Abstract
Optical chiral metamaterials have recently attracted considerable attention because they offer new and exciting opportunities for fundamental research and practical applications. Through pragmatic designs, the chiroptical response of chiral metamaterials can be several orders of magnitude higher than that of natural chiral materials. Meanwhile, the local chiral fields can be enhanced by plasmonic resonances to drive a wide range of physical and chemical processes in both linear and nonlinear regimes. In this review, we will discuss the fundamental principles of chiral metamaterials, various optical chiral metamaterials realized by different nanofabrication approaches, and the applications and future prospects of this emerging field.
Export citation and abstract BibTeX RIS
1. Introduction
Chirality refers to the geometric property of a structure lacking any mirror symmetry plane. It exists in many forms in nature, ranging from molecules, to proteins, and to crystals. In contrast, a structure is achiral if it is indistinguishable or superimposable on its mirror image. Chiral geometries can produce intriguing optical effects. For example, optical activity or optical rotation is the rotation of polarization of linearly polarized light as it travels through a chiral material, while circular dichroism is the differential absorption of left-handed polarized (LCP) and right-handed polarized (RCP) light. Optical activity was first discovered by Arago as sunlight passing through a quartz crystal placed between two crossed polarizers [1]. The optical rotation in quartz originates from the low symmetry of its crystalline structure and highly depends on the wavelength of light. Shortly after Arago's discovery, optical activity of molecules was observed in turpentine (by Biot), and subsequently in solutions of camphor and sugar [1]. Chirality also widely exists in biological species. For example, gyroid nanostructures produce the vivid colors of butterfly wings [2], and chiral microstructure patterns make Chrysina gloriosa (jeweled beetles) appear more brilliant under LCP illumination than the case under RCP illumination [3]. Chirality is of great importance in the study of chemistry and biology because molecules with different spatial configurations can lead to distinctly different physiological responses. However, the optical activity of a natural material is generally very weak, and therefore the chiral effect is detectable only when the optical path length is much larger than the wavelength of light.
Metamaterials are artificial composite materials with exotic properties, which have attracted the intensive interest of physicists, material scientists, chemists and engineers over the past few decades. Metamaterials comprise of periodically or randomly distributed artificial structures with size and spacing much smaller than the wavelength of interest [4–7]. Using different metamaterial designs, researchers have been able to engineer material properties with unprecedented degrees of freedom and demonstrate extremely low-frequency plasmons [8], artificial magnetism [9] and negative refractive indices [10]. One early research focus in metamaterials is to realize negative refractive indices, which promise numerous applications such as super-resolution imaging [11–13], invisibility cloaking [14–18] and illusion optics [19]. Negative permittivity and permeability can be simultaneously achieved in the same frequency band by properly combining electric and magnetic resonators, which gives rise to negative refractive indices. This concept was initially verified at microwave frequencies and then extended deeply into the visible regime [20–24].
As a subset of metamaterials, chiral metamaterials exhibit a number of intriguing properties. For example, if the chirality is sufficiently strong, negative refractive indices can be realized even though neither ε nor μ is negative. Such a chiral route towards negative refractive indices was theoretically proposed by Tretyakov et al [25], and later independently discussed by Pendry [26] and Monzon et al [27]. This concept was then experimentally demonstrated in the microwave [28–30], terahertz [31] and optical regimes [32, 33]. In addition, chiral metamaterials have attracted much interest because of their extremely strong optical activity [29, 31, 34–37], which promises the ability to create compact broadband circular polarizers [38] and asymmetric transmission [39–42]. Moreover, superchiral electromagnetic fields in chiral plasmonic nanostructures are expected to substantially enhance the interaction between light and chiral molecules by several orders of magnitude [43], which may open a new avenue for chiroptical detection with unprecedented sensitivity.
The rest of the review is organized as follows. We will first discuss in section 2 the symmetry group of chiral metamaterials and its effect on the form of the scattering matrix, followed by the review of optical chiral metamaterials categorized by different nanofabrication approaches in section 3. In section 4, we will survey two aspects of chiroptical effects: extrinsic chirality and the superchiral field. Section 5 will discuss the potential applications of chiral metamaterials, including nonlinear optics, enhanced light–matter interactions and active chiral metamaterials. Finally we will provide a conclusion and a brief perspective on the future development of chiral metamaterials.
2. Chirality parameters and symmetry considerations
As we have discussed, a material is defined as chiral if it lacks any plane of mirror symmetry. In electromagnetics, a chiral medium is a subset of bianisotropic media in which the electrical and magnetic fields are coupled together. For a general chiral medium, the optical response is usually described by the constitutive relations:


where
and
are the permittivity, permeability and chirality tensors, respectively. ε0 and μ0 are the permittivity and permeability of vacuum.
is the electric field,
is the magnetic field,
is the electric displacement and
is the magnetic induction. For isotropic chiral materials, the constitutive parameters could be simplified to scalars (ε, μ and χ). The refractive indices for the right- (+) and left-handed (−) circular polarizations are different, and are given by

Equation (3) shows that waves of opposite handedness will obtain different accumulations of phase as they travel through a chiral material. However, the two circular polarizations have the identical impedance of where
is the impedance of vacuum.
A linearly polarized wave can be described as the superposition of an LCP and an RCP wave with identical amplitude. Starting from equation (3), one can readily prove that the refractive index difference between the two circularly polarized waves leads to the rotation of the linear polarization by an angle where d is the thickness of the chiral medium and λ0 is the wavelength of light in vacuum. This is the physical implication and mechanism of optical rotation. Alternatively, the rotation angle can be written as
where T+ and T− are the transmission coefficients for the two spin states. For an absorbing chiral medium, the imaginary parts of the refractive index for RCP and LCP light are different, indicating that RCP and LCP light have different attenuation and therefore circular dichroism (CD) occurs. From equation (3) one can also see that if the chirality χ is strong enough, a negative refractive index may exist for one circularly polarized light even when both ε and μ are positive. This is the chiral route to negative refraction as originally proposed by Tretyakov et al [25], Pendry [26], and Monzon and Forester [27], and experimentally demonstrated by several groups [28, 31, 32].
The properties of metamaterials are frequently described in terms of effective material parameters, which can be obtained from scattering parameters by the retrieval method [44–46]. However, such an approach requires the tensor format of the complex constitutive parameters to be determined in advance. This feature dramatically increases the complexity in analyzing sophisticated optical effects such as anisotropy, nonlocality and spatial dispersion [47, 48]. As a result, a more suitable means for describing the properties of metamaterials is the optical response itself. For planar metamaterials, the optical responses can be easily described by Jones matrices, which relate the complex amplitudes of the incident and the scattered fields [49, 50].


Here R and T are called reflection and transmission matrices for linear polarization.
and
are the incident, reflected and transmitted electric fields polarized along the x direction, respectively. The similar notations with a superscript of y represent the fields polarized along the y direction. By changing the Cartesian base to a circular base, the Jones matrices for the two states of circular polarization are obtained [50]
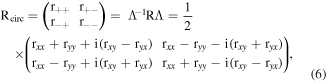
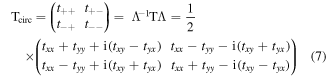
where is the change of basis matrix, and the subscript +/− denotes clockwise/counterclockwise circularly polarized waves as viewed along the +z direction, respectively.
Symmetry consideration is an efficient method for predicting how the symmetry of a structure affects the characteristics of the Jones matrices and ultimately the optical properties of the structure. Either rotation or reversion of a structure is accomplished mathematically by applying particular matrix operations. If a metamaterial exhibits a certain symmetry group, the Jones matrices after transformation must be identical to the original ones. We will briefly discuss in the following how mirror and rotational symmetries affect the reflection and transmission matrices of a chiral slab.
The mirror symmetry with respect to the incident plane (defined as the xz-plane) results in the absence of the off-diagonal elements in the Jones matrices of linear polarizations and thus the Jones matrices for circular polarizations become symmetric (
[50]. Since the optical activity is usually characterized by
there is no polarization rotation due to chirality. The off-diagonal elements are also identical so that two circularly polarized waves have the same efficiency of polarization conversion. Therefore, neither optical activity nor circular dichroism can exist in mirror-symmetric structures. This result explains, from the point view of Jones matrix, why chirality only exists in structures that lack mirror symmetries.
In the cases of three-fold (C3) or four-fold (C4) rotational symmetries, the symmetry consideration leads to and
[50]. For circular polarizations, we therefore obtain
The same reasoning holds true for the transmission matrix so that
In the special case of normal incidence, the De Hoop reciprocity states that the reflection matrix obeys the general identity R = RT, where the superscript T represents the transpose operation [51–53]. If we combine these restrictions together, the linear polarization conversions are proved to be zero
Consequently, the Jones matrices can be written as
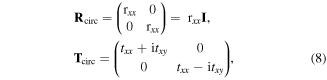
where I is the identity matrix. Therefore, the reflection coefficient is the same for all polarizations, which can also be predicted by the effective medium method. For a structure with C3 or C4 symmetry, the expression of the chirality parameter can be simplified to a scalar χ, which leads to the absence of polarization conversions [46, 54, 55]. Calculation in the transmission problem of a chiral slab also reveals that the reflection is identical for both LCP and RCP illuminations in an isotropic chiral material [36]. The underlying reason is that the impedance of such a chiral medium is only determined by the ratio between its permittivity and permeability, and is independent of the chirality parameter. Furthermore, when losses are absent, analyses in reciprocity and energy conservation dictate that the transmission coefficients of the two spin states are also identical [53].
If the chiral metamaterials become anisotropic, such as the two-fold (C2) rotational symmetry, the polarization conversion between the two spin states in reflection would appear. It is important to note that two-dimensional (2D) planar structures cannot possess structural chirality in a three-dimensional (3D) space. This is because of the equality of the off-diagonal elements in Jones matrices that originated from the in-plane mirror symmetry, which results in However, the dichroism in the total transmission of two spin states is still available in well-designed structures, which show unequal efficiencies in polarization conversions
[56]. Such a phenomenon is also termed as asymmetric transmission in reciprocal materials [42, 57]. In addition, metamaterial structures without any rotational symmetries can also exhibit strong chiral responses. For example, twisted-arc structures have been proposed to achieve giant circular dichroism in both microwave [58, 59] and optical frequencies [60, 61]. In these cases, the forms of the Jones matrices can hardly be specified from symmetry considerations and it would be more convenient to analyze the chiroptical responses by the induced current distribution.
Metasurfaces provide a distinct way to manipulate the flow of circularly polarized light [62]. Different from the traditional metamaterials, metasurfaces control the optical responses of circularly polarized light by spatially modulating the phase responses of achiral elements rather than designing chiral metamolecules. A variety of exotic optical phenomena based on this technology have been realized, such as dispersionless abnormal reflection and refraction [63], optical vortex generation [64], optical activity [65], all-dielectric focusing [66], achromatic generation of optical angular momentum [67] and high-efficiency holograms [68].
3. Fabrication of optical chiral metamaterials
Optical chiral metamaterials, which consist of building blocks with feature sizes on the nanoscale level, can generally be fabricated by the top-down and bottom-up approaches. The top-down approach uses various techniques, such as ion beam lithography, electron beam lithography and direct laser writing, to cut, mill and shape materials into the desired shape and order. The bottom-up approach, in contrast, usually seeks to arrange small components into more complex assemblies. Both of these two fabrication approaches are widely employed to fabricate optical chiral metamaterials as discussed in the following.
3.1. Optical chiral metamaterials fabricated by top-down methods
Chirality is inherently a 3D structural property. One classical example of chiral metamatrials is a 3D helix structure (figure 1). In [38], a uniaxial photonic metamaterial composed of 3D gold helices (figure 1(a)) was investigated as a broadband circular polarizer in the mid-infrared regime. These nanostructures are fabricated by direct laser writing of helical pores followed by electrochemical deposition of gold. To fabricate an array of helical pores, a positive-tone photoresist is selected and spun onto a glass substrate. Only those regions that are sufficiently exposed by light are removed. Deposition of gold is then controlled by the applied current density and the growth time. Plasma etching is finally used to remove the polymer and realize a square array of 3D gold helices on a glass substrate. The measured transmission spectra in the wavelength region from 3.5 to 7.6 μm reveal the anticipated functionality of blocking one direction of circularly polarized light, while transmitting the other circular polarization (figure 1(a)). N-helical [52] and tapered chiral metamaterials [69] have also been designed to reduce the polarization conversion and broaden the operation bandwidth. However, this approach turns out to be impractical for bichiral structures, which consist of both left-handed and right-handed spirals arranged along three orthogonal spatial axes [70]. This is because certain parts of the horizontal helical cavities are cut off from the electrolyte before they can be completely filled, leaving behind gaps in the final structures. Radke et al have fabricated bichiral plasmonic crystals by combining direct laser writing with electroless silver plating (figure 1(b)) [71]. First, two-photon femtosecond direct laser writing in a negative-tone photoresist fabricates a 3D bichiral crystal. Next, the dielectric template is coated with a conformal silver film via electroless plating. Electroless plating does not require any external current source and possesses the advantage of coating all exposed surfaces. This feature is very challenging to achieve with standard deposition methods, such as vacuum evaporation and sputter coating, because of the self-shadowing of the complex 3D structures.
Figure 1. 3D chiral metamaterials fabricated by top-down methods. (a) Fabrication process of 3D helices by direct laser writing and electrochemical deposition of gold (left panel) and the transmission spectra in the mid-infrared region for structures with opposite handedness (right panel). (b) SEM image of a bichiral crystal with right-handed corners and left-handed helices after electroless silver plating. (c) Glancing-angle deposition of nanohelices and the CD spectra. Inset: transmission electron microscopy (TEM) images of grown structures with left (top) and right (bottom) chirality. (d) Atomic force microscopy (AFM) image of 3D spirals fabricated by colloidal hole-mask lithography. (e) SEM image of 3D L-shape nanostructures fabricated by on-edge lithography. (f) SEM image and CD spectra of triple-helical nanowires fabricated by tomography rotatory growth, on the basis of FIBID. (a) is adapted from [38], (b) is adapted from [71], (c) is adapted from [74], (d) is adapted from [77], (e) is adapted from [78] and (f) is adapted from [80] with permissions.
Download figure:
Standard image High-resolution imageGlancing-angle deposition is another approach for fabricating 3D chiral plasmonic nanostructures [72–75]. Its working principle is based on the geometric shadow effect that materials do not deposit in the shadow created by existing structures. Plasmonic nanohelices have been fabricated via the technique of low-temperature shadow deposition [74, 76]. Through precise control over the nanoseed pattern, substrate and temperature, nanohelices of opposite handedness have been successfully fabricated. The measured circular dichroism spectra of the nanohelices in sodium citrate solution show a peak for LCP light and a dip for RCP light around 600 nm (figure 1(c)). Chiral gold nanoshells have also been made by vacuum evaporation on achiral nanopillars with tilted deposition angles [72]. Large-area 3D gold spirals have also been manufactured by colloidal hole-mask lithography in combination with tilted-angle rotation evaporation (figure 1(d)) [77]. Control over both the rotating speed and direction of the samples can flexibly switch the handedness and working frequency of chiral enantiomers. Circular dichroism signals up to 13% have been measured in the 100–400 THz frequency range. This method offers a simple low-cost manufacturing method for cm2-sized chiral plasmonic metamaterials. Another method for fabricating 3D chiral materials is on-edge lithography [78]. In this approach, a template with a corrugated profile is first generated. Then, a thin chromium layer is deposited by physical vapor deposition, followed by spin coating of a layer of electron beam resist. The resist is subsequently exposed and the resulting mask is evaporated with a layer of gold. The 3D L-shape is finally obtained after lift-off (figure 1(e)). This fabrication approach offers an accurate method for generating 3D chiral nanostructures within a single lithography step.
Focused ion beam induced deposition (FIBID) has recently been employed for realizing broadband chiral metamaterials in the near-infrared region [79]. Platinum nanospirals are formed by FIBID and scanning electron microscopy (SEM) in a dual configuration system. Precursor metal–organic compounds are decomposed by an ion beam and absorbed onto the substrate. The handedness of nanospirals is controlled via sweeping the ion beam in a clockwise or counterclockwise direction. Surface charge effects on different substrates can influence the growth and evolution of nanostructures and induce a dimensional gradient of the wire diameter of the spiral structure. Multiple-helical structures have been created using tomographic rotatory growth, which combines the basic principles of tomography with the FIBID technique (figure 1(f)) [80]. Rotational symmetry is ensured by a packed multi-helix arrangement in a single cell, which eliminates cross-polarization conversion from anisotropy. These triple-helical nanowires show up to 37% of circular dichroism in a broad range from 500–1000 nm.
Focused electron beam induced deposition (FEBID) is also a suitable technique for the realization of three-dimensional nanostructures for optical applications [81–83]. Nanoscaling of chiral structures has been achieved by comparing the FIBID and FEBID approaches [84]. Some physical factors affecting the fabrication processes, including resolution limits, growth control and 3D proximity effects, have been carefully analyzed.
Stacked-planar structures are an alternative approach to accomplishing strong chiroptical responses. In general, such structures can be fabricated by standard electro-beam lithography along with alignment in a layer-by-layer manner. Figure 2(a) shows a chiral structure of twisted split rings [85]. The transmittance spectrum can be tuned by adjusting the twisted angle. Specifically, when the angle is 90°, the electric fields in the gaps of the two split rings are perpendicular to each other and thereby no electric dipole–dipole interaction occurs. In addition, as the higher-order multipolar interaction is negligible in a first approximation, the electric coupling can be ignored. Therefore, the resonances are determined by magnetic dipole–dipole coupling. For the 90° twisted dimer, the magnetic dipoles in the two split rings at the two resonances ( and
are aligned parallel and antiparallel, respectively, and thus produce chiroptical responses. This strategy is similar to the concept of stereoisomers in chemistry in that isomers have identical chemical formula but different spatial arrangements of atoms in a molecule. It is known that the electronic properties of a molecule are generally influenced by its composite as well as its configuration. These properties determine how the electronic wave functions mix and hybridize, as well as the interaction and binding between the atoms in the molecule [86]. Similarly, a cluster composed of several nanoparticles provides the possibility of configuring the hybridization of plasmonic resonances, thus enhancing the overall chiroptical responses in plasmonic oligomers (figure 2(b)) [87–89]. Diverse chiral nanostructures have been proposed based on this methodology, including bilayer L-shapes (figure 2(c)), arcs (figure 2(d)) and so on [32, 60, 90–94].
Figure 2. Stacked-planar chiral metamaterials. (a) Schematic, field-emission electron microscopy and transmission spectra of a twisted gold split-ring dimer metamaterial. (b) CD spectra and SEM images of three chiral oligomers. (c) L-shape chiral nanostructure. (d) Twisted-arc chiral metamaterials and the corresponding transmission spectra. (e) Multilayer twisted metamaterial as a broadband circular polarizer. (f) Schematic and SEM images of chiral metamaterials fabricated by depositing gold film on structured polycarbonate substrates. (a) is adapted from [85], (b) is adapted from [87], (c) is adapted from [90], (d) is adapted from [60], (e) is adapted from [95] and (f) is adapted from [96] with permissions.
Download figure:
Standard image High-resolution imageBroadband circular dichroism can also be achieved by cascading multilayer identical nanorod metasurfaces with a relative rotational twist [95]. As shown in the schematic of figure 2(e), each layer of nanorod is intrinsically achiral. Nevertheless, the chiroptical response emerges when neighboring layers are stacked in a helical-like fashion. The transmission spectra of the four-layer twisted structure demonstrate a broadband circular dichroism in the visible regime. Some constraints can be relaxed in such planar, twisted chiral structures, including the complexity of shape requirements and the lateral alignment between neighboring layers. Moreover, a disposable plasmonic chirality has been recently proposed, where a metafilm is grown on a nanoindented polycarbonate substrate fabricated by high-throughput injected molding [96]. The generated structure consists of a solid nanostructure and an identical shaped void directly above it (figure 2(f)). Babinet's principle implies that the switch of electric and magnetic fields between the solid and inverse structure gives rise to the chiroptical response. The optical properties can be tuned by changing the film thickness. This method offers a cost-effective and flexible way of manipulating the chirality in plasmonic structures.
3.2. Self-assembled chiral nanostructures
While top-down fabrication technologies are capable of precisely making nanostructures with a feature size of or below 100 nm, they are normally non-scalable, time-consuming and expensive. By contrast, self-assembly technology provides an alternative bottom-up approach for realizing structures at the truly nanometer scale yet in a cost-efficient, highly-tunable and fast manner. Over the past few years, self-assembled chiral nanostructures have attracted extensive interest and shown significant progress.
Self-assembly technology takes advantage of the fundamental forces of nature to synthesize building blocks into multi-atom functional systems. Objects at the nanometer scale can be organized into equilibrium structures due to the delicate balance among various forces [97, 98], which include Van der Waals forces, capillary forces, static and/or transient electromagnetic forces, convective forces, friction forces, and so on. Based on such self-assembly methods, various metallic nanoparticles with different chemical compositions, geometries and sizes can be precisely positioned and controlled. As a result, plasmonic structures with tunable and controllable dimensions can be assembled in a programmable manner with nanometer precision.
The DNA base-pair interaction is widely used for the synthesis of plasmonic chiral nanostructures. DNA-based formation of silver nanoparticles with CD properties has been demonstrated in some early work [99, 100]. Metallic nanoparticles can also be assembled into pyramid geometries with DNA to enhance the chiral responses [101, 102]. In [101], Mastroianni et al demonstrated chiral pyramidal nanostructures, in which DNA strands function as a scaffold for controlling the position of gold nanoparticles with different diameters. The TEM images of synthesized chiral pyramids are shown in figure 3(a). This work provides a way of creating artificial multi-atom molecules with controllable optical properties. The building blocks in such multi-atom molecules are not limited to gold nanocrystals. It is possible to link other metallic and semiconductor particles via this method. Theoretical modeling for such pyramid structures has been performed [103, 104]. The pyramidal structure consists of two pairs of nanoparticles with different sizes. The intensity, spectral characteristics and handedness of the CD response can be tuned by changing the separation along the symmetry-breaking arm. Simulations predict significant CD tunability when the length of the symmetry-breaking arm is varied [104].
Figure 3. Plasmonic chiral nanostructures based on a self-assembly method. (a) Illustration of chiral pyramids of gold nanocrystals obtained with DNA scaffolds and TEM images. (b) Four gold nanoparticles assembled with bifacial DNA template in left-handed, right-handed and achiral structures and the corresponding CD spectra of these structures. (c) Illustration of synthesizing a 3D gold-particle helix by rolling a planar DNA template into a 3D DNA origami tube. (d) Schematic illustration of DNA-guided self-assembly of plasmonic nanohelices with both left and right handedness and the corresponding CD signals. (e) Double-helix nanoparticle superstructures by peptide-based assembly platforms. 3D surface renderings of the tomographic volumes show the left-handed or right-handed structure of double helices. (f) Schematic of chiral plasmonic nanostructures assembled with gold nanorods and cellulose nanocrystals. (g) Enhanced chiral dichroism after binding gold nanoparticles with peptide molecules that have chiral properties. (a) is adapted from [101], (b) is adapted from [106], (c) is adapted from [112], (d) is adapted from [113], (e) is adapted from [119], (f) is adapted from [120] and (g) is adapted from [134] with permissions.
Download figure:
Standard image High-resolution imageBifacial DNA origami templates can also be used to fabricate chiral nanostructures with metallic nanoparticles [105–107]. For example, it has been reported that bifacial DNA origami templates can be used to assemble 3D chiral metamaterials with four gold nanoparticles [106]. The experimental schema is shown in figure 3(b). The rectangular DNA origami template is predesigned with three binding sites on the top surface and the fourth site on the bottom surface, right below one of the three top binding sites. Left-handed and right-handed structures can be obtained by setting the fourth binding sites in the left-handed or right-handed geometry. A characteristic bisignate CD spectrum is observed for left-handed and right-handed chiral nanostructures, compared with achiral nanostructures.
The idea of folding DNA strands to create artificial nanostructures [108–110] has stimulated the rapid development of plasmonic chiral metamaterials [111–114]. One experimental study of DNA-based chiral metamaterial is shown in figure 3(c) [112]. The gold nanoparticles coated with special DNA strands are able to bond with complementary DNA strands on the rectangular template at predesigned positions. Upon the use of folding strands, the rectangular DNA template will roll up and eventually become a 3D DNA origami tube. This DNA 'origami' method enables the synthesis of both left-handed and right-handed metamaterials [113], which exhibit pronounced CD responses as shown in figure 3(d). As expected, the signal of the left-handed chiral plasmonic metamaterials and the signal of the right-handed ones are complementary to each other. The CD spectrum can be controlled by depositing additional silver or silver–gold alloy shells on the gold nanoparticles. Since silver has a plasmonic resonance at a shorter wavelength, a blue shift of the CD resonance can be achieved when changing the metal composition.
Assembling metallic nanoparticles with other chiral templates has also been widely studied, such chiral templates are organogel [115], cysteine [116], peptide [117–119], cellulose nanocrystals [120–123], cholesteric liquid crystals [124–128], chiral mesoporous silica [129, 130], supramolecular fibers [131] and block copolymer templates [132, 133]. For example, one work demonstrated the self-assembly of cysteine (CYS) and gold nanorods [116]. Opposite CD responses were observed for L- and D-CYS assembled gold nanorods, while no chiral responses were observed for DL-CYS. The CD responses can be manipulated from visible to near-infrared wavelengths by changing the aspect ratios of the assembled CYS and gold nanorods. Plasmonic chiral nanostructures can be also synthesized through a peptide-based methodology [119]. As shown in figure 3(e), mixing a gold nanoparticle precursor solution and a HEPES buffer with C12–L-PEPau or C12–D-PEPau will form left-handed or right-handed double-helix structures, respectively, which produce strong CD responses. The CD signal can be controlled by varying the thickness of the silver coating. In another work [120], plasmonic chiral metamaterials with cellulose nanocrystals have been assembled. The cellulose nanocrystals are rod-like nanoparticles that were self-assembled into cholesteric liquid crystalline phases. The nanoparticles were highly crystalline and negatively charged with high concentration in suspensions. Chiral plasmonic films were prepared by incorporating gold nanorods in self-assembled cellulose nanocrystals that have chiral arrangements, as shown in figure 3(f). A blue shift in the CD spectrum was observed as the concentration of nanorods increases. The chiral optical activity can be tuned by changing nanorod dimensions and the helical pitch of the cellulose nanostructure. The helical pitch can be tuned by adding NaCl to the nanorod-cellulose nanocrystal suspensions.
Last but not least, plasmonic chiral nanostructures can be realized by binding chiral molecules with metallic nanoparticles [134–137]. In one study [136], plasmonic enhancement of the CD spectrum was demonstrated through the attachment of l-GS-bimane chromophore to the surfaces of the silver nanoparticles. The two orders of enhancement in magnitude made it possible to observe a very weak induced CD signal of the bimane chromophore [136]. It was also found that chiral dichroism can be induced by binding peptide molecules on gold nanoparticles [134], as shown in figure 3(g). Two types of hybrid structures have been studied. One is E5–AuNPs, in which E5 is a 38 amino acid helical peptide that links with the gold nanoparticles through a thiol linkage. The other is FlgA3–AuNPs, where the FlgA3 peptide is an unstructured random coil peptide that binds gold nanoparticles via noncovalent interactions. The chiral responses of two kinds of hybrid structures have been observed.
4. Chiroptical effects
4.1. Extrinsic chirality
It is worth noting that optical activity can be observed even when the structures are intrinsically achiral in geometry (figure 4). Such extrinsic chirality arises from the mutual orientation of the achiral metamaterials and the incident beam. This mechanism was detected in liquid crystals in the past [138], but only revisited recently in achiral metamaterials [139]. As shown in figure 4(a), using asymmetric split rings, researchers have observed strong circular dichroism and birefringence indistinguishable from those of chiral 3D materials. The underlying mechanism is the simultaneously induced electric and magnetic dipoles that are parallel to each other. The oblique incidence is crucial for observing extrinsic chirality, because it breaks the mirror symmetry for any plane containing the wave vector (propagating direction). Extrinsic chirality can also induce a large circular dichroism in individual single-walled carbon nanotubes, with the degree of polarization reaching 65% [140]. Moreover, electromagnetic chirality can occur in 1D patterned composites whose components are achiral [141]. A schematic diagram is given in figure 4(b). Electromagnetic waves propagate through a 1D metamaterial whose unit cell is obtained by stacking layers of different media of different thickness along the x-axis. In the epsilon-near-zero regime, the nonlocal effect can be comparable or even greater than the local linear part of the dielectric response and results in enhanced chiroptical responses.
Figure 4. Extrinsic chirality. (a) Planar metamaterials based on an array of asymmetrically split rings manifest optical activity and circular dichroism at oblique incidence of light. (b) One-dimensional multilayer metamaterials show chirality in the epsilon-near-zero regime. The bottom panel depicts the optical activity of such a slab. (c) A randomly arranged anisotropic metasurface exhibits a near-complete photon spin selectivity under evanescent excitations. The left panels show the in-plane electric field amplitudes. The middle panel depicts the reflection spectra. The right panels are the AFM and SEM images of the elongated gold nanoparticles. (a) is adapted from [139], (b) is adapted from [141], and (c) is adapted from [142] with permissions.
Download figure:
Standard image High-resolution imageExtrinsic chirality in anisotropic plasmonic metasurfaces can be greatly boosted by interaction with evanescent excitations [142]. Figure 4(c) schematically illustrates the process in which the incoming light of opposite handedness impinges onto an array of gold nanoparticles. When illuminated slightly above the critical angle, no transmission is allowed. For incident wavelengths close to the plasmonic resonance of the nanoparticles, the metasurface can efficiently absorb the LCP light while the other spin state is almost totally reflected. The effect of photon spin selectivity originates from the mutual orientation of the achiral metasurface, so-called extrinsic chirality, and highly depends on both the incident angle and the relative orientation of the nanoparticle. Almost perfect selectivity (∼90%) of the incident photon spin has been observed at visible frequencies. Since polarization manipulation has intriguing applications in photonics, the enhanced chiroptical effects from evanescent waves may pave a new way for efficient on-chip chiroptical devices.
4.2. Superchiral fields
Molecules of opposite chirality, called enantiomers, always show identities in almost all physical properties (e.g., density, weight, electronic and vibrational frequencies). Opposite enantiomers become distinguishable only when they interact with other chiral objects, such as circularly polarized light. Therefore chirality-sensitive spectroscopic techniques, including circular dichroism, optical rotatory dispersion and Raman optical activity, are widely used in biomolecular science [1, 143]. However, the inherently weak chiroptical effect of molecules imposes significant challenges in biomolecular detection, especially when the concentration of molecules is low. In recent years, chiral plasmonic metamaterials have aroused a lot of interest because their chiroptical effects can be several orders of magnitude higher than those of common biomolecules [34, 144]. Furthermore, chiral plasmonic metamaterials can efficiently enhance the optical signals from biomolecules [145–148]. This is because of the enhancement of the chiral field in the near-field region, known as the superchiral field, which may provide a method for detecting the handedness of a single molecule.
The optical chirality C, a time-even pseudoscalar, is used to quantify the enhancement of this light–matter interaction [149–151]
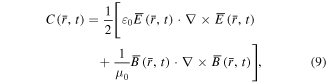
where and
are the time-dependent electric and magnetic fields, respectively. Although the quantity of chirality C was introduced in 1964, the physical meaning was disclosed very recently [151]. It has been demonstrated that this quantity is closely related to the rate of excitation of a chiral molecule. If one can increase the chirality C at the location of chiral molecules, the sensitivity for the detection of the handedness can also be enhanced [151, 152]. For time-harmonic fields, chirality C can be written as a simpler version [43]

Since the maximum optical chirality in free space is obtained for circularly polarized light where ω is the angular frequency and c is the velocity of light in vacuum, the relatively local enhancement of chirality can be calculated by
The positive and negative signs correspond to the right-handed and left-handed circular polarizations defined from the point of view of the source, respectively.
Recent work has shown that plasmonic nanostructures, whose geometry directly influences the distributions of their near fields, can be utilized to generate chiral fields with strong optical chirality. Interestingly, this strong optical chirality exists not only in 2D and 3D chiral structures under circularly polarized illuminations [43], but also in achiral structures illuminated by linearly polarized light [153–155]. For example, figure 5(a) shows the optical chirality of a left-handed helix with left-handed circularly polarized light. The absolute values of optical chirality are much smaller for the nonmatching configurations, in which the same structure is illuminated with the opposite spin state. Planar gammadion also exhibits a similar behavior for circularly polarized light, as illustrated in figure 5(b). However, the gammadion shows both positive and negative optical chirality with similar strength, while for the helix the values corresponding to the nonmatching configuration are much smaller. Moreover, in gammadion structures, regions with enhanced optical chirality are at the same position and have same signs for both spin states. Therefore, a change of incident polarization cannot provide a large change in the optical chirality at a certain position, which makes this design less practical in applications. Interestingly, the chiral near fields can also be formed in achiral structures [153, 154]. For example, optical chirality occurs in the near field of nanoslit pairs illuminated with linear polarized light (figure 5(c)) [153].
Figure 5. Superchiral field. Optical chirality enhancement for (a) a left-handed helix and (b) a planar gammadion with left-handed circularly polarized light. (c) Local electromagnetic chirality in the center of nanoslit pairs, 1 nm above the transmission interface of the array. Solid and dashed lines represent the two enantiomers. (d)–(f) Superchiral field-based biosensing. (d) CD spectra of left-handed/right-handed planar chiral metamaterials. (e) The averaged resonance shift (left) and difference in resonance shifts (right) between right-handed and left-handed planar chiral metamaterials on adsorption of chiral biomolecular layers. (f) Hemoglobin (top) and β-lactoglobulin (bottom) molecules adopt different geometries upon adsorption due to their secondary structures (α-helix, cyan cylinders; β-sheet, ribbons). (a) and (b) are adapted from [43], (c) is adapted from [153], and (d)–(f) are adapted from [145] with permissions.
Download figure:
Standard image High-resolution imageAn experimental study of superchiral fields for biosensing was reported in [145], which employed gold gammadion arrays working at visible and near-infrared frequencies. As shown in figure 5(d), three resonant modes exist in this planar chiral metamaterial. The spectra of the left-handed and right-handed gammadions are mirror images of each other, as expected. By coupling the incident light into localized surface plasmon resonances of the gold nanostructures, the local chiral field is greatly enhanced and thereby molecules adsorbed on the surface of the chiral metamaterials will strongly influence the resonant wavelength. The property of resonance shifts is caused by the change in the refractive index, which is determined by the chirality of the molecules and that of the fields. According to the first line in figure 5(e), almost no shift is observed for an achiral adsorption such as ethanol. Specifically, large dissymmetry is observed in the difference in resonance shifts of tryptophan and β-sheet proteins. The measured results also provide indirect information on the geometry of chiral molecules, which can be used to explain the asymmetric difference in the resonance shifts. As shown in figure 5(f), biomacromolecules rich in α-helices are more isotropically distributed at the interface, while the β-sheet proteins result in anisotropic aggregation in lateral directions.
5. Applications
5.1. Nonlinear optics in chiral metamaterials
The application of chirality in biochemical engineering is not limited to the linear regime. In fact, chiroptical effects in second-harmonic generation (SHG) are typically orders of magnitude larger than their linear counterparts [156–158]. In the second-harmonic process, two photons at a fundamental frequency are annihilated into a single photon at twice the frequency [159]. This response can be described by a nonlinear polarization, which can be written in the electric dipole approximation as where ω is the angular frequency,
is the second order susceptibility tensor, E is the electric field and i, j, and k are the Cartesian indices. By applying the inversion symmetry to this equation, we can find that SHG only exists in noncentrosymmetric materials or matter that lacks inversion symmetry. Thus chiral metamaterials are naturally suitable for SHG because all chiral structures are intrinsically noncentrosymmetric. Based on this concept, researchers have performed SHG measurements to detect the handedness of planar G-shape nanostructures [144, 160, 161], as well as twisted-cross gold nanodimers [162]. It has been demonstrated that the chirality can be distinguished with linearly polarized light regardless of the polarization direction and the polarization state of the second-harmonic light [160].
To better understand the underlying physics of the nonlinear process in chiral nanostructures, the relationship between second-harmonic responses and superchiral fields was investigated in [163]. Traditional Slavic symbols (gammadions) were chosen in the experiments, and the separation distance between neighboring units was gradually decreased from 493 nm to 64 nm. Simulation results indicated that the strong density of optical angular momentum at interfaces can give rise to the enhancement of a localized superchiral field and thus strong second-harmonic signals are observed in experiments. In addition, whereas conservation under space and time reversal causes the linear response in chiral metamaterials to be reciprocal, it has also been indicated that the nonlinear ones are in fact non-reciprocal [163]. CD spectra show that CD does not change its sign upon flipping the sample, which is generalized as reciprocal chiroptical behavior. In the nonlinear regime, however, the SHG-CD does change its sign. These findings provide the means for experimental mapping of the local superchiral fields and design guidance for plasmonic devices with strong chiral light–matter interactions. Based on this fact, the separation distance of neighboring units was optimized to enhance the macroscopic circular dichroism in the second-harmonic field (figure 6(a)). Strong SHG-CD signals have also been observed in twisted-arc structures that lack the four-fold rotational symmetry [61]. An image of the 'GT' logo has been created by a point-by-point calculation of the SHG-CD (figure 6(b)). In addition, anisotropic SHG-CD measurements in four-fold symmetric G-shaped nanostructures have demonstrated that both the value and the sign of the CD response are highly dependent on the incident angles of the fundamental illuminations [161]. The SHG probe can thereby also serve as an extremely sensitive probe of the structural symmetry, and allow us to distinguish between chirality and anisotropic effects.
Figure 6. Chiroptical effects in the second-harmonic regime. (a) SHG-CD from two chiral centers (gammadions with opposite handedness) as a function of the gap size. (b) SHG-CD image (right panel) of a 'GT' patterned logo based on twisted-arc structures and the SEM images of the two enantiomers (left panels). (c) SHG-CD of the sample with curved nanowires for 0° (blue circles) and 180° (red squares) orientation of the sample. (a) is adapted from [163], (b) is adapted from [61], and (c) is adapted from [165] with permissions.
Download figure:
Standard image High-resolution imageChiroptical effects in the second-harmonic field have also been investigated in anisotropic achiral structures [164, 165]. Figure 6(c) shows the measured circular dichroism in the second-harmonic field. Blue circles show the measured SHG-CD for the sample with the curvature of nanowires pointing down. The SHG-CD increases as the incident angle becomes larger. The situation is reversed when the sample is rotated by 180 degrees (red circles). Specifically, the achiral structure of curved nanowires has shown more than 50% visibility by exploiting the extrinsic chirality in the second-harmonic field [165].
5.2. Chiral light–matter interactions
The enhancement of chiral light–matter interaction is one of the research focuses in chiral metamaterials. Superchiral fields, as discussed before, have been suggested to overcome the problem of weak chiroptical signals in nature. Subsequently, significant effort has been given to find various nanostructures that enhance chiroptical signals, such as plasmonic structures [43, 145, 153, 155], dielectric nanoparticles [166] and negative-index metamaterials [167]. The chiral Purcell factor has also been proposed for characterizing the ability of optical resonators to enhance chiroptical signals [168]. In [169], the effect of parity-time symmetric potentials on the radiation of chiral dipole sources has been theoretically investigated. Through appropriate design of parity-time symmetric potentials, chiral quantum emitters can be distinguished by their decay rates.
The interaction between quantum emitter and chiral metamaterials has been experimentally investigated in [170]. In this work, chiral arc metamaterials were adopted as the two enantiomers, as shown in figure 7(a). Achiral quantum dots occupied the entire space around the upper arc and only a slight part of the lower arc. In the linear regime, the circular dichroism of the arc metamaterials reached as high as 50% at the wavelength of resonance (∼780 nm), which led to the highest contrast of the transmission images of the chiral metamaterials patterned into the Georgia Tech mascot. The light confinement at the resonant wavelength was largely boosted and thereby implied a chiral-selective enhancement in nonlinear light–matter interaction. Specifically, the chiral contrast of the two-photon luminescence was witnessed when a circularly polarized light was incident on the embedded quantum emitters. The two-photon luminescence from the chiral system was remarkably enhanced by over 40× with respect to a reference case without the metamaterials. Moreover, the enhancement was chirality-enabled and was sensitive to the handedness of the illuminations. The enhancement factor for the two spin states achieved a contrast as large as 3 in the experiment. These findings manifest the potential for applications in chiral-selective imaging, sensing, and spectroscopy.
Figure 7. Chiral light–matter interactions. (a) Schematic diagram of enantiomer A (top left) and enantiomer B (bottom left) of the twisted-arc chiral metamaterial. Quantum dots fill the volume mostly surrounding the region around the upper arc. The right panels show the SEM images of two enantiomers. (b) Two-photon luminescence profile at 800 nm under different input circular polarizations for enantiomer A. (c) Schematic diagram of a circular polarized light detector consisting of a chiral metamaterial with a semiconductor that serves as a hot-electron acceptor. (d) Experimentally measured (dots) and theoretically calculated (solid curve) photoresponsivity spectra under LCP (blue) and RCP (red) illumination for left-handed metamaterials. (a) and (b) are adapted from [170], (c) and (d) are adapted from [175] with permissions.
Download figure:
Standard image High-resolution imageApart from the enhancement of quantum emitters discussed above, another fascinating application is chirality-selective optoelectronics. In the photoemission process, light absorption in solids can produce hot carriers whose energies are larger than those of thermal excitations at ambient temperatures [171]. Hot carrier science has played an important role in the development of quantum mechanics in the past, and nowadays offers exciting opportunities for fundamental research and applications in optoelectronics, catalysis, and photochemistry. Hot electrons generated from light absorption in a metal can be emitted over a Schottky barrier to produce current. Properly designed plasmonic nanostructures may greatly enhance light absorption and provide valuable control over the emission of the hot electrons in practical applications [172–174]. Chiral plasmonic nanostructures enable disparate light absorption for different circular polarizations and result in chiral-responsive hot-electron devices. To prove this concept, an ultracompact device for circularly polarized light detection has been proposed [175]. As shown in figure 7(b), the designed chiral metamaterial consists of Z-shaped silver stripes on top of a dielectric spacer and an optically thick silver backplane. The chiral metamaterial acts as a perfect LCP light absorber at the resonant wavelength and it reflects nearly 90% of the light of the other spin state. The simulated circular dichrosim (CD = ALCP−ARCP) reaches as high as 0.9, promising enhanced discrimination between LCP and RCP in photodetection. In experiment, a Schottky barrier is formed by integrating a semiconductor layer (n-type silicon) on top of the chiral plasmonic structure. Photoresponse spectra match well with the measured absorption spectra, and demonstrate peak photoresponsivity of the resonant state up to 2.2 mA W−1. The corresponding quantum efficiency reaches as high as 0.2%. This efficiency is two times that of chiral organic semiconductor transistors [176]. Furthermore, large circular dichroism gives rise to a significant distinction in photocurrents for the two spin states, with a polarization discrimination ratio of 3.4 and a difference in photoresponsivity of 1.5 mA W−1.
5.3. Active chiral metamaterials
Active control over the chirality of metamaterials has the potential of serving as a key element of future optical systems such as polarization sensitive imaging and interactive display. However, achieving active control over chiral metamaterials is challenging since it involves the reconfiguration of the metamolecule from a left-handed enantiomer to a right-handed counterpart and vice versa. In the terahertz regime, the generation of charge carriers via an optical pump in silicon has been utilized to actively switch the overall handedness of chiral structures [177–180]. A pulsed laser working at 800 nm is utilized to excite electron–hole pairs across the 1.12 eV bandgap of silicon. Under the illumination of a 500 mW pump laser, the photoconductivity of silicon can be as high as 50 000 S m−1. This feature enables all-optical switching of the handedness in chiral metamaterials in the THz regime. An all-optical tunable chirality has also been realized in a double-layer metamaterial, which consists of a nonlinear nano-Au:polycrystalline indium-tin oxide layer between two L-shaped nano-antennas in the building block [181]. A 45 nm shift of the peak in the circular dichroism spectrum has been observed under a 40 kW cm−2 weak pump. This work opens up the possibility for ultralow-power and ultrafast all-optical tunable chirality at visible frequencies.
Switchable chirality can also be achieved by micro-electro-mechanical systems. In [182], a planar spiral structure is vertically deformed into its three-dimensional counterparts once a pneumatic force is applied (figure 8(a)). The pneumatic force is supplied through air channels with the pressure source of N2 gas regulated by a pressure injector. Enantiomer switching is realized by selecting the deformation direction, which allows the alteration of the polarity of the optical activity without changing the spectral shape. To eliminate the cross-polarization conversion from birefringence, spiral arrays with C4 symmetry have been further developed. A polarization rotation of up to 28 degrees has been experimentally observed, which provides a compact polarization modulator in the THz regime.
Figure 8. Active chiral metamaterials. (a) Displacement of the spiral structure with respect to the applied pressure of N2 gas. The sign of the pressure is positive when the pressure is applied to the bottom chamber, resulting in left-handed spirals, and vice versa. (b) Active chiral plasmonic dimer stack consisting of 50 nm GST-326 (red) with two 10 nm ZnS/SiO2 layers (gray) sandwiched between gold nanorods embedded in PC403 (light red). The right panel depicts the shift of CD signal when the amorphous-to-crystalline phase transition occurs. (c) Schematic diagram of the reconfigurable chiral metamolecules based on DNA self-assembly. Two gold nanorods are hosted on a reconfigurable DNA origami template consisting of two connected bundles, which subtends a tunable angle. (d) Measured CD signals of the plasmonic metamolecules over time at a fixed wavelength of 725 nm. The plasmonic metamolecules can be driven to either handedness by adding removal or return strands. (a) is adapted from [182], (b) is adapted from [189], (c) and (d) are adapted from [192] with permissions.
Download figure:
Standard image High-resolution imagePhase-change materials [183–186], whose refractive index can be modified by temperature, voltage or light pulses, have been integrated with metamaterials to dynamically control the properties of metamateirals [187, 188]. Very recently, active control of chirality has been experimentally verified in [189], in which a layer of phase-change material Ge3Sb2Te6 is sandwiched between two stacked nanorods (figure 8(b)). Such sandwich structures can ensure an optimum interaction between Ge3Sb2Te6 and the plasmonic near field, and does not require complex lithography. A thermally induced transition from the amorphous state to the crystalline state occurs when the phase-change layer is heated up to the transition temperature (160 °C), and leads to a change in refractive index from 3.5 + 0.01i to 6.5 + 0.06i. Simulation results demonstrate that a giant spectral shift of around 20% at mid-infrared frequencies occurs when the phase transition happens. The measured circular dichroism spectrum is highly consistent with the theoretical prediction and a large spectral shift of 18% is realized. The wavelength tunability has also been utilized to obtain a reversal in the sign of the circular dichroism. Thermal switching of the handedness at a fixed wavelength is realized by cascading an active right-handed chiral dimer with a passive left-handed one. In the amorphous state, the strong positive CD signals from the active right-handed chiral dimer dominate and make the entire structure right-handed. In the crystalline state, the CD peak red shifts and the signals from the passive left-handed chiral dimer determines the entire chirality of the cascade system. Experiments have demonstrated that for cascade chiral dimers, the CD signal at 4200 nm in the amorphous state is exactly opposite to that in the crystalline state. These findings pave a new way towards thermal-controlled polarization modulation in the mid-infrared region and may find potential applications in thermal imaging and detection.
DNA-directed assembly has recently been proven to be a viable method for the controlled arrangement of plasmonic nanostructures, thus offering reconfigurable chirality [190–192]. As shown in figure 8(c), two gold nanorods are hosted on a reconfigurable DNA template, which consists of two connected bundles folded from a long single-stranded DNA scaffold. The relative angle between nanorods can be controlled by two DNA locks that extended from the sides of the DNA origami bundles. By adding designed DNA fuel strands, the 3D metamolecules can be switched between different conformational states, thus giving distinct CD spectra. Both of the DNA locks are open in an initially relaxed state. In the left cyclic process, a removal strand is added to dissociate the DNA strand from the arm through a branch migration process. Unreactive waste is produced during this process. The two DNA bundles are joined together, and therefore a left-handed system is established. With the addition of a return strand, the DNA lock is opened again through a second brand migration process. Hence, the system returns to its relax state. Figure 8(d) presents the measured CD signal over time between three distinct states: relaxed, left-handed and right-handed. The wavelength here is fixed at 725 nm. This CD signal clearly describes the cycling between three states of this system. The combination of plasmonics with DNA technology provides a versatile platform for designing active devices and may advance the development of smart probes for life science and biochemistry.
6. Conclusion and outlook
We have seen rapid growth in the field of optical chiral metamaterials, and it will continuously advance. The development of bottom-up and top-down approaches pushes the operation frequency of chiral metamaterials to the THz region and ultimately the optical frequency. Top-down approaches often provide excellent fabrication accuracy and flexibility, yet they are normally costly, low speed and cannot be applied to mass production. The situation is almost reversed in the case of the bottom-up approaches, which are cheaper, faster and regarded as the best methods for mimicking the self-assembly process in real life. We expect that the combination of these two approaches could provide a balance between quality and cost for a promising metamaterial industry. The chiroptical response has been largely discussed in the linear regime, while the nonlinear chiroptical effect in chiral metamaterials is still in its infancy. We expect that a variety of nonlinear processes, such as third harmonic generation, optical rectification and parametric up/down conversion, can provide new routes for the manipulation and utilization of chiroptical responses in either the near or far fields. Judiciously designed plasmonic nanostructures can greatly enhance chiral light–matter interactions, which provide potential opportunities for detecting and characterizing the ubiquitous chirality in nature with unparalleled sensitivity. Although the underlying mechanism and some experimental verification have been demonstrated in several studies highlighted in this review, further fundamental studies are needed to optimize these processes. In particular, it is important to determine what kind of chiral structures are suitable for practical applications. We also expect that more active chiral devices can be produced, reaching dynamic control of not only the far-field chirality but also the near-field counterparts. Last, but not least, since many biologically active molecules and proteins are chiral, we believe that bio-inspired and bio-compatible chiral devices with performance that is superior to existing technologies are greatly needed. This in turn will significantly advance developments in biochemistry, biophotonics and other interdisciplinary areas.
Acknowledgments
Z Wang acknowledges the support from China Scholarship Council (201406320105), and Y Liu acknowledges the 3M Non-Tenured Faculty Award.