.02.052
X. Xiao, F. Yang, K. Cheng, X. Wang, J. Yin, K. Ye, G. Wang, D. Cao, NiCo2O4 nanostructures with various morphologies as the high-performance electrocatalysts for H2O2 electroreduction and electrooxidation. J. Electroanal. Chem. 729, 103–108 (2014). https://doi.org/10.1016/j.jelechem.2014.07.010
B. Xue, K. Li, S. Gu, L. Zhang, J. Lu, Ni foam-supported ZnO nanowires and Co3O4/NiCo2O4 double-shelled nanocages for efficient hydrogen peroxide detection. Sens. Actuators B: Chem. 262, 828–836 (2018). https://doi.org/10.1016/j.snb.2018.02.091
M. Sakthivel, R. Sukanya, S.M. Chen, K. Pandi, K.C. Ho, Synthesis and characterization of bimetallic nickel–cobalt chalcogenides (NiCoSe2, NiCo2S4, and NiCo2O4) for non-enzymatic hydrogen peroxide sensor and energy storage: electrochemical properties dependence on the metal-to-chalcogen composition. Renew. Energy 138, 139–151 (2019). https://doi.org/10.1016/j.renene.2019.01.079
R.L. King, G.G. Botte, Hydrogen production via urea electrolysis using a gel electrolyte. J. Power Sources 196 (5), 2773–2778 (2011). https://doi.org/10.1016/j.jpowsour.2010.11.006
W.M. Omymen, J.R. Rogan, B.Z. Jugović, M.M. Gvozdenović, B.N. Grgur, Photo-assisted electrochemical oxidation of the urea onto TiO2-nanotubes modified by hematite. J. Saudi Chem. Soc. 21(8), 990–997 (2017). https://doi.org/10.1016/j.jscs.2017.05.010
J. Kim, W.J.K. Choi, J. Choi, M.R. Hoffmann, H. Park, Electrolysis of urea and urine for solar hydrogen. Catal. Today 199, 2–7 (2013). https://doi.org/10.1016/j.cattod.2012.02.009
B.K. Boggs, R.L. King, G.G. Botte, Urea electrolysis: direct hydrogen production from urine. Chem. Commun. 32, 4859–4861 (2009). https://doi.org/10.1039/b905974a
A.A. Ibrahim, R. Ahmad, A. Umar, M.S. Al-Assiri, A.E. Al-Salami, R. Kumar, S.G. Ansari, S. Baskoutas, Two-dimensional ytterbium oxide nanodisks based biosensor for selective detection of urea. Biosens. Bioelectron. 98, 254–260 (2017). https://doi.org/10.1016/j.bios.2017.06.015
G. Dhawan, G. Sumana, B.D. Malhotra, Recent developments in urea biosensors. Biochem. Eng. J. 44, 42–52 (2009). https://doi.org/10.1016/j.bej.2008.07.004
S. Amin, A. Tahira, A. Solangi, V. Beni, J.R. Morante et al., A practical non-enzymatic urea sensor based on NiCo2O4 nanoneedles. RSC Adv. 9, 14443–14451 (2019). https://doi.org/10.1039/c9ra00909d
N. Senthilkumar, G. Gnana kumar, A. Manthiram, 3D hierarchical core–shell nanostructured arrays on carbon fibers as catalysts for direct urea fuel cells. Adv. Energy Mater. 8(6), 1702207 (2018). https://doi.org/10.1002/aenm.201702207
N.S. Nguyen, G. Das, H.H. Yoon, Nickel/cobalt oxide-decorated 3D graphene nanocomposite electrode for enhanced electrochemical detection of urea. Biosens. Bioelectron. 77, 372–377 (2016). https://doi.org/10.1016/j.bios.2015.09.046
H.J. Choi, S.M. Jung, J.M. Seo, D.W. Chang, L. Dai, J.B. Baek, Graphene for energy conversion and storage in fuel cells and supercapacitors. Nano Energy 1, 534–551 (2012). https://doi.org/10.1016/j.nanoen.2012.05.001
V. Vedharathinam, G.G. Botte, Understanding the electro-catalytic oxidation mechanism of urea on nickel electrodes in alkaline medium. Electrochim. Acta 81, 292–300 (2012). https://doi.org/10.1016/j.electacta.2012.07.007
H.N. Siti, J. Jalil, A.Y. Asmadi, Y. Kamisah, Roles of rutin in cardiac remodeling. J. Funct. Foods 64, 103606 (2020). https://doi.org/10.1016/j.jff.2019.103606
M.L. Yola, C. Göde, N. Atar, Determination of rutin by CoFe2O4 nanoparticles ionic liquid nanocomposite as a voltammetric sensor. J. Mol. Liq. 246, 350–353 (2017). https://doi.org/10.1016/j.molliq.2017.09.072
S. Cui, L. Li, Y. Ding, J. Zhang, H. Yang, Y. Wang, Mesoporous NiCo2O4-decorated reduced graphene oxide as a novel platform for electrochemical determination of rutin. Talanta 164, 291–299 (2017). https://doi.org/10.1016/j.talanta.2016.10.109
K.H.G. Freitas, O. Fatibello-Filho, I.L. de Mattos, Square-wave voltammetric determination of rutin in pharmaceutical formulations using a carbon composite electrode modified with copper (II) phosphate immobilized in polyester resin. Braz J. Pharm. Sci. 48, 2175–9790 (2012). https://doi.org/10.1590/S1984-82502012000400007
J. Kang, X. Lu, H. Zeng, H. Liu, B. Lu, Investigation on the electrochemistry of rutin and its analytical application. Anal. Lett. 35, 677–686 (2002). https://doi.org/10.1081/AL-120003169
P. Miao, T. Liu, X. Li, L. Ning, J. Yin, K. Han, Highly sensitive, label-free colorimetric assay of trypsin using silver nanoparticles. Biosens. Bioelectron. 49, 20–24 (2013). https://doi.org/10.1016/j.bios.2013.04.038
X. Lin, Z. Zhu, C. Zhao, S. Li, Q. Liu, A. Liu, L. Lin, X. Lin, Robust oxidase mimicking activity of protamine-stabilized platinum nanoparticles units and applied for colorimetric sensor of trypsin and inhibitor. Sens. Actuators B: Chem. 284, 346–353 (2019). https://doi.org/10.1016/j.snb.2018.12.109
Y. Lin, R. Shen, N. Liu, H. Yi, H. Dai, J. Lin, A highly sensitive peptide-based biosensor using NiCo2O4 nanosheets and g-C3N4 nanocomposite to construct amplified strategy for trypsin detection. Anal. Chim. Acta 1035, 175–183 (2018). https://doi.org/10.1016/j.aca.2018.06.040
B. Kaur, B. Satpati, R. Srivastava, Synthesis of NiCo2O4/Nano-ZSM-5 nanocomposite material with enhanced electrochemical properties for the simultaneous determination of ascorbic acid, dopamine, uric acid and tryptophan. New J. Chem. 39, 1115–1124 (2015). https://doi.org/10.1039/c4nj01360c
T. Tite, E.A. Chiticaru, J.S. Burns, M. Ioniţǎ, Impact of nano-morphology, lattice defects and conductivity on the performance of graphene based electrochemical biosensors. J. Nanobiotechnol. 17, 101 (2019). https://doi.org/10.1186/s12951-019-0535-6
Acknowledgements
The author would like to acknowledge the support of the Principal, Jagdish Chandra DAV College, Dasuya, Distt. Hoshiarpur, Punjab, India (144205).
Author information
Authors and Affiliations
Corresponding author
Rights and permissions
Open Access This article is licensed under a Creative Commons Attribution 4.0 International License, which permits use, sharing, adaptation, distribution and reproduction in any medium or format, as long as you give appropriate credit to the original author(s) and the source, provide a link to the Creative Commons licence, and indicate if changes were made. The images or other third party material in this article are included in the article's Creative Commons licence, unless indicated otherwise in a credit line to the material. If material is not included in the article's Creative Commons licence and your intended use is not permitted by statutory regulation or exceeds the permitted use, you will need to obtain permission directly from the copyright holder. To view a copy of this licence, visit http://creativecommons.org/licenses/by/4.0/.
About this article
Cite this article
Kumar, R. NiCo2O4 Nano-/Microstructures as High-Performance Biosensors: A Review. Nano-Micro Lett. 12, 122 (2020). https://doi.org/10.1007/s40820-020-00462-w
Received:
Accepted:
Published:
DOI: https://doi.org/10.1007/s40820-020-00462-w