ABSTRACT
Every 42 years, the Earth and the Sun pass through the plane of the orbits of the main satellites of Uranus. In these occasions, mutual occultations and eclipses between these bodies can be seen from the Earth. The current Uranus equinox from 2007 to 2009 offers a precious opportunity to observe these events. Here, we present the analysis of five occultations and two eclipses observed from Brazil during 2007. For the reduction of the CCD images, we developed a digital coronagraphic method that removed the planet's scattered light around the satellites. A simple geometric model of the occultation/eclipse was used to fit the observed light curves. Dynamical quantities such as the impact parameter, the relative speed, and the central time of the event were then obtained with precisions of 7.6 km, 0.18 km s−1, and 2.9 s, respectively. These results can be further used to improve the parameters of the dynamical theories of the main Uranus satellites.
Export citation and abstract BibTeX RIS
1. INTRODUCTION
Besides the mutual events among the Jupiter Galilean moons, up to now the only other observed intersatellite occultations and eclipses were those of the Saturn system in 1995 (see, for example, Aksnes et al. 1984; Franklin et al. 1991; Arlot et al. 1992, 1997; Thuillot et al. 2001). The Jovian observations were used to improve the parameters of the dynamical theories of the Jupiter satellites (see, for example, Aksnes & Franklin 2001; Lainey et al. 2004). From the Saturn events, very precise satellite positions were obtained (Vasundhara et al. 2003; Noyelles et al. 2003).
Every 42 years, the Earth and the Sun pass through the orbital plane of the Uranus main satellites. The last Uranus equinox was in 1966. From 2007 to 2009, the Earth and the Sun are once more crossing the equatorial plane of Uranus. Since it will not occur again until 2050, this is a unique opportunity to observe mutual events among the Uranus five main satellites: Miranda, Ariel, Umbriel, Titania, and Oberon. Such observations can improve the ephemeris of the satellites and help us to map their surfaces (Christou 2005; Arlot et al. 2006).
Concerning the ephemeris, its match with contemporary intersatellite astrometric measurements reaches 50 mas for the four larger Uranus satellites and 100 mas for Miranda (Jones et al. 1998; Veiga & Vieira Martins 1999; Shen et al. 2002; Veiga et al. 2003). On the other hand, the errors on the determination of the centroids by modern techniques are as small as 20 mas. This evidences the presence of systematic errors and points out the need for improvements on the parameters of the dynamical models. Since the accuracy of the so-called astrometric photometry can be better than 10 mas, the observation of mutual events is a powerful tool to meet these requirements. Furthermore, in the determination of the parameters of the current theories of the Uranus system, most of the used astrometric positions of the satellites were obtained with their orbital planes faced-on. Now, in contrast, the orbital planes are edge-on, a scenario that favors the determination of inclinations.
Concerning the satellite surfaces, a systematic mapping was made by Voyager 2 in 1986 (Smith et al. 1986). However, at that time, their subsolar points were seen from near the South Pole. Now these points are near the equator and so the regions of the northern hemisphere are currently visible.
In order to provide precise predictions for the Uranus events, a number of tables were published by Christou (2005) and Arlot et al. (2006). These predictions are based on the analytic model GUST 86 (Laskar & Jacobson 1987) and on the numerical model LA06 (Lainey 2008). Using those tables, we have successfully observed seven foreseen events visible from the Laboratório Nacional de Astrofísica, Itajubá, Brazil (IAU code 874). Note that for the current Uranus equinox, only three events have been analyzed and published so far at the time of this writing. One was an occultation of Umbriel by Oberon (Hidas et al. 2008), another was an eclipse of Titania by Umbriel (Arlot et al. 2008), and a third was a predicted occultation of Oberon by Miranda which in fact was not detected (Birlan et al. 2008). A report on two other occultations—Titania and Ariel by Umbriel—is also in press (Miller & Chanover 2009). The one involving Ariel was also observed by us (see Section 4).
In the following, we describe our observations in Section 2 and the reduction procedures in Section 3. The results are presented in Section 4 and discussed in Section 5. In the Appendix, we describe in detail our general model for occultations and eclipses, including the expressions and derivatives used for fitting the observed light curves.
2. OBSERVATIONS
The observations were carried out with the 1.6 m, f/10, Perkin-Elmer reflector with a Ritchey–Chretien optics located at the Laboratório Nacional de Astrofísica, Itajubá, Brazil (λ = +45°35', ϕ = −22°32', h = 1860 m, IAU code = 874). The used detector was a thin, back-illuminated EEV CCD (model 02-06-1-206) with 385 × 578 pixels2 of 03 (22 μm). A near-infrared filter with a passband of 7200–1000 Å was used to minimize the Uranus scattered light. This filter associated with the CCD color sensitivity gives an effective passband of 7200–9000 Å which cuts the blue light from the planet (Karkoschka 1998). The CCD electronics allows for simultaneous integration and readout tasks during sequential exposures. Yet, to completely remove the time loss in between the exposures due to readout overhead, it was necessary for each event to optimally define a small window on the CCD array, so as to include just the two involved targets plus another satellite as flux calibrator. The planet was also integrally included in to allow for the digital coronagraphy of the images (see the following section).
From the foreseen events (Arlot et al. 2006), we successfully observed the light curves for five occultations and two eclipses. The predicted visibility of the recorded events is given in Table 1. They are the event date, the midtime (in UT), the event type (occ for occultation and ecl for eclipse, T for total and P for partial), the target satellites of the event (A, Ariel; U, Umbriel; T, Titania; O, Oberon; M, Miranda), and the flux drop (R band) of the occulted or eclipsed satellite. Observational information is also presented in this table: the solar phase angle, the distance to the planet center, the duration of the event, the mean zenithal distance, the exposure time, the number of fitted points (CCD frames), and the seeing.
Table 1. Predicted Visibility of the Recorded Events and Observational Information
Date | Midtime (UTC) | Event | |||||||||||||||
---|---|---|---|---|---|---|---|---|---|---|---|---|---|---|---|---|---|
yr | m | d | hr | m | s | Type | Flux Drop (R) | Dist. (UR) | Δt (s) | Solar Angle (°) | Zenithal Distance (°) | Exp. Time (s) | Frame Number | Seeing ('') | |||
2007 | 8 | 13 | 3 | 5 | 33 | A | occ | U | P | 0.264 | 6.3 | 627 | 1.35 | 31 | 3 | 549 | 2.0 |
2007 | 8 | 14 | 1 | 33 | 44 | U | occ | O | P | 0.094 | 7.1 | 346 | 1.31 | 50 | 3 | 485 | 1.1 |
2007 | 8 | 19 | 7 | 59 | 12 | U | occ | A | P | 0.318 | 6.2 | 877 | 1.11 | 54 | 3 | 650 | 1.4 |
2007 | 10 | 8 | 0 | 43 | 7 | A | occ | M | T | 0.130 | 1.7 | 152 | 1.36 | 17 | 1 | 856 | 2.6 |
2007 | 10 | 12 | 0 | 2 | 20 | T | ecl | M | T | 1.000 | 1.9 | 179 | 1.54 | 20 | 1 | 785 | 0.8 |
2007 | 10 | 18 | 0 | 28 | 30 | A | occ | M | P | 0.071 | 3.1 | 148 | 1.78 | 16 | 3 | 283 | 1.8 |
2007 | 11 | 28 | 1 | 41 | 15 | A | ecl | T | P | 0.198 | 5.9 | 400 | 2.77 | 60 | 2 | 627 | 3.8 |
Notes. Visibility data are based on the predictions by Arlot et al. (2006). Target satellites of the event: A, Ariel; U, Umbriel; T, Titania; O, Oberon; M, Miranda. Event type: occ stands for "occults," ecl for "eclipses," T for total, and P for partial. Distance to planet center in units of Uranus radius (UR = 2''). Δt = predicted event duration in seconds.
Download table as: ASCIITypeset image
Zenithal distances were always smaller than 60°, being smaller than 30° for four of the events. Seeing was smaller than 15 for three events. Exposure time varied from 1 to 3 s in a compromise between the signal-to-noise ratio (S/N) of the satellites' fluxes as opposed to the time resolution and to the contamination of the scattered light, which depends on the distance of the satellites from the planet and on the sky transparency. Acquisition started several minutes before the predicted start time of the event and continued for several minutes after its end. In all cases, a good sampling in time resolution was achieved with a high number of frames obtained during the event itself.
All five larger Uranus satellites, including Miranda, were eventually recorded on an occultation or on an eclipse. Among the occultations, only Titania was not observed. Among the eclipses, neither Umbriel nor Oberon was observed. The satellite distances from the Uranus center varied from 34 to 14'' (Uranus radius was 2''). Event durations varied from 2.5 to 14.5 minutes. Solar phase angles were about 1
5 for all the events but the last one in the year. The predicted types of all the events were confirmed by the analysis of the light curves, as will be seen in the following sections.
3. REDUCTIONS
In the following, we describe the digital coronagraphy applied to the images, the differential aperture photometry, and the fit of the occultation/eclipse model to the observed light curves. The software routines used for these tasks are part of the photometric module of the astrometric/photometric reduction package Platform for Reduction of Astronomical Images Automatically (PRAIA; Assafin 2006; Assafin et al. 2007).
3.1. Digital Coronagraphy
One of the main difficulties in the reduction of the images is the contamination of the scattered-light halo surrounding Uranus over the nearby satellites. Many processes were tested before the campaign of the mutual events (Assafin et al. 2008) and finally a digital coronagraphy method was adopted. This method consists of computing the bright source light profile within a given area of the digitized field. After this is done, the original image is subtracted from that profile and a new, digitally coronagraphed image is obtained.
The procedure is as follows. The count estimates for the light of the bright source are calculated for each pixel, at their exact geometric center. For each pixel (i, j), the distance d from its geometric center to the (xc, yc) central coordinate of the bright source is recorded. Then a ring of 1 pixel width, radius = d, and center (xc, yc) is established around the bright source center. Next, a histogram of the counts is produced based on the counts of each ring pixel (m, n). The (m, n) counts are weighed according to the small displacements between the geometric center of the (m, n) ring pixels and the exact ring circumference of radius = d. The mode of the histogram is then recorded as the best count estimate for the source light at the pixel (i, j). The procedure is repeated until all pixels (i, j) within the working area around the source are processed. The obtained source light profile is then subtracted from the original image to obtain the coronagraphed image of the field. The more accurate the (xc, yc) center is, the smaller the residual scattered light originating from the bright source becomes, and the better the coronagraphy result is.
This accurate centering is accomplished by applying the above procedure for an array of (xc, yc). First, an initial (xc, yc) estimate is obtained, based on a two-dimensional circular Gaussian fit. Then, starting from an array of 3 × 3 pixels around this bright center, we determine the pixel, then the subpixel (xc, yc) coordinates that give the least scattered residuals around the bright source figure. The procedure is repeated until the scattered residual does not change by more than a given convergence value (adopted as 1%). Of course, a better initial (xc, yc) estimate and a better computation of the scattered residuals are obtained if the entire planet image is present in the frame, as in our case. After we compute the most accurate center for the bright source by this method, we use the final derived coordinates (xc, yc), and apply the coronagraphy procedure once more; this time over an area large enough to encompass the objects of interest, in our case the Uranus satellites.
One should note that one advantage of the process is that it is relatively insensitive to the presence of diffraction spikes, bright objects nearby the source (like satellites), saturation artifacts in the source image or even to the existence of anomalous darker regions. This is because higher or lower count pixels will have low frequencies along the rings and thus will not affect the mode of the count histograms.
In Figure 1, we present a sample image of the August 14th occultation of Oberon by Umbriel. From left to right, we display the original image, the image of the computed planet brightness profile, and finally the output image digitally coronagraphed.
Figure 1. Digital coronagraphy over a sample image from the August 14th event. From left to right: original frame, Uranus brightness profile, and the output coronagraphed image. The satellites aligned from top to bottom are Titania (calibrator), Ariel, Miranda, and the event targets Umbriel and Oberon (indistinguishable one from the other).
Download figure:
Standard image High-resolution image3.2. Photometry
For each image, after bias subtraction and flat-field correction with the IRAF package, the digital coronagraphy is applied. Then, a differential aperture photometry is carried out on the pair of target satellites of the event and on another main satellite of Uranus, used as reference for flux calibration. The flux ratio between the total flux of the targets and that from the calibrator is thus derived. The S/N, the flux ratio error, and the seeing are calculated and the Julian date of each frame is stored. After processing all images, the observed light curve of the event is finally obtained.
The apparent visual magnitudes of the satellites are approximately Ariel = 14, Umbriel = 15, Titania = 14, Oberon = 14, Miranda = 16, as given by the JPL's Horizons ephemeris service (Giorgini et al. 1996). The brightest satellite farthest from the planet was always chosen as the flux calibrator. In most cases, it was Titania. For two of the events, it was possible to use two satellites as calibrators.
Usually, the sky background annulus around the objects had a radius of 20 pixels (6'') and a width of 4 pixels (12). For each event, many light curves were derived with different flux apertures for the satellites. The light curve with the circular aperture that yielded the least scatter (i.e., the highest S/N and least flux error) was selected for modeling analysis. Typically, the aperture radius was 2.5 times the value of seeing.
The photometric parameters used in each event are presented in Table 2: the event date, the target satellites of the event, the calibration satellite, the diameter of circular aperture (in units of seeing), and the average flux ratio error.
Table 2. Photometric Reduction Information
Event Date | ||||||||
---|---|---|---|---|---|---|---|---|
yr | m | d | Target Satellites | Calibration Satellites | Aperture (× Seeing) | Flux Ratio Error | ||
2007 | 8 | 13 | Ariel | occ | Umbriel | Titania | 2.5 | 0.0078 |
2007 | 8 | 14 | Umbriel | occ | Oberon | Titania | 2.8 | 0.0094 |
2007 | 8 | 19 | Umbriel | occ | Ariel | Titania | 2.6 | 0.0080 |
2007 | 10 | 8 | Ariel | occ | Miranda | Titania+Oberon | 1.2 | 0.0300 |
2007 | 10 | 12 | Titania | ecl | Miranda | Oberon | 2.8 | 0.0130 |
2007 | 10 | 18 | Ariel | occ | Miranda | Titania+Oberon | 1.8 | 0.0168 |
2007 | 11 | 28 | Ariel | ecl | Titania | Oberon | 1.0 | 0.0285 |
Notes. Apparent visual magnitudes from JPL are approximately Ariel = 14, Umbriel = 15, Titania = 14, Oberon = 14, Miranda = 16 (Giorgini et al. 1996). For two events, two satellites were used as flux calibrators. The diameter of circular aperture is in units of seeing.
Download table as: ASCIITypeset image
3.3. Modeling of Occultations and Eclipses and Fitting of the Observed Light Curves
We developed a simple geometrical model to fit the light curves. It serves both for occultations and eclipses, partial, total, annular, or central. The satellites are taken as gray spheres which scatter the sun light following the Lambert law. In this model, the flux ratio depends on three parameters associated with the dynamics of the satellites and three parameters associated with their geometry and reflectivity. The first dynamical parameter is the impact parameter. For the occultation, it is the minimum distance between the centers of the satellites and for eclipses it is the minimum distance between the center of the eclipsed satellite and the center of the cone of shadow projected by the other one. The second parameter is the relative speed of the two moons as seen from the observer for the occultations and from the Sun for the eclipses. The third is the central time of maximum occultation or eclipse. For all these quantities, we consider their projection on the plane of the sky. The parameter associated with reflectivity is the ratio of the albedos of the visible face of the satellites and the geometrical quantities are the apparent radii of the two satellites. In the Appendix, we develop a model giving all the expressions and their derivatives, for all cases. In particular, for the partial occultation case, the obtained formula is the same as that given in Hidas et al. (2008); for some related details, see also Wasserman et al. (1976).
The only drawback of the model is that it does not account for the solar phase angle. In our case, the phase angles are about 15, which amounts to a correction of the satellite center by 0.013r, where r is the radius of the satellite. For Titania (the largest satellite), the highest phase angle of all the observations was verified (2
77), which gives a center correction of about 21 km (1.5 mas). No penumbra was considered in the model, as the involved distances between the satellites in the eclipses were smaller than 9000 km, corresponding to penumbra widths smaller than 2 km.
We fitted each observed light curve to the model as follows. First, we normalized the curve adjusting the points outside the event (but not too far) with a polynomial model of nth degree. After some tests, we adopted n = 1 (straight line) for all events. Inclination of the fitted lines was almost negligible for all cases, indicating almost insignificant linear variations in the relative fluxes before and after the events, caused by displacements of objects from their fixed photometric apertures, due to seeing variation and high relative speed between the satellites. After that, all points in the curve (including the event) were divided by the fitted line. The resulting data points were thus the normalized calibrated flux sum of the two target satellites alone. Outside the event, in average this flux sum was equal to unity. The resulting light curve was then fitted by using a nonlinear least-squares procedure (see the Marquardt method in Bevington 1969). The initial guess of the model parameters in this iterative procedure came from the extracted JPL ephemeris (Giorgini et al. 1996) of the target satellites. After a fairly sufficient number of iterations (we conservatively adopted 100), we converged to a solution. Outlier points were excluded one by one in an iterative process, whenever the observed minus fitted flux residual was larger than 3σ. The entire procedure was repeated until no more outliers were found. The light-curve model parameters, their error estimates, and correlations were then finally stored.
In all fits, we fixed the values of the satellites radii to those observed by the Voyager 2 spacecraft (Thomas 1988). We also avoided the problem of strong correlation between the albedo ratio and the impact parameter by fixing the albedo ratios to values extrapolated from the ones given by Karkoschka (2001). In fact, these albedo ratios are consistent within the errors with some direct estimates that we are obtaining from images taken in the same runs before and after some of the events. The results of the investigation of these sets of observations will be published in a forthcoming paper.
4. RESULTS
The light curves of all the observed events are plotted in Figure 2. The differences between the observed points (normalized fluxes of target satellites) and the fitted curve are also shown. For display purposes, only the fraction of the curve encompassing the duration of the mutual event is presented.
Figure 2. Light curves of the seven mutual events between the main Uranus satellites, observed in Brazil during 2007, where occ stands for "occults" and ecl for "eclipses," A: Ariel, U: Umbriel, T: Titania, O: Oberon, and M: Miranda. For each plot, the points in the upper part are the observed normalized flux ratio of the satellites and the continuous line is the fitted curve. In the lower part, the (O − C) residuals of the fittings for each observed point are shown. For display purposes, only a fraction of the curve centered on the event is presented. Time is in UTC.
Download figure:
Standard image High-resolution imageOne distinct set of light curves comprises the three occultations from August. These events had long durations (more than 5 minutes) and involved the satellites Ariel, Umbriel, and Oberon, at distances from Uranus of about six times its radius. They were observed in good conditions of seeing (2'' or smaller) and with satisfactory exposure time (3 s). The fits were very good with the normalized χ2 smaller than 0.05. The errors in the central time, impact parameter, and relative speed were, respectively, about 1 s, 6 km, and 0.06 km s−1 or less. The occultation of Ariel by Umbriel on August 19th was also independently observed by Miller & Chanover (2009). They have obtained parameters and errors quite similar to our fits for this event.
Another set comprises the three light curves including Miranda. Events including this body will always be more difficult because of its relative faintness and proximity to Uranus as compared with the other satellites. The durations of the events with Miranda were always smaller than 3 minutes and were always within three planetary radii of Uranus. Seeing conditions varied from very good (08) to regular (2
6). The observation made on October 8th with seeing 2
6 was a total occultation of Miranda by Ariel. Its fitted impact parameter had an error of 82 km, which is not bad, but even so was 10 times larger than the typical errors obtained for the other events. The event on October 12th presented a very small but clear flux drop, successfully observed and measured due to very good seeing conditions (0
8).
The observation on November 28th was an eclipse of Titania by Ariel. The sky condition of this event was poor with seeing 38. The points of the light curve are very scattered and the errors in the determination of all parameters are relatively large with respect to the other fits.
The model parameters from the fittings of all seven observed events are presented in Table 3, together with their predicted values. In this table, "A" indicates our results. Predicted GUST86 (G) parameters were taken from Arlot et al. (2006) and from Christou (2005). LA06 (L) parameters were extracted from Arlot et al. (2006). The fixed albedo ratios used were extrapolated from Karkoschka (2001) to match the solar phase angle and filter passband of the observations. Also listed are the central time of the event, the impact parameter and the relative speed. For the three fitted parameters, their errors are presented as well as the mean error of the fit (i.e., the standard deviation of the observed minus fitted curve residuals) and the normalized χ2 of the fitted curve. For comparison purposes, the absolute and apparent radii of the satellites as taken from the Voyager 2 spacecraft (Thomas 1988) are also given at the bottom of the table.
Table 3. Results of the Observed Events
Central Time (UTC) | Impact Parameter | Relative Speed | |||||||||||||
---|---|---|---|---|---|---|---|---|---|---|---|---|---|---|---|
Ref. | Albedo Ratioa | hr | m | s | σ (s) | km | σ (km) | mas | σ (mas) | km s−1 | σ (km s−1) | mas s−1 | σ (mas s−1) | Mean Error | χ2 (Norm) |
Ariel occults Umbriel—2007 Aug 13 | |||||||||||||||
A | 1.8333 | 3 | 6 | 04.51 | 0.85 | 146.43 | 6.00 | 10.5 | 0.4 | 3.670 | 0.019 | 0.264 | 0.001 | 0.012 | 0.013 |
G | 3 | 4 | 10 | 19 | |||||||||||
L | 3 | 5 | 33 | 14 | |||||||||||
Umbriel occults Oberon—2007 Aug 14 | |||||||||||||||
A | 0.8136 | 1 | 34 | 00.81 | 1.19 | 720.22 | 2.77 | 51.8 | 0.2 | 6.283 | 0.060 | 0.452 | 0.004 | 0.014 | 0.046 |
G | 1 | 31 | 27 | 44 | |||||||||||
L | 1 | 33 | 44 | 65 | |||||||||||
Umbriel occults Ariel—2007 Aug 19 | |||||||||||||||
A | 0.5455 | 7 | 59 | 53.49 | 0.91 | 452.76 | 2.36 | 32.6 | 0.2 | 2.411 | 0.011 | 0.174 | 0.001 | 0.012 | 0.009 |
G | 7 | 57 | 27 | 65 | |||||||||||
L | 7 | 59 | 12 | 36 | |||||||||||
Ariel occults Miranda—2007 Oct 8 | |||||||||||||||
A | 1.1318 | 0 | 43 | 43.06 | 0.20 | 8.14 | 82.29 | 00.6 | 5.9 | 9.193 | 0.029 | 0.660 | 0.002 | 0.143 | 0.661 |
G | 0 | 43 | 23 | 30 | |||||||||||
L | 0 | 43 | 7 | 02 | |||||||||||
Titania eclipses Miranda—2007 Oct 12 | |||||||||||||||
A | 0.8585 | 0 | 3 | 24.14 | 5.31 | 863.87 | 9.18 | 61.9 | 0.7 | 5.084 | 0.499 | 0.364 | 0.036 | 0.023 | 0.821 |
G | 0 | 1 | 41 | 56 | |||||||||||
L | 0 | 2 | 20 | 27 | |||||||||||
Ariel occults Miranda—2007 Oct 18 | |||||||||||||||
A | 1.1318 | 0 | 28 | 48.28 | 3.42 | 484.23 | 10.81 | 34.6 | 0.8 | 7.870 | 0.380 | 0.562 | 0.027 | 0.012 | 0.312 |
G | 0 | 28 | 39 | 100 | |||||||||||
L | 0 | 28 | 30 | 39 | |||||||||||
Ariel eclipses Titania—2007 Nov 28 | |||||||||||||||
A | 1.4384 | 1 | 41 | 48.47 | 8.41 | 883.58 | 14.67 | 61.2 | 1.0 | 4.814 | 0.256 | 0.333 | 0.018 | 0.066 | 0.721 |
G | 1 | 39 | 23 | 38 | |||||||||||
L | 1 | 41 | 15 | 59 |
Notes. In the first column, "A" refers to our results. Respective predicted GUST86 (G) and LA06 (L) values were taken from Arlot et al. (2006) and from Christou (2005). The mean error is the standard deviation of the observed minus fitted curve residuals. χ2 is the normalized χ2 of the fitted curve points (values smaller than 1 indicates good fitting). The statistical significance was not smaller than 0.99 for all fittings. The absolute and approximate apparent radii of the satellites as taken from the Voyager 2 spacecraft (Thomas 1988) are 236 km (17 mas) for Miranda, 579 km (42 mas) for Ariel, 585 km (42 mas) for Umbriel, 789 km (55 mas) for Titania, and 761 km (55 mas) for Oberon. aFixed albedo ratios used for each event, extrapolated from Karkoschka (2001).
Download table as: ASCIITypeset image
As can be seen from Table 3, all curves were satisfactorily fitted as the normalized χ2 was always smaller than 1. The statistical significance was not smaller than 0.99 for all fittings. The relative speed and the impact parameter were fitted with errors ten times smaller than their values. In all events, almost all the values found are closer to those predicted from LA06. Even so, the differences are still significant, being frequently much larger than three times the parameter errors.
5. DISCUSSION
We observed and reduced seven mutual events of the Uranus satellites and compared the results with two different predictions. Like in Hidas et al. (2008), we find that our observations are in closer agreement with LA06, with offsets not larger than 200 km, thus within the LA06 estimated errors of a few hundred kilometers (Lainey 2008). However, considering the small errors obtained for the dynamical parameters, we conclude that our results can be used to further improve the ephemerids of the main satellites. In fact, the errors were 2.9 s for the central time, 7.6 km (0.54 mas) for the impact parameter (excluding the event on October 8th), and 0.18 km s−1 (0.013 mas s−1) for the relative speed.
These results were achieved due to two important reduction steps, carried out by the use of the PRAIA reduction package. The first one was to perform a digital coronagraphy of the images to flatten the sky flux regardless of the proximity to the planet. The second one was to use a simple model to describe partial and total occultations and eclipses. In fact, with the fitting of the model to the light curves we could evaluate the correlations between the parameters and determine that the correlation between impact parameter and albedo ratio was about 95%. So, like in Hidas et al (2008), we used albedo ratio values extrapolated from Karkoschka (2001). Nevertheless, these values may not yet represent perfectly well the actual albedo ratios since the conditions of our observations, such as wavelength passband, solar phase angle, and the part of the surface observed of every satellite, were not the same as those of the Karkoschka observations.
Our results can be improved if we determine directly the albedo ratios from the images obtained before and after the events. In fact, for almost all events, we observed the Uranus system for many hours. Since the rotation velocity of the satellites is very small, the photometry of the satellites—once photometrically resolved—should yield albedo ratios very similar to those of the corresponding mutual event.
Our light curve analytic model also allows for the evaluation of local albedo variations. In fact, a decentered spot with distinct albedo in the surface of the occulted body will result in an asymmetric light curve (see Wasserman et al. 1976). Taking the derivative to the first order of the normalized flux ratio with respect to the albedo ratio from our model, one can easily estimate this asymmetry as a function of the spot's diameter and albedo. The actual detection of such spots is nevertheless limited to light curves of extreme quality derived from very high S/N observations. For instance, using the model for the August 13th occultation, it would take a spot with 17% of the occulted body radius and with twice its albedo to create a flux discrepancy (asymmetry) of twice the observed flux ratio error for measurements before and after the event midtime.
Penumbra widths were estimated in less than 2 km and thus were not considered here. On the other hand, improvements can be made including the solar phase angle in the model. In fact, in Table 1, we can see that the phases of the satellites were typically around 15, but for the highest value of 2
77, involving the largest moon Titania, we get a positional shift of about 21 km (1.5 mas). This is a small contribution considering the impact parameter values alone, but is more than three times the typical errors of 0.2 mas from our best observations.
M.A. acknowledges grant E-26/170.686/2004/FAPERJ and grants 306028/2005-0 and 478318/2007-3 (CNPq). F.B.R. thanks the financial support by the CAPES. J.I.B.C. acknowledges grant 151392/2005-6/CNPq and grant E-26/100.229/2008/FAPERJ. A.H.A. thanks grant 307126/2006-4 ("Produtividade em Pesquisa" CNPq). R.V.M. acknowledges grant 304124/2007-9/CNPq.
APPENDIX: MODEL FOR TOTAL AND PARTIAL OCCULTATIONS AND ECLIPSES: ANALYTICAL EXPRESSIONS AND DERIVATIVES
A simple geometrical model was developed to fit the light curves of occultations and eclipses, partial, total, annular, or central. Here, we describe in detail the analytical expressions and their derivatives with respect to the parameters of the model. The expressions shown in this appendix can be readily used in nonlinear least-squares procedures to fit the observed light curves of any mutual event in general. First, we obtain the expressions for occultations. Then, it is explained why the same formalism developed for occultations also applies for the eclipses.
In the following, we always refer to apparent sizes regardless of the actual sizes of the bodies. The basic hypothesis is that the satellites are illuminated spheres which scatter the light equally in all directions (Lambert law). So, the satellites illuminated by the sun light are seen from the Earth-like two gray disks. Let us then consider the geometry of a partial occultation as shown in Figure 3, where two disks S1 and S2 of radius R2 < R1 partially intercept each other. The intercepted area A of occultation is then

where S(O1BC) and S(O2BC) are the areas of the circular sectors of the disks S1 and S2, respectively, and Δ(O1BC) and Δ(O2BC) are the areas of the triangles. But

so that

Let k1 and k2 be the albedos of the satellites 1 and 2. Then, when the larger body S1 occults the smaller S2, their total flux F1o2 is

Now, let F1+2 be the sum of the fluxes of the satellites 1 and 2 without occultation. Thus, the normalized flux Focc of the two satellites, i.e., the total flux F1o2 divided by F1+2, when the larger body S1 occults the smaller S2, is given by

The angles in Focc are easily calculated from the distance d = O1O2 and from the satellites' apparent radii. Considering the triangle O1O2B, from the cosine law we have for α1 and α2

where i = 1 or 2 and j = 2 or 1, respectively.
Figure 3. Geometry of a partial occultation where the disks S1 and S2 of radius R2 < R1 partially intercept each other by an area A (see the Appendix).
Download figure:
Standard image High-resolution imageNote that in Figure 3 the distance d is larger than R2. However, for d < R2, the intercepted area A between the disks is now given by

Even so, the expressions for F1o2 and thus for Focc are still valid, since .
Now, if the smaller satellite S2 passes in front of the larger one S1, the formal expressions deduced above still hold for Focc, once we replace the albedos k1 by k2 and vice versa.
In the case of a total occultation, when d < R1 − R2, there are two situations depending on what body is in front of the other. When it is the smaller satellite S2 being occulted by the larger one S1, then we have
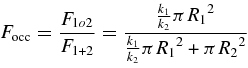
and when it is the smaller satellite S2 which is in front of the larger one S1
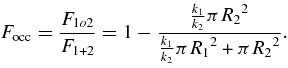
The dynamics of occultations and of eclipses is defined by the relative motion between the satellites. This is given by

where x is the impact parameter, v is the relative speed between the satellites, and t0 is the central time. Therefore, these three dynamical parameters can be associated with the geometry of the occultation through the distance d between the satellites, which in turn determines the flux ratio Focc of the observed light curve. Thus, the model of the light curve depends on four parameters: the albedo ratio , x, v, and to. In compact form, the model can be written as
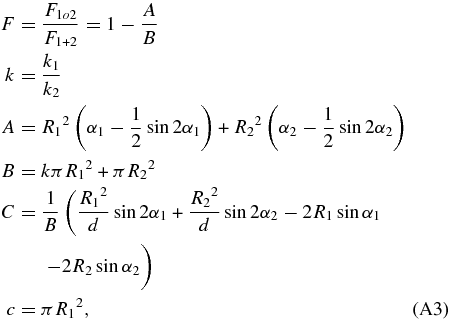
where the angles α are computed from Equation (A1), using Equation (A2). As before, in the case that the larger satellite S1 is behind the smaller one S2, we must exchange the albedos indices in k in Equations (A3).
We usually need the derivatives to the first order to solve for the model parameters in a nonlinear least-squares procedure to fit the flux ratio of the observed light curve (Bevington 1969). We start with initial parameter values taken from the JPL's Horizons ephemeris service (Giorgini et al. 1996) and, using the derivatives, we solve for the small parameter increments in an iterative least-squares procedure until the parameter values converge. These derivatives are not difficult to calculate and so we present here only the results. Thus, in the case of partial occultations, when the larger body S1 occults the smaller one S2, we have
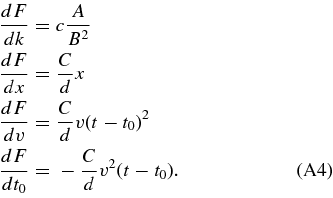
In total occultations, we have two cases for the derivative with respect to the albedo ratio. If the smaller satellite S2 is being occulted, then

and, in contrast,

All expressions developed here for the occultations are rigorously valid for eclipses, once we replace the occulting/occulted body radius and albedos by those of the eclipsing/eclipsed body. The reason is that both eclipsing and eclipsed objects cannot be resolved in the images, due to their relative proximity as compared to their distance from the observer. Therefore, the flux of the eclipsing body is inevitably added to that of the eclipsed one in the photometric measurements. In this case, the resulting measured total flux F1e2 and the normalized flux Fecl of eclipses can then be described exactly with the same formalism of the total flux F1o2 and normalized flux Focc of occultations. Finally, it is easily verified that either annular or central events are also taken into account in the above formalism. In practice, Equations (A1)–(A6) can thus be used in any case for fitting light curves of mutual events.
An issue is the strong correlation between the impact parameter and the albedo ratio. In the present work, we did not adjust albedo ratios in the light curve fittings, but rather took fixed values and only adjusted the other three purely dynamical parameters.
Note that an eclipse is an occultation as seen from the Sun so that, for ephemeris-improving purposes, a correct interpretation of the fitted parameters must be done according to the type of mutual event. For example, when considering the listed central times in occultations we must discount the light times of each satellite with respect to the observer, but in the case of eclipses the light time between the two satellites must also be considered.
It must be emphasized that the solar phase angle and the penumbra in the model above were not considered. In fact, they are small for the Uranus mutual events.
Footnotes
- *
Based on observations made at Laboratório Nacional de Astrofísica (LNA), Itajubá-MG, Brazil.