Abstract
Ultrafast imaging using plane or diverging waves has recently enabled new ultrasound imaging modes with improved sensitivity and very high frame rates. Some of these new imaging modalities include shear wave elastography, ultrafast Doppler, ultrafast contrast-enhanced imaging and functional ultrasound imaging. Even though ultrafast imaging already encounters clinical success, increasing even more its penetration depth and signal-to-noise ratio for dedicated applications would be valuable.
Ultrafast imaging relies on the coherent compounding of backscattered echoes resulting from successive tilted plane waves emissions; this produces high-resolution ultrasound images with a trade-off between final frame rate, contrast and resolution. In this work, we introduce multiplane wave imaging, a new method that strongly improves ultrafast images signal-to-noise ratio by virtually increasing the emission signal amplitude without compromising the frame rate. This method relies on the successive transmissions of multiple plane waves with differently coded amplitudes and emission angles in a single transmit event. Data from each single plane wave of increased amplitude can then be obtained, by recombining the received data of successive events with the proper coefficients.
The benefits of multiplane wave for B-mode, shear wave elastography and ultrafast Doppler imaging are experimentally demonstrated. Multiplane wave with 4 plane waves emissions yields a 5.8 ± 0.5 dB increase in signal-to-noise ratio and approximately 10 mm in penetration in a calibrated ultrasound phantom (0.7 d MHz−1 cm−1). In shear wave elastography, the same multiplane wave configuration yields a 2.07 ± 0.05 fold reduction of the particle velocity standard deviation and a two-fold reduction of the shear wave velocity maps standard deviation. In functional ultrasound imaging, the mapping of cerebral blood volume results in a 3 to 6 dB increase of the contrast-to-noise ratio in deep structures of the rodent brain.
Export citation and abstract BibTeX RIS

Content from this work may be used under the terms of the Creative Commons Attribution 3.0 licence. Any further distribution of this work must maintain attribution to the author(s) and the title of the work, journal citation and DOI.
1. Introduction
Ultrafast plane wave imaging was introduced in the last decade as a way to maximize ultrasound imaging frame rate, with the initial goal of tracking shear wave propagation within the body (Tanter et al 2008). In order to reach the highest frame rate, a single plane wave is transmitted instead of line-per-line focused beams. This allows imaging frame rates up to 10 000 frames per second, depending only on the imaging depth. In this plane wave imaging approach there is no focalisation in transmission, but thanks to conventional beamforming in reception, the received data can be used to retrieve the localization of echoes. This lack of transmit focalization inevitably leads to poorer image quality, especially in terms of contrast, but ultrafast plane wave imaging still offered a new window on fast and transient phenomena.
Recently, our group developed a new way to improve the performance of ultrafast plane wave imaging by using coherent compounding of several tilted plane waves (Montaldo et al 2009, Bercoff et al 2011). By using appropriate time delays, the transmit focalization can be synthetically recreated in each pixel of the image thanks to the coherent summing of the backscattered echoes of a few plane waves transmitted with different angles. Such a coherent recombination was proposed in the framework of synthetic imaging for increased image quality (Karaman et al 1995, Lockwood et al 1998, Nikolov and Jensen 2003, Udesen et al 2008). Plane wave coherent compounding has been demonstrated to allow the realization of B-mode equivalent quality images as in the standard focused approach with only a third of the insonifications (Montaldo et al 2009), thus achieving both high quality and high frame rate, even in the case of very rapidly moving targets (Denarie et al 2013). A clear trade-off between high quality and high frame rate can be defined on the number of angles. This concept has then been applied to shear wave elastography and later to Doppler imaging (Bercoff et al 2011) and ultrafast diverging wave imaging (Papadacci et al 2014), and is now at the core of ultrafast imaging. The large number of current and potential future applications embraced by ultrafast ultrasound imaging (Tanter and Fink 2014) encourages the development of optimized sequences to improve the contrast, the signal-to-noise and the resolution of ultrafast images.
This paper investigates one of these improvements, as multiplane wave imaging is an ultrafast imaging technique based on coded coherent plane wave compounding that aims at increasing the signal-to-noise ratio of ultrafast images without compromising neither frame rate nor resolution.
The multiplane wave method basic principles are detailed in section 2. Multiplane wave imaging performance is compared to the classical plane wave coherent compounding technique in section 3 in phantom B-mode acquisitions. Then multiplane wave imaging is also applied to shear wave elastography imaging and ultrafast Doppler imaging. We demonstrate how this novel imaging approach improves signal-to-noise ratio (SNR) and contrast of B-mode images, how it provides accurate elasticity maps with smaller variance for elastography applications, and how it detects deeper blood signals in Doppler mode. This technique could be of great interest for deep observations in the tissues where attenuation weakens the signal.
2. Multiplane wave imaging basic principles
A schematic representation of the classical coherent plane wave compounding method with N = 2 plane waves is represented in figure 1(a) (left part). At time T0 the medium is insonified with a first plane wave tilted with an angle α1 and the backscattered echoes are recorded. Then, at time T1, the medium is insonified with a second plane wave tilted with an angle α2 and the backscattered echoes are recorded. After delay-and-sum beamforming of the received data, two low quality radio frequency (RF) images are obtained. The coherent addition of these two images generates a higher quality image as described in Montaldo et al (2009). Each individual plane wave is transmitted with a fixed Pulse Repetition Frequency (PRF) which is limited by the propagation time, i.e. by the imaging depth. In the N = 2 plane waves example, two images from two different plane waves are required to create a single final image; as a result the final frame rate is half the PRF. More generally the final frame rate is equal to the PRF divided by the number of plane waves used in the coherent summation. Thus, the more plane waves are used for the final image, the better its quality but also the lower the frame rate. This highlights the conventional trade-off between image quality and frame rate of coherent plane wave compounding.
Figure 1. (a) Coherent plane wave compounding with N = 2 plane waves. (b) Multiplane wave compounding with N = 2 plane waves.
Download figure:
Standard image High-resolution imageMultiplane wave imaging provides further improvement of the image signal-to-noise ratio for a given frame rate with an extremely simple mathematical and hardware implementation. The multiplane wave method improves the quality of final images by artificially increasing the amplitude of the transmit signal without compromising the frame rate. Indeed, the emitted waveform amplitude is often limited by ultrasound transducer electronic circuit characteristics, probe design or eventually by safety considerations such as Mechanical Index limits. Thus, as the amplitude is often already close to its maximum value, increasing the transmit amplitude is not directly possible.
The original solution proposed in this work is schematically represented in figure 1(b) (left part) in the case of N = 2 plane waves. At transmit time T0, two wavefronts tilted with two different angles α1 and α2 are quasi simultaneously transmitted into the medium with a very small interleaved delay dt. A multiplicative factor +1 is applied on both wavefronts. The backscattered echoes are received and stored in memories. At transmit time T1, the same wavefronts are transmitted again, but this time with multiplicative factors +1 for the first wavefront and −1 for the second one. Once again, the resulting echoes are received and stored in memories. At this step there are two RF images, one for each acquisition at times T0 and T1. Then the two images are coherently summed: in a first combination, the contributions of angle α2 disappear in the linear regime of propagation thanks to the multiplicative factors applied on the angle α2 ((+1) + (−1) = 0), whereas the contributions of angle α1 are added ((+1) + (+1) = 2). Thus the summation enables to obtain the same image as if only a single transmit, tilted with an angle α1, was sent with double amplitude. In a second combination, the subtraction of the two images makes the contributions of angle α1 disappear ((+1) − (+1) = 0) while contributions for angle α2 add up ((+1) − (−1) = 2). The subtraction enables to obtain the same image as if only a single transmit, this time tilted with an angle α2, was also sent with double amplitude. Finally, the time delay dt between the two tilted plane waves is compensated for, and the two resulting images are coherently summed to obtain the final image, as done in the coherent plane wave compounding method.
The time between two consecutive images is only limited by the propagation time in the medium. As a consequence, if this set of multiplane waves is sent with the same plane wave coherent compounding technique PRF, the final frame rate will also be equal to PRF/N (in this example PRF/2). Thus, the multiplane approach leads to a virtual increase of the transmit signal amplitude without compromising the ultrafast frame rate.
In this multiplane wave example (figure 1(b)), since the emission matrix contains the two plane waves, its duration is at least twice longer (figures 1(a) and (b) right parts), resulting in a larger blind near field zone where the image cannot be reconstructed. Furthermore, a fixed pause approximately as long as the duration of the wavefront has been introduced between two plane waves to let transducers return to zero and avoid artefacts. Since the ultrasonic device cannot receive backscattered echoes until the emission is over, and as the emission matrix is longer in the multiplane wave case, the blind area on the surface of the image due to the emission duration is larger. The blind area is defined as dblind~ c × tem/2, with c the ultrasound celerity and tem the emission duration. In this example (figures 1(a) and (b) right part) the classical coherent plane wave compounding method with α = ±1° gives dblind ~1540 × 0.8 × 10−6/2 ~ 0.6 mm, whereas the N = 2 multiplane wave case with same inclination (α = ±1°) gives a larger blind area: dblind ~ 1540 × 2.4 × 10−6/2 ~ 1.8 mm. The blind area remains negligible for a small number of plane waves and for weak inclinations, but has to be taken into account in the case of a higher number of plane waves or higher inclinations. Fortunately, such a high number of plane waves will be mostly required for imaging deeper structures, relatively to the probe width.
The multiplane wave compounding method introduced with N = 2 plane waves can be generalized to any values of N. An appropriate combination of multiplicative factors +1 and −1 has to be applied to each set of plane waves, in order to reconstruct each plane wave of the set as if it had been sent separately from the others. In this work, an Hadamard matrix has been used because it creates independent vectors with +1 and −1 components (i.e. without losing the maximum amplitude level for each transmission). Hadamard matrices have many applications in signal processing, coding or cryptography (Horadam 2007) and have already been applied to synthetic aperture imaging in Ultrasound (Chiao et al 1997, Mosca et al 2008). An Hadamard matrix is a square matrix with +1 or −1 coefficients and whose rows are mutually orthogonal. One of the interesting properties of these matrices is that the multiplication of HN (Hadamard matrix of order N) by its transpose is equal to N times the identity matrix:

This property is used for the multiplane wave emission matrix, in order to reconstruct each single plane wave separately. The order of an Hadamard matrix must be 1, 2 or a multiple of 4. The smallest Hadamard matrix is H1 = [1]. The following ones, of order 2k (with 2 ⩽ k ∈ N), can be computed using Sylvester's construction (Sylvester 1867):
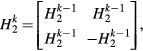
with H2 the Hadamard matrix of order 2, . The column coefficients of HN can been seen as the multiplicative factors used for the different emissions, whereas the row coefficients represent the combination of subtraction or addition operations needed to retrieve one plane wave individually with an amplitude N and cancel all the others (figure 2).
Figure 2. (a) Schematic representation of multiplane wave case with N = 4 plane waves. (b) Example of Hadamard matrix with N = 4.
Download figure:
Standard image High-resolution imageIn this work, the Hadamard basis was used with N ranging from 2 to 32 plane waves.
The virtual increase of the emitted amplitude obtained by coherent summation is expected to result in an overall increase of the signal-to-noise ratio of the image. Assuming a white electronic noise in the acquisition boards, the time standard deviation of each pixel in the image should be divided by , ie a SNR gain of 10 ⋅ log10(N) on the energy. When electronic noise is dominant over side lobes and clutter, such as in deep structures, a significant increase of the contrast can be expected compared to coherent plane wave compounding. Similarly, the improvement of the signal-to-noise ratio has a direct impact on other imaging modalities based on ultrafast imaging, such as shear wave elastography or ultrafast Doppler imaging.
3. Results
3.1. Performance quantification of B-mode imaging in phantom
The sequence was implemented on an AixplorerTM ultrasound system (SuperSonic Imagine, Aix-en-Provence, France) running Matlab (MathWorks, Natick, Massachusetts, USA). The performance was assessed both in terms of signal-to-noise ratio and in terms of contrast using an imaging phantom (Small parts phantom, model 551, ATS laboratories, Bridgeport, USA) of 3 mm diameter anechoic inclusions embedded in a homogeneous speckle environment. The 128 first elements of a 160-element array working at 6 MHz central frequency with a 0.2 mm pitch (SuperSonic Imagine, Aix-en-Provence, France) were used and the receive bandwidth of the scanner was set at 90% using the on board finite impulse response (FIR) filter. One hundred frames were acquired both with coherent plane wave compounding and multiplane wave imaging.
In order to compare plane wave and multiplane wave image quality the SNR has been plotted as a function of depth. For each depth, the SNR has been averaged in the probe axis direction over a 10 pixels area containing inclusions. For each pixel, the ultrasonic signal has been computed as the mean over the 100 images and the noise as the standard deviation.
The performance was also assessed in terms of contrast. The contrast represents the ability of the imaging method to detect an anechoic object embedded in a homogeneous scattering medium. The contrast has been computed as follows:
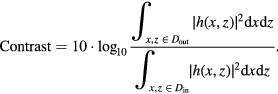
The numerator represents the background signal. It is computed as the mean of the 100 images in the chosen region of interest (ROI) close to the inclusion (Dout, 10 × 10 pixels = 2 mm × 2.4 mm rectangle). The denominator represents the anechoic inclusion signal and is computed as the mean of the 100 images in the chosen ROI in the inclusion area (Din, same size as Dout).
SNR and contrast have been quantified for N ranging from 2 to 32 plane waves. In the case of a small number of plane waves, it is interesting to use a relatively high angular step between two successive plane waves (in this case
). Such a value ensures a good decorrelation of the received signals in the compounding step even with a low number of plane waves. A small number of plane waves further achieves a very high frame rate. Thus, this strategy is typically employed for ultrafast imaging based modalities. In case where a large number of plane waves is preferred, in order to perform higher quality imaging at lower frame rate, this configuration will quickly yield angular values higher than the directivity of the probe. In the case of a very high number of plane waves it is thus better to use an alternative strategy where a fixed maximal angular aperture
is chosen within the probe directivity, and with a variable angular step
derived from the number of plane waves. In such a scenario, the frame rate is decreasing with the number of plane waves but quality is increasing.
3.1.1. High frame rate configuration with small number of plane waves.
SNR graphs as a function of depth were plotted in figure 3(a) for N = 2, 4 and 8 plane waves with a fixed between two successive plane waves. The blue thin curves represent the compound method and the red thick ones the multiplane method. Because of ultrasonic attenuation, the amplitude of the backscattered echoes decreases exponentially with depth, and as a result so does the recorded signal. On the contrary, noise mainly comes from electronics and can be assumed uniform in the image, whatever the depth. As a result the SNR, defined as the ratio between signal and noise, is decreasing exponentially with depth, as shown on all the SNR graphs in figure 3(a). The multiplane wave method always gives a better SNR than the compound method. Indeed, while the multiplane wave method emits N = 2, 4 or 8 plane waves at the same time, the compound method only emits 1 plane wave per emission. As the reconstructed signal is expected to be N times higher with multiplane and as the electronic noise is equivalent, the theoretical gain is
, NemissionMP being the number of plane waves sent at each emission with the multiplane wave method. Gains of Gth(2) = 3 dB,
and
can be assumed, which is consistent with the gains experimentally measured Gexp(2) = 2.8 ± 0.4 dB, Gexp(4) = 5.8 ± 0.5 dB, and Gexp(8) = 8.3 ± 0.6 dB. The multiplane wave imaging SNR improvement gets more visible with depth. While the blue curves—plane wave compounding method—are almost totally flat and equal to zero in the area of the 5th inclusion (around depth 60 mm), the last two red curves (N = 4 and 8)—multiplane wave method—highlight the presence of the inclusion.
Figure 3. Quantification in terms of SNR and contrast using a small number of plane waves: comparison between coherent plane wave compounding (blue thin line) and multiplane wave (red thick line) using N = 2, 4 or 8 tilted plane waves with . (a) Signal-to-noise ratio as a function of depth computed on an area containing inclusions. Note the SNR increase obtained with multiplane wave for the last inclusions. Gains experimentally measured (Gexp(2) = 2.8 ± 0.4 dB, Gexp(4) = 5.8 ± 0.5 dB, and Gexp(8) = 8.3 ± 0.6 dB) are consistent with theoretical gains:
, which corresponds respectively to 3 dB, 6 dB and 9 dB. (b) Corresponding B-mode images. (c) Contrast as a function of the number of plane waves used, for an inclusion close to the surface of the phantom (30 mm—solid line) and an inclusion deeper (60 mm—dotted line).
Download figure:
Standard image High-resolution imageAn improvement of image quality can clearly be seen in figure 3(b) where compound and multiplane wave images are compared side by side for the same number of plane waves. The multiplane wave method exhibits a visible improvement in deep areas. The deepest inclusion (around the depth 60 mm) emerges with the multiplane wave compound method using N = 4 plane waves, it is clearly visible with N = 8 plane waves, while it remains totally invisible with the coherent plane waves compound method, even with 8 plane waves. For both the methods, close to the surface the contrast improves when the number of plane waves increases. For the same number of plane waves - which also means the same frame rate—the contrast close to the surface increases slightly when using the multiplane wave method. These naked eye observations are confirmed with the graphs plotted in figure 3(c).
Two inclusions were investigated in figure 3(c): one close to the phantom surface (30 mm, framed with solid line) and another one deeper in the phantom (60 mm, framed with dotted line). The contrast of the inclusion closest to the surface is clearly improved by adding plane waves with both methods, yet the multiplane wave method only slightly improves contrast for the same amount of plane waves (figure 3(c) solid line). On the contrary, in deeper regions, the main factor improving contrast is the use of the multiplane wave method (figure 3(c) dotted line). With N = 8 plane waves, the gain of multiplane wave versus compound method is 4 dB, whereas the use of 8 plane waves instead of 4 with classical compound method leads to almost no gain.
3.1.2. High quality configuration with large number of plane waves.
In figure 4, the performance of plane wave compounding and multiplane wave imaging are compared, using a high number of plane waves (up to N = 32 plane waves) with a fixed angular aperture. is set at 9°, which ensures an angular step
(the lowest useful value according to equation (12) of Montaldo et al (2009) for this probe) even for N = 32 plane waves. The blue thin curves represent the compound method and the red thick curves the multiplane method. Once again the SNR experimental gains Gexp(8) = 8.3 ± 0.7 dB, Gexp(16) = 11.4 ± 0.7 dB, and Gexp(32) = 13.5 ± 0.6 dB, are consistent with the theoretical gains Gth(8) = 9 dB, Gth(16) = 12 dB, and Gth(32) = 15 dB, computed using
, as seen in figure 4(a). The B-mode images also highlight the improvement of the image quality in depth thanks to multiplane wave imaging (figure 4(b)). The inclusion around the depth 60 mm remains invisible with plane wave compounding imaging, even with N = 32 plane waves, but is visible with multiplane wave imaging from N = 8 plane waves. For a high number of plane waves, the angular step
becomes smaller, the plane waves are less decorrelated and the contrast starts to converge in figure 4(c).
Figure 4. Quantification in terms of SNR and contrast for a high number of plane waves: comparison between coherent plane wave compounding (blue thin lines) and multiplane wave imaging (red thick lines). (a) SNR as a function of depth, computed on an area containing inclusions. (b) B-mode images using N = 8, 16 or 32 tilted plane waves with a fixed . The squares depict the localization of the inclusions used for contrast quantification in figure 4(c). (c) Contrast as a function of the number of plane waves used, for an inclusion close to the surface of the phantom (30 mm: solid line) and two inclusions deeper (~50 mm: dashed line and ~60 mm: dotted line).
Download figure:
Standard image High-resolution imageFigure 5. Shear wave imaging (elastography) on breast phantom using 4 angles (−3, −1, 1, 3°) using (a) coherent plane wave compounding or (b) multiplane wave imaging.
Download figure:
Standard image High-resolution imageFinally, for both small and large number of plane waves cases, the maximum achievable frame rate has been computed as with tB/F the back and forth time for the ultrasonic wave to reach the maximum imaging depth dmax (dmax = 80 mm in these B-mode acquisitions) and N the number of plane waves. The values computed for N = 2, 4, 8 and N = 8, 16, 32 plane waves are respectively displayed in figures 3(a) and 4(a). Multiplane wave imaging has been previously introduced as a way to increase SNR without compromising frame rate but it can also be seen as a method to achieve a higher frame rate than plane wave compounding, with equivalent image quality.
As a conclusion, we experimentally confirmed here that the multiplane wave compound method performs better in terms of SNR and contrast, especially at depths where the electronic noise is the main factor of contrast loss. The use of the multiplane wave technique is of great help as it improves the image quality in terms of SNR but also in terms of contrast in deeper areas without decreasing the frame rate. In the following part, two modalities dedicated to applications that require a very high frame rate are presented: shear wave imaging (elastography) and ultrafast Doppler imaging. The benefits of the method will be investigated for both applications.
3.2. Shear wave imaging
In human soft tissues, low-frequency shear waves, typically 50 to 500 Hz, propagate at a very low speed (~1 to 10 m s−1). Shear wave elastography imaging consists in imaging the tissue displacement induced by the shear waves by using compressional ultrasonic waves propagating at a high speed, around 1500 m s−1. To observe the propagation of the shear wave, the frame rate must reach values typically higher than 1000 Hz. In this study, shear waves are generated by the acoustic radiation force of a 150 μs ultrasonic focused beam using the Supersonic Shear Imaging technique (Tanter et al 2008). The sequence was implemented on an AixplorerTM ultrasound system (SuperSonic Imagine, Aix-en-Provence, France) running Matlab (MathWorks, Natick, Massachusetts, USA). The 128 first elements of a 160-element array working at 6 MHz central frequency with a 0.2 mm pitch (SuperSonic Imagine, Aix-en-Provence, France) were used and the receive bandwidth of the scanner was set at 90% using the on board FIR filter.
The shear wave propagation induced in a breast phantom (breast elastography phantom model 059, CIRS, Norfolk, USA) was imaged using 4 plane waves (−3,−1, 1, 3°) with coherent plane wave compounding (figure 5(a)) or with multiplane wave imaging (figure 5(b)). In both cases the PRF was set at 11.7 kHz. After compounding, the echographic images were thus obtained at a 2.9 kHz frame rate.
For both sequence, the time standard deviation of the particle velocity was quantified long after the shear wave propagation (N = 10 images) and spatially averaged in the square ROI depicted in figure 5. Standard deviations of σPlaneWave = 0,329 ± 0.004 mm s−1, and σMultiplaneWave = 0,159 ± 0.002 mm s−1 were measured (estimated over Nacq = 10 acquisitions). As expected, the multiplane wave sequence with N = 4 yields a two-fold reduction of the particle velocity time standard deviation (σPlaneWave/σMultiplaneWave = 2.07 ± 0.05). This value is consistent with the theory in the case of 4 plane waves emission. This reduction in noise thanks to multiplane wave imaging can be seen in the tissue velocity images displayed in figure 5, especially in depth, as shown in the square ROI depicted for the two methods.
Tissue velocity estimates directly impact the computation of shear wave velocity maps as recently shown by Deffieux et al (2012). The benefits in terms of standard deviation were quantified on several acquisitions. The extent of reconstructed area in the shear wave velocity map was also investigated. The local estimation of the shear wave velocity is done by using a simple time of flight algorithm (Tanter et al 2008). The time of flight Δt is estimated by a cross-correlation between the displacement time profile at location x and at location . In this experiment, Δx has been set at 1.2 mm and the same acquisition has been repeated 10 times, for the two imaging methods. The average shear velocity maps obtained on a breast phantom using this algorithm are represented in figure 6. For each pixel of the image, the standard deviation over the 10 acquisitions has been estimated. Then, the shear velocity pixel was only drawn when the corresponding standard deviation was less than 20% of the mean shear velocity, which corresponds to 0.4 m s−1 in this experiment. 'Reconstructed area' denotes the area containing the pixels that are below the threshold. The background image is the B-mode image. By using coherent plane wave compound, 42% of the image in the black rectangular box is reconstructed, whereas with multiplane wave imaging the reconstructed area increases to 59%. Once again, the multiplane wave method enables deeper imaging than the coherent plane wave compounding method, thanks to the direct impact of the SNR on the estimation of shear wave velocity (Deffieux et al 2012).
Figure 6. Shear wave imaging (elastography) on a breast phantom using 4 angles (−3 −1, 1, 3°). Comparison between coherent plane wave compound (left) and multiplane wave compound (right): average shear velocity map (N = 10 acquisitions) superimposed over B-mode image. A threshold was put on the standard deviation to select the shear velocity pixels to draw (0.4 m s−1). Using coherent plane wave compound 42% of the image in the black rectangular box is reconstructed, with multiplane wave the surface of reconstruction rises 59%.
Download figure:
Standard image High-resolution imageThe standard deviation of the shear wave velocity maps in the reconstructed area for the two sequences has been estimated using N = 10 acquisitions. Standard deviations σPlaneWave = 0.15 ± 0.09 m s−1, and σMultiplaneWave = 0.07 ± 0.05 m s−1. were measured. As expected, the multiplane wave sequence with N = 4 plane waves emission yields a halving of the shear wave velocity standard deviation in the reconstructed area. Once again, this value is consistent with the theory in the case of 4 plane waves emission since a decrease in the tissue velocity standard deviation also yields a decrease in shear wave velocity standard deviation (Deffieux et al 2012).
The multiplane wave approach leads to a strong improvement of the shear wave imaging accuracy: a noise reduction in the shear wave propagation movie induces a larger reconstruction of the shear velocity maps and a higher accuracy for the estimation of the local shear velocity.
3.3. In vivo ultrafast Doppler imaging
The multiplane wave imaging technique was applied to in vivo Ultrafast Doppler acquisitions of the living rat brain. Ultrafast Doppler imaging acquires samples simultaneously for all pixels in the image, whereas conventional Doppler successively focuses the beam along the transducer array to obtain the whole image (Bercoff et al 2011). Ultrafast Doppler acquisition relies on ultrafast frame rates, which means that typically for 1 s of acquisition, each pixel of the image contains 1000 temporal points. It has been shown that in the 16 plane waves coherent compounding case, this high frame rate enables a 30 times increase of the Doppler sensitivity compared to conventional Doppler (Mace et al 2013).
For this experiment the rat underwent a surgical thinning of the skull under anesthesia (Ketamine/Xylazine) to enable the propagation of ultrasound. It was then placed in a stereotaxic device and maintained under anesthesia (Ketamine/Medetomidine) during acquisition. The acquisitions were performed using a 15 MHz ultrasonic probe (128 elements, pitch 0.08 mm, Vermon, Tours, France) and an AixplorerTM ultrasound system (SuperSonic Imagine, Aix-en-Provence, France) running Matlab (MathWorks, Natick, Massachusetts, USA). The receive bandwidth of the scanner was again set at 90% using the on board FIR filter. The positioning was performed with a motor system controlled by Matlab. The two imaging methods were compared for N = 2, 4 and 8 plane waves tilted with angles separated by 2°, corresponding to the following sets [−1 1]°, [−3 −1 1 3]°, and [−7 −5 −3 −1 1 3 5 7]°. The Pulse Repetition Frequency was set at 1600 Hz, 3200 Hz and 6400 Hz respectively, in order to achieve 800 Hz frame rate for the final Doppler images after recombination. This frame rate is sufficient to correctly sample axial blood flow speed up to 4.1 cm s−1 without aliasing. Acquiring 200 frames in 0.4 s enables to capture 2 complete cardiac cycles. The Doppler images obtained by averaging over 200 frames are presented in figures 7(a) and (b) for the two ultrafast imaging techniques. For each set of plane waves, the multiplane wave method exhibits an improvement of the image compared to the coherent compound method: more vessels are visible, mostly in depth. The contrast-to-noise ratio (CNR) has been computed for vessels in a deep area (figure 8). The multiplane sequence leads to up to a 6 dB increase in detectability of the vessels in deep areas (around 14 mm from the probe) using N = 8 plane waves.
Figure 7. In vivo ultrafast Doppler imaging on a rat brain: comparison between (a) coherent plane wave compounding and (b) multiplane wave imaging using N = 2, 4 or 8 tilted plane waves: [−1 1]°, [−3 −1 1 3]°, and [−7 −5 −3 −1 1 3 5 7]°. Each image was normalized between its maximum value and its median background noise value ([0 1]), the dynamic range was then set between −0.1 and 1 in order to fully visualize the background noise dynamic. The four white rectangles are the areas zoomed and depicted in figure 8.
Download figure:
Standard image High-resolution imageFigure 8. Zoom in on the 4 areas depicted in figures 7(a) and (b) for N = 8 planes waves case. Comparison between coherent plane wave compounding (up) and multiplane wave imaging (bottom) for vessels around 8.5 mm and 14 mm from the probe. The contrast to noise ratio has been calculated as CNR = (vessel –
tissue) / std(PWtissue), with
vessel/tissue the mean value of the power Doppler in an area containing vessel (solid line) or tissue (dotted line), and std the standard deviation. CNR was estimated at −1.8 dB, 5.5 dB and 8.2 dB for the plane wave Doppler image (respectively for 2, 4 and 8 plane waves) and 3.9 dB, 8.5 and 14.0 dB for the multiplane wave Doppler image (respectively for 2, 4 and 8 plane waves), showing a strong increase in detectability of the vessels in deep areas thanks to the multiplane sequence.
Download figure:
Standard image High-resolution imageThis improvement could be of great interest in the particular case of deep organs Doppler imaging such as the kidney. The use of multiplane wave imaging could also be of great interest for functional ultrasound imaging to assess deep structures or to increase SNR in transcranial functional imaging. For example, deep functional areas in the rat brain such as substancia nigra, amygdala or hypothalamus usually suffer from a lack of detectability caused by their depth. Since these areas lie at a similar depth as the ROI in which we found a 6 dB increase in CNR using 8 plane waves, we can expect a better signal-to-noise ratio for the functional activity of these areas thanks to multiplane wave imaging.
4. Discussion
In this work, a new ultrasonic sequence was introduced for ultrafast imaging with improved SNR and improved contrast compared to conventional coherent compounding imaging. The sequence is based on the emission of multiple plane waves with different inclinations and different coded amplitudes within the same transmit event. A Hadamard matrix (−1, +1) was used to code the amplitude coefficients. By applying the appropriate coefficients on the received data, each data was reconstructed as if emitted by a single plane wave but with lower noise. The benefit of using an Hadamard matrix is twofold: first, it ensures the maximum amplitude level for each transmission. Second, the synthetic data recombination to retrieve the backscattered echoes for each plane wave is performed using extremely simple and robust operations (only additions and subtractions).
This sequence allows an increase of the SNR by a factor N and has successively been tested for N = 2, 4, 8, 16 and 32 plane waves. This SNR increase translates into a better image contrast, especially in deep areas of the image where the loss of contrast is mostly governed by electronic noise rather than clutter. This is particularly interesting for high noise applications such as deep organs, highly attenuating media or hypoechogeneous tissue.
This SNR increase is beneficial not only for the B-mode contrast but also for shear wave experiments as tissue velocity estimation quality is directly linked to the acquisition SNR (Deffieux et al 2012). For N = 4 emissions a halving of the tissue velocity standard deviation was observed.
A direct consequence of the SNR increase in the estimation of the tissue velocity field is a better estimation of shear wave velocity maps in shear wave elastography imaging. For N = 4 emissions, a two-fold reduction of the standard deviation of the shear wave velocity maps was observed as predicted by theory (Deffieux et al 2012). This is particularly relevant for deep organs imaging and staging, such as liver fibrosis staging or liver cancer imaging, where the estimation accuracy is critical and where SNR can be poor because of the depth of the region of interest. Increasing the acquisition SNR decreases both the shear wave velocity standard deviation and its bias.
In the case of ultrasensitive Doppler, a high number of images increases the overall sensitivity (Mace et al 2013). Increasing the SNR by using multiplane wave imaging directly brings an additional sensitivity of the Doppler image and so enables the detection of new vessels in the brain. Together with better spatiotemporal clutter rejection as demonstrated recently in (Demene et al 2015), the multiplane sequence can further increase the sensitivity of ultrasensitive Doppler sequence. This will enable a better detection of small blood flow variation for functional ultrasound imaging (Mace et al 2013) and reduce the decorrelation artefacts caused by noise in functional connectivity imaging (Osmanski et al 2014).
The multiplane wave sequence with N emissions is equivalent, SNR wise, to averaging received data N times—sometimes denoted N buffers accumulation. However, for the same SNR, the benefit of the multiplane sequence is to not lower the final frame rate, which can be critical for imaging highly moving organs such as the heart, for quantifying the fast flow with ultrafast Doppler, or for making measurements in stiff tissue where shear waves propagate very fast. For a given frame rate and a given SNR, the multiplane sequence requires less reception buffers and as a consequence reduces the amount of transferred and processed data. Both approaches can be implemented directly on the acquisition board, using very simple summations and subtractions prior to the data transfer to the main computer and beamforming.
The multiplane sequence is also equivalent in terms of SNR to a fold increase of the emitted amplitude of individual plane waves. However, increasing the emitted amplitude is not always possible due to pulser limitation, probe voltage limitation and also mechanical index (MI) limitation in some cases. For this reason, the sequence is promising for many applications where a high frame rate is needed and signal-to-noise ratio is limited. One such application is shear wave elastography of the liver where SNR is critical to obtain high accuracy of the shear wave velocity measurement for fibrosis staging and where high frame rate is required to avoid breathing artefacts. The sequence must then be adapted to a curved array for abdominal imaging. Another application requiring both high frame rate and high SNR is cardiac shear wave elastography (Papadacci et al 2014). For this purpose, the multiplane sequence can be adapted to the use of diverging waves instead of plane waves.
For an equivalent MI, the multiplane wave sequence gives a higher spatial peak temporal average Intensity (ISPTA) than conventional coherent compounding sequence. However, this ISPTA increase is not a limiting factor when the ultrafast frame rate is required during limited periods and not continuously. This is the case for shear wave elastography where ultrafast imaging is performed only during tens of milliseconds to produce a quantitative elasticity map. For such applications, the Food and Drug Administration (FDA) requirements for ISPTA limits the number of ultrafast bursts performed in one second. When the ISPTA is the limiting factor, the only advantage of the multiplane sequence is its higher frame rate.
For a given SNR, the multiplane sequence allows the use of lower pressure; as a result, it could be beneficial for applications where the MI is limited and where high frame rate is required, such as in ultrafast contrast imaging techniques relying on time-dependent microbubbles echoes in the fundamental mode (Couture et al 2009). It could also be useful for cornea or retina Doppler imaging or shear wave elastography for which FDA requirements limits the mechanical index to 0.23.
Compared to coded emissions such as chirp or Golay codes (Chiao and Thomas 2000, Misaridis and Jensen 2005a, 2005b, 2005c), the multiplane sequence enables higher frame rate with lower complexity. The coding is here performed both in space and time and the decoding operation is only made of extremely simple operations. On the contrary, chirp excitations require a more complex deconvolution process whose efficiency can be altered by the medium attenuation.
Contrarily to chirp excitations, no a priori on the insonified medium is required with multiplane wave, even though linearity of the propagation must be enforced. Indeed, in this paper, we assume that the cancellation (+1) + (−1) = 0 is perfect, but non-linear propagation generates harmonic signals, that, if not filtered, will not cancel out in the combination step and will create range lobes, and ultimately reduce the image contrast. In our implementation, several steps have been made to filter out harmonic signals in the received data: emissions were made at the central frequency of the probes (6 and 15 MHz) and a 90% FIR bandwidth filter was applied at the reception. In such a configuration, the multiplane method is likely to work even in the presence of non-linearity.
Another possible limitation of the technique is fast motion, such as cardiac B-mode imaging where the cancellation of some waves might not be perfect due to phase coherence losses (Denarie et al 2013). In that case it might not be possible to use a large number of plane wave transmissions without additional motion correction steps. For a given number of plane waves and a given tissue velocity, the exact outcome of contrast decrease due to fast motion and of contrast enhancement due to lower noise is yet to be investigated.
The multiplane sequence relies on the amplitude encoding of plane waves instead of individual elements as in synthetic aperture. In this work, an Hadamard matrix was chosen and the different plane waves were encoded in amplitude (−1 or +1) with a small pause between them to avoid the superimposition of plane waves in the emission matrix. Superimposition of plane waves would allow shorter emission matrix and a reduction of the blind zone in the beginning of the image. However, the individual plane wave amplitudes would then have to be decreased to ensure that the superimposition amplitude remains below the maximum output amplitude of the scanner. Moreover, multiplane imaging will be mainly useful for deep imaging where a larger blind area caused by a long emission matrix is not a problem. However, it could be interesting to reduce the blind area by using more compact emissions matrix with appropriate plane waves superimposition in the particular case of high frequency imaging where attenuation can decrease the signal-to-noise ratio and where the probe driving voltage is generally much lower than the scanner maximum output.
5. Conclusion
In this work, a new ultrafast ultrasound imaging sequence based on multiple plane wave emission was proposed and validated. Multiplane wave imaging is a promising mode for ultrafast ultrasound imaging that allows the same high frame rates as coherent plane wave compounding, while significantly improving signal-to-noise ratio, without affecting spatial resolution. The improved signal-to-noise ratio directly impacts the contrast of B-mode images for deep structures where the electronic noise is predominant over clutter. It further significantly improves both the reconstruction and accuracy of shear wave velocity measurements and the overall quality of Power Doppler images. Multiplane wave imaging has the potential to further disrupt ultrafast based imaging modalities in many clinical applications especially for deep organs imaging.
Acknowledgments
The research leading to these results has received the funding from the European Research Council under the European Union's Seventh Framework Programme (FP7/2007–2013) / ERC grant agreement no 339244-FUSIMAGINE. This work was also partly supported by the Agence Nationale de la Recherche under the program 'Future Investments' with the reference Laboratory of Excellence ANR-10-LABX-24 LABEX WIFI within the French Program 'Investments for the Future' under reference ANR-10- IDEX-0001-02 PSL.