Abstract
Global greenhouse gas (GHG) emissions can be traced to five economic sectors: energy, industry, buildings, transport and AFOLU (agriculture, forestry and other land uses). In this topical review, we synthesise the literature to explain recent trends in global and regional emissions in each of these sectors. To contextualise our review, we present estimates of GHG emissions trends by sector from 1990 to 2018, describing the major sources of emissions growth, stability and decline across ten global regions. Overall, the literature and data emphasise that progress towards reducing GHG emissions has been limited. The prominent global pattern is a continuation of underlying drivers with few signs of emerging limits to demand, nor of a deep shift towards the delivery of low and zero carbon services across sectors. We observe a moderate decarbonisation of energy systems in Europe and North America, driven by fuel switching and the increasing penetration of renewables. By contrast, in rapidly industrialising regions, fossil-based energy systems have continuously expanded, only very recently slowing down in their growth. Strong demand for materials, floor area, energy services and travel have driven emissions growth in the industry, buildings and transport sectors, particularly in Eastern Asia, Southern Asia and South-East Asia. An expansion of agriculture into carbon-dense tropical forest areas has driven recent increases in AFOLU emissions in Latin America, South-East Asia and Africa. Identifying, understanding, and tackling the most persistent and climate-damaging trends across sectors is a fundamental concern for research and policy as humanity treads deeper into the Anthropocene.
Export citation and abstract BibTeX RIS

Original content from this work may be used under the terms of the Creative Commons Attribution 4.0 license. Any further distribution of this work must maintain attribution to the author(s) and the title of the work, journal citation and DOI.
1. Introduction
Greenhouse gas (GHG) emissions from fossil fuels and land use have continuously grown since the 19th century, reaching their highest ever level in 2019. The Paris Agreement in 2015 set out an ambition to limit the global temperature increase to 1.5 °C and 2 °C above pre-industrial levels. Yet, on the basis of current trends in emissions, planned infrastructure, and national policy commitments, the Paris targets are in jeopardy (Höhne et al 2020). The time window is narrowing to counter these trends across all sectors and global regions.
Global GHG emissions sources are usually attributed to five broad sectors, characterised by the Intergovernmental Panel on Climate Change (IPCC) Working Group III (WG3) as energy systems, industry, buildings, transport, and AFOLU (agriculture, forestry and other land uses). Together, these sectors cover aspects of energy supply (energy systems), energy demand (industry, buildings and transport), non-energy related process emissions (industry), and land-based emissions and removals (AFOLU).
Each sector encounters its own challenges in terms of climate change mitigation. GHG emissions from energy systems are dominated by coal-powered electricity generation, often from a limited number of highly polluting units that are long-lived and politically challenging to retire (Jakob et al 2020). Transport and building emissions are more diffuse and spread across many actors; they are linked to urban form, physical infrastructures and everyday behaviour, thus involving non-trivial technological and social challenges to mitigation (Creutzig et al 2015). Industry emissions are associated with the production of metals, chemicals, cement and other basic materials demanded by our economies. Many of these processes are inefficient and offer a large scope for rapid emissions cuts, although some are difficult to fully mitigate (Davis et al 2018, Rissman et al 2020). AFOLU emissions and removals are linked to the production of food, feed and timber production—a particularly difficult sector as these are essential services, carried out by millions of actors, on a globally limited area of land with multiple competing demands (IPCC 2019).
There have been few attempts to describe global and regional emissions trends and drivers on a consistent and comprehensive sectoral basis. There is a substantive literature that compiles global emissions inventories for carbon dioxide (CO2) (Grassi et al 2018, Quéré Le et al 2018, Friedlingstein et al 2019), methane (CH4) (Kirschke et al 2013, Saunois et al 2020), and nitrous oxide (N2O) emissions (Janssens-Maenhout et al 2019, Tian et al 2020). There are also dedicated IPCC chapters and reports analysing the contributions of each sector, including energy systems (Bruckner et al 2014), buildings (Lucon et al 2014), transport (Sims et al 2014), industry (Fischedick et al 2014) and AFOLU (Smith et al 2014, IPCC 2019). And it is commonplace for national and regional studies to analyse sectoral emissions trends and drivers, such as for China (Guan et al 2018), the US (Feng et al 2015), or the EU (Spencer et al 2017). In this study, we aim to update and synthesise these literatures, comprehensively and consistently covering all sources of GHG emissions, for all world regions and across all sectors, drawing from expertise in each area to explain key trends and drivers. The core research questions of this review are as follows:
- (a)What are the recent trends in global, regional and sectoral greenhouse gas emissions?
- (b)What are the driving factors underlying these trends?
In addressing these questions, we combine several elements of analysis. First, we draw from a comprehensive GHG emissions dataset to describe recent global and regional trends in sector emissions. This provides the context for an in-depth review of the main drivers underpinning sector emissions trends. We further support this review with sector specific Kaya decompositions, and other relevant descriptive data. Our aim is to provide a summary of the main trends and challenges, sector by sector, which collectively shape our prospects for a rapid and deep transition to avoid dangerous climate change.
2. Methods and materials
This article is a literature review supported by several elements of empirical analysis. Here we briefly summarise our approach to literature identification, data sources and analysis, with further details provided in the supplementary materials (available online at stacks.iop.org/ERL/16/073005/mmedia).
2.1. Literature search and inclusion
This topical review provides a targeted review of the literature on emission trends and their underlying drivers across different sectors of the economy (energy systems, industry, buildings, transport and AFOLU). Due to the broad scope and a potentially very expansive literature base, we inform and focus our review in each sector with a supplementary data analysis on global and regional emissions trends. This analysis (described in the following section) brings forward the main and fastest growing sources of GHG emissions in each sector, as well as the regional profiles of these emissions. It further identifies on going trends in key driving forces, elaborated in Kaya decompositions.
Based on these analyses, teams of sector experts determined the scope of their review, structured into common components across sectors. These include drivers of global and regional demand, and factors that influence emissions intensity and efficiency. We rely on the deep sectoral knowledge of our sector teams for the identification of the relevant literature. We also conducted targeted keyword searches on the Web of Science and Google Scholar platforms to inform our selection of literature. These include, for example, relevant activity data underlying sector trends (such as trends in 'building floor space' per capita, 'motorisation', or 'renewable energy deployment'). This focus on relevant key drivers in our searches ensures tractability given the scope of our review, but we search more exhaustively with a structured keyword query for Kaya literature in particular (the search string is provided in the supplementary materials).
2.2. Global and regional GHG emissions trends
We use the EDGAR v5.0 database to track global, regional and sectoral GHG emissions from 1990 to 2018 (Crippa et al 2019). EDGAR includes CO2 emissions from fossil fuel combustion derived from International Energy Agency data (IEA 2020c), supplemented with CH4 and N2O emissions from savannah burning sourced from the Food and Agricultural Organisation of the United Nations (FAO 2019), and CH4 and N2O from forest and peat fires taken from the Global Fire Emissions Database (GFED v4.1s; van der Werf et al 2017).
EDGAR does not include land-use change and management CO2 emissions and removals (hereafter 'land-use CO2' emissions). We therefore source these separately, using the average of three global bookkeeping models (Hansis et al 2015, Houghton and Nassikas 2017, Gasser et al 2020) in a convention established by the Global Carbon Project (Friedlingstein et al 2019). These include CO2 emissions from peat burning and draining from FAO and GFED.
Land-use CO2 estimates follow the approach of the global modelling community, which attributes anthropogenic CO2 fluxes based on underlying drivers, not where they occur (Friedlingstein et al 2019). In other words, fluxes caused by direct land-use change and land management processes are accounted for, while fluxes driven by, e.g. indirect anthropogenic effects of changes in environmental conditions on land not subject to modelled management activities, are excluded (Grassi et al 2018, Jia et al 2019). (Note that the latter would be included in the AFOLU flux under UNFCCC reporting, if they occurred on what countries define as 'managed land' for reporting, which is a larger area of forests than considered as subject to management by the models. Thus National Greenhouse gas Inventories sum to larger CO2 removals (and thus smaller net global CO2 emissions) than the global models because of the net effects of CO2-fertilisation, N fertilisation and climate change on the larger areas of 'managed forests' (Grassi et al 2018)).
We use 100 year global warming potentials as updated for the IPCC 6th Assessment Report (AR6) to facilitate comparability between the different gases in this data set (CO2, CH4, N2O and fluorinated (F-)gases). Emissions are presented as global and regional totals; the latter using a ten region split also developed for IPCC AR6. A list of countries within each region is available in the supplementary materials.
Consistency in the allocation of emissions and energy use to sectors is important for our analysis. We follow the IPCC 5th Assessment Report (AR5) in allocating emissions sources from the EDGAR database exclusively to five overarching sectors: Energy Systems, Industry, Buildings, Transport and AFOLU. Within each sector we construct a second level categorisation ('sub-sectors') comprising groups of structurally similar emissions sources. An explanation of these categories and the detailed sector allocation is available as a supplementary data file.
2.3. Direct versus indirect emissions
Our emissions estimates distinguish between two accounting methods: scope 1 or 'direct' emissions, comprising the emissions produced by owned or controlled sources; and scope 2, or 'indirect' emissions, where the emissions associated with the generation of electricity and heat in the energy systems sector are allocated to sectors where this energy is consumed. A scope 2 reallocation of indirect emissions is particularly consequential for the buildings and industry sectors, where some consumed energy is produced on-site (e.g. in gas boilers), but a large fraction is also sourced upstream from power plants via electricity consumption.
To allocate emissions from the electricity and heat sector to final sectors as indirect emissions, we use estimates provided in the CO2 emissions dataset of the IEA (2020a). These estimates are based on individual electricity and heat specific emission factors, and assumea fixed efficiency of 90% for heat plants (in order to calculate the share of emissions associated with heat versus electricity in combined heat and power plants). Since the total emissions of the electricity and heat sector in IEA differs to EDGAR, we calculate the proportion of indirect emissions in each final sector in IEA, then multiply these values using the EDGAR electricity and heat total.
We do not consider 'consumption-based' emissions, which comprise all embodied emissions associated with the consumption of a product or service. This is relevant for tracking upstream and downstream emissions related to regional and global supply chain networks (Hubacek et al 2014, Li et al 2020), but is beyond the limits of our work.
2.4. Decomposition of global and sectoral emissions drivers
To support our analysis and review of the main driving forces and regional differences underlying emissions trends, we perform a Kaya decomposition analysis for each sector (Kaya 1990). Kaya analysis is a common method applied across the climate mitigation literature, which expresses emissions (tCO2) as a function of population (persons), GDP (2010 US$, PPP) and energy (joules), with the respective terms F, P, G and E:

where G/P is GDP per capita, E/G is the energy intensity of GDP and F/E is the carbon intensity of energy. To apply the analysis at a sector level, we use the IEA World Energy Balances (IEA 2020c) to isolate the primary energy supply for the energy systems sector, and total final energy consumption for the industry, transport, and buildings sectors. We then match these sectors with CO2 emissions (F) sourced from the IEA (2020a). To ensure consistency in our sector definitions—between the prior emissions trends analysis (which uses EDGAR data) and this Kaya analysis (IEA data)—we rely on a detailed mapping of IEA energy sectors to EDGAR emissions sources, available in the supplementary materials.
The Kaya decomposition for these four sectors is narrower in scope than our overall estimates of GHG emissions, as it focuses only on the CO2 emissions associated with energy use, i.e. fossil fuel combustion. It does not include GHG emissions from cement, ceramics, landfill, land-use change and agriculture, as these are not directly related to energy use, and thus could not be evaluated in the same decomposition. Nor do we include indirect emissions in the decomposition. Finally, since there is no meaningful way to allocate population and GDP data for sectors, we simply use regional and global totals in every decomposition, using population estimates from the United Nations (UNDESA 2019) and GDP in purchasing-power parity terms from the IEA (2020a), extended with growth rates from the IMF (2020) for the most recent years.
The Kaya decomposition is conceptually straightforward in the case of energy systems, industry, buildings, and transport sectors. However, it is less appropriate for AFOLU, where underlying activities are indirectly driven by energy use and GDP. Instead, area of land under different uses and agricultural output are closer proxy drivers of AFOLU emissions. We therefore substitute the terms for an AFOLU specific decomposition, as established by Hong et al (2021), where AFOLU GHG emissions (tCO2eq) is the function of population (persons), agricultural output (kcals), and agricultural land area (hectares), with the respective symbols H, P, A and L:

A/P is agricultural output per capita, L/A is the land required for a unit of agricultural output, and H/L is GHG emissions per unit of land. In this decomposition H is composed of agricultural CH4 and N2O emissions from EDGAR supplemented with land-use CO2 emissions from the bookkeeping models (Hansis et al 2015, Houghton and Nassikas 2017, Gasser et al 2020). Due to land data constraints, this decomposition misses a single year (1990–2017) relative to other sectors (1990–2018).
2.5. Uncertainties and growth rate calculation
There are different uncertainties associated with historical GHG emissions estimates. These can be traced to uncertainties in (and a lack of) underlying activity and inventory data (particularly in non-OECD countries), the use of average emissions factors across countries and fuel types, different interpretations of oxidisation and combustion, and other assumptions (Andrew 2020).
Fossil fuel combustion emissions are generally regarded to have comparatively small uncertainties due to the sophistication and standardisation of historical energy data reporting. By contrast, uncertainties are much higher for AFOLU CO2 emissions and all other greenhouse gases. Following IPCC AR5 (Blanco et al 2014) we assume uncertainties of ±8% for global emissions of CO2 from fossil fuel combustion, ±20% for CH4 emissions and Fgases, ±50% for AFOLU CO2 emissions, and ±60% for N2O emissions.
Two particular subsectors are noteworthy for having both high emissions and high uncertainties: fugitive CH4 emissions and land-use CO2 emissions. Regarding the former, recent studies have found substantial discrepancies between nationally reported GHG inventories for oil and gas fugitive emissions versus observational evidence of anthropogenic CH4 emissions (Alvarez et al 2018, Weller et al 2020). Notably, Hmiel et al (2020) concluded from pre-industrial ice core 14CH4 measurements that natural geological sources of methane emissions are a much smaller fraction of total atmospheric methane than previously estimated, with a potential underestimate of global anthropogenic CH4 emissions of 25%–40%. The likely source is oil and gas infrastructure leaks, which occur across the supply chain, from extraction, processing and up to gas distribution and final use (Christian et al 2016, Alvarez et al 2018, Weller et al 2020).
Uncertainties around AFOLU CO2 emissions in the latest Global Carbon Budget are around 46% over 2009–2018, based on the mean and one standard deviation of three bookkeeping models (Friedlingstein et al 2019). BLUE emission estimates (Hansis et al 2015) are globally higher than the Houghton and Nassikas (HN) (2017) model, in part driven by the explicit representation of land-use transitions at the sub-grid scale in BLUE and the preferential allocation of pasture on natural grassland in HN (Hansis et al 2015). Further, trends of individual datasets differ over recent years, e.g. with an upward trend in BLUE vs a downward trend in HN since the 2000s for land-use CO2 emissions. These different trends are at least partly attributable to the underlying land-use forcing (Gasser et al 2020). The third model, OSCAR (Gasser et al 2020), follows the approximate mean of BLUE and HN.
We derive the growth rates of emissions trends and Kaya factors over periods greater than one year, as follows:
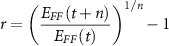
where is the emissions value or Kaya factor in a given year (t).
3. Results and discussion
3.1. All sectors
Global GHG emissions continued to rise between 2010 and 2018, although the rate of emissions growth has slowed since 2014 (figure 1). GHG emissions were the highest in human history in 2018, reaching 58 GtCO2eq. The largest share of emissions in 2018 came from the energy systems sector (34%; 20 GtCO2eq), followed by industry (24%; 14 GtCO2eq), AFOLU (21%; 12 GtCO2eq), transport (14%; 8.3 GtCO2eq) and the operation of buildings (6%; 3.3 Gt CO2eq). These estimates are based on direct emissions produced in each sector. As we discuss below, the industry and buildings sectors further contributed to emission growth indirectly, by drawing on electricity and heat production in the energy systems sector.
Figure 1. Global and regional GHG emissions trends for all sectors. Panel (a) shows total global anthropogenic GHG emissions divided into major sectors. Panel (b) shows regional emission trends in the years 1990, 2000, 2010, and 2018. This figure shows the direct (scope 1) allocation of emissions to sectors.
Download figure:
Standard image High-resolution imageGHG emissions in 2018 were about 11% (5.8 GtCO2eq) higher than GHG emission levels in 2010 (52 GtCO2eq). One third of this increase in GHG emissions between 2010 and 2018 was from energy systems (1.9 GtCO2eq), followed by industry (1.8 GtCO2eq, 30% of the increase), transport (1.2 GtCO2eq, 20%), AFOLU (0.72 GtCO2eq, 12%) and buildings (0.22 GtCO2eq, 4%).
In terms of regions, East Asia and North America together accounted for 40% of global GHG emissions in 2018, within which emissions are dominated by China and the United States. The highest absolute increase between 2010 and 2018 was in Eastern Asia (2.6 GtCO2eq), more than double the growth of the next highest region, Southern Asia (1.1 GtCO2eq). Four regions—the Middle East, Africa, Eurasia and South-East Asia—accounted for the rest of the global emissions increase with approximately 0.5 GtCO2eq each. The most rapid relative growth in emissions since 2010 occurred in Southern Asia at 3.6% per year, followed by the Middle East (2.6%/yr), Eastern Asia (2.4%/yr) and Eurasia (1.9%/yr). The only region with a decline in emissions since 2010 has been Europe (−0.3 GtCO2eq, −0.8%/yr). North America, Latin America, and developed countries in the Asia Pacific saw only minimal growth over this period (+0.1%/yr, +0.1%/yr and +0.4%/yr, respectively).
Trends by sector differ widely across regions. Developed countries in Asia Pacific, Europe and North America tend to have higher shares of emissions from energy systems, industry and transport, and lower shares from AFOLU. Overall emissions in these regions are relatively stable, apart from the energy systems sector in Europe and North America, which have seen gradual reductions since 2010 (−1.8%/yr and −1.5%/yr, respectively). This general pattern is reversed in the case of Africa, Latin America and South-East Asia. In these regions AFOLU is the largest emitting sector (specifically: CO2 emissions from deforestation), yet much of the recent growth comes from the energy systems, industry and transportation sectors. Similarly, fast growing emissions in Eastern and Southern Asia are mainly associated with the industry, energy systems and transport sectors, some at rates exceeding 4%/yr.
The largest individual sub-sector contributing to global GHG emissions in 2018 was electricity and heat generation at 13.9 GtCO2eq (24%). This subsector can be reallocated to consuming sectors as indirect (scope 2) emissions, thus highlighting the importance of energy demand as a driver of global climate change (de la Rue du Can et al 2015, Creutzig et al 2016, 2018). From this perspective, the relative importance of the industry and buildings sectors jump dramatically, from 25% to 35%, and 6% to 17%, respectively (figure 2).
Figure 2. Direct versus indirect emissions. The stacked bar on the left indicates total global greenhouse gas emissions in 2018, split by sectors based on direct (scope 1) emissions accounting. The arrows shown next to the electricity and heat sector depict the reallocation of these emissions to final sectors as indirect (scope 2) emissions. This increases the contribution to global emissions from the industry and buildings sector (central stacked bar). The stacked bar on the far right indicates the shares of subsectors in global emissions when indirect emissions are included.
Download figure:
Standard image High-resolution imageThe highest emitting subsectors after electricity and heat are 'other industry' 30 , land-use change and management, road transport, residential buildings, metals, chemicals, enteric fermentation (i.e. livestock rearing), non-residential buildings, oil and gas fugitive emissions, and the waste sector. Indirect emissions account for a large proportion of emissions in some of these sectors, particularly the residential and non-residential buildings subsectors (more than 50% of emissions), and the other industry, metals and chemicals subsectors (more than 20% of emissions). Among the largest sub-sectors, the fastest growing from 2010 to 2018 have been oil and gas fugitive emissions (+2.1%), road transport (+2%), coal mining fugitive emissions (+2%), and metals (+2%) (figure 3). Some of these emissions trends, however, are marked by significant uncertainty (see section 2.5).
Figure 3. Total global emissions by highest emitting subsectors. Only 15 subsectors out of 21 are shown. Indirect emissions from electricity and heat production are reallocated to subsectors as indicated. Growth rates in the left-hand panel are average annual rates across 2010–2018.
Download figure:
Standard image High-resolution imageOur data runs to 2018 and therefore misses the most recent break in emissions trends resulting from COVID-19 induced lockdowns in 2020. Several studies have observed sharp reductions in global daily emissions over the course of 2020 (Le Quéré et al 2020, Lenzen et al 2020, Liu et al 2020c). The lockdowns impacted emissions in the transport sector most heavily, particularly aviation, followed by electricity and industry emissions (Le Quéré et al 2020). Most of these reductions will be temporary, but COVID-19 may shape the driving forces of sectoral emissions in the long run, depending on the nature of stimulus packages and associated investments that are rolled out in the wake of the pandemic (Shan et al 2020).
We now turn our attention to the underlying factors of emissions growth. For this purpose, we decomposed the trends of CO2 emissions from fuel combustion (excluding other GHGs) into several driving factors, starting with an overview of all sectors in figure 4 and then investigating individual sectors in more detail in the following sections.
Figure 4. Kaya decomposition of CO2 emissions drivers (total of all sectors). The indicated growth rates are averaged across the years 2010–2018. Note that the energy term by itself is not part of the decomposition, but is depicted here for comparison with the Kaya factors. This figure is for fossil fuel CO2 emissions only, in order to ensure compatibility with underlying energy data.
Download figure:
Standard image High-resolution imageIn general, economic growth (measured as GDP) and its main components, affluence (GDP per capita) and population growth, all remained the strongest drivers of GHG emissions in the last decade, following the long-term trend (Burke et al 2015, Yao et al 2015, Malik et al 2016, Sanchez and Stern 2016, Stern et al 2017, Chang et al 2019, Dong et al 2019, 2020, Liobikiene and Butkus 2019, Liu et al 2019, Mardani et al 2019, Pan et al 2019, Parker and Bhatti 2020). Globally, affluence remained by far the strongest upward driver, increasing almost in tandem with energy consumption and CO2 emissions up until 2015, after which some modest relative decoupling occurred (figure 4).
The main counteracting, yet insufficient, factor that led to emissions reductions was decreased energy use per unit of GDP in almost all regions (−2.1% globally). These reductions in energy intensity are a result of technological innovation, regulation, structural change and increased economic efficiency (Yao et al 2015, Sanchez and Stern 2016, Chang et al 2019, Dong et al 2019, Liu et al 2019, Marin and Mazzanti 2019, Mohmmed et al 2019, Stern 2019, Wang et al 2019b, Goldemberg 2020). The decades-long trend that efficiency gains were outpaced by an increase in worldwide affluence continued unabated in the last 10 years (Haberl et al 2020, Wiedenhofer et al 2020, Wiedmann et al 2020). Therefore, GHG emissions only show relative, not absolute, decoupling from GDP at the global level (Deutch 2017, Wood et al 2018). In addition, the emissions-reducing effects of energy efficiency improvements are diminished by the energy rebound effect, which has been found in several studies to significantly offset some energy savings (Rausch and Schwerin 2018, Bruns et al 2019, Stern 2020).
A significant decarbonisation of the energy system was only noticeable in North America, Europe and Eurasia. Globally, the amount of CO2 per unit of energy used has remained practically unchanged over the last three decades, as the rapid growth of renewable energy in some regions has been matched by new fossil plants in others (Chang et al 2019, Jackson et al 2019, Peters et al 2020). Population growth has also remained a persistent upward driver in almost all regions (figure 4).
Global economic growth as the main driver of GHG emissions plays out particularly strong in China and India (Liu et al 2019, Ortega-Ruiz et al 2020, Yang et al 2020, Zheng et al 2020, Wang et al 2020b), although both countries show signs of relative decoupling because of structural changes (Marin and Mazzanti 2019). A change in China's production structure and consumption patterns (i.e. the type of goods and services consumed) have become the main moderating factors of emissions after 2010, while economic growth, consumption levels and investment remain the dominating factors driving up emissions (Jiborn et al 2020, Zheng et al 2020). In India low emission efficiency and expansion of production and trade caused the growth of emissions (Wang and Zhou 2020).
There are pronounced differences both in recent changes in the absolute levels and drivers of GHG emissions when differentiating countries by income levels (Dong et al 2019) or by regions (Chang et al 2019). In high-income countries, significant improvements in energy intensity led to declining CO2 emissions between 2010 and 2015, despite increasing income levels and populations (Dong et al 2019). In upper middle-income and lower middle-income countries, rising income more than offset any energy structural or intensity gains, leading to increased emissions. And CO2 emissions increased the most in low-income countries, due to significant increases in carbon intensities, income levels and population (Dong et al 2019). Importantly, some of these trends are partially related to shifts in global supply chains, where some production emissions could also be allocated to final consumers under a so-called consumption-based perspective, mostly in high- and middle-income countries (an issue we do not address in this article) (Peters et al 2011).
3.2. Energy systems
Overall, energy systems make up the largest share of the five sectors, with 34% of total global GHG emissions (although electricity and heat emissions can also be reallocated to demand sectors, particularly buildings and industry). GHG emissions in the energy sector grew to 20 GtCO2eq in 2018, with the strongest growth occurring between 2000 and 2010 (2.9% per year) and slowing down to 1.3% growth per year between 2010 and 2018 (figure 5).
Figure 5. Global and regional GHG emissions trends for the energy sector. Panel (a) shows total global energy systems GHG emissions divided into major subsectors. Panel (b) shows regional emission trends in the years 1990, 2000, 2010, and 2018. This figure shows the direct (scope 1) allocation of emissions to sectors. Note that emissions from the electricity and heat subsector are allocated as indirect (scope 2) emissions to the buildings, industry and transport sectors in the following sections (thus double counting between these respective sector figures).
Download figure:
Standard image High-resolution imageMost energy systems emissions are associated with the power sector, i.e. coal, gas and other plants that produce electricity and heat. These accounted for 71% of the total (14 GtCO2eq) in 2018. Fugitive emissions from oil and gas production (2.5 GtCO2eq) and coal mining (1.3 GtCO2eq) account for the second and third largest contributions. The overall growth of the energy systems sector between 2010 and 2018 (+1.9 GtCO2eq) can be mainly attributed to electricity and heat (+1.2 GtCO2eq, 62% of growth), followed by oil and gas (+0.37 GtCO2eq, 19% of growth) and coal mining (0.18 GtCO2eq, 9% of growth) fugitive emissions.
On a regional basis, East Asia stands out as the largest contributor to energy systems emissions in 2018 (6.3 GtCO2eq) and with the largest absolute growth from 2010 to 2018 (+1.2 GtCO2eq), averaging 2.6% per year. North America (2.8 GtCO2eq) is the second highest emitter, followed by Eurasia (1.8 GtCO2eq) and Europe (1.7 GtCO2eq). Of these, only Eurasia has grown in emissions (+0.1 GtCO2eq, 0.6%/yr), while Europe and North America have seen slight declines over the last decade (−1.8%/yr and −1.5%/yr, respectively, leading to −0.27 GtCO2eq and −0.35 GtCO2eq reductions). Southern Asia, South-East Asia and the Middle East are not amongst the largest absolute contributors, but they exhibit the largest annual growth rates of 4.9%, 4.3% and 3.3% respectively between 2010 and 2018. Africa, Asia-Pacific Developed and Latin America have seen only modest growth at or below 1% per year. Electricity and heat is currently the dominant source of GHG emissions from energy systems in various regions of the world, representing more than 50% of emissions in most and reaching as high as 80% in Southern Asia and East Asia. However, in some countries and regions fugitive emissions from oil and gas and coal production figure more prominently, such as in the Middle East (39% of energy systems emissions), Africa (31%), Latin America (25%) and Eurasia (24%).
At the global level, growth in CO2 emissions from energy systems have closely tracked rising GDP per capita (figure 6), affirming the substantial literature describing the mutual relationships between energy, electricity demand, exergy and economic growth (Khanna and Rao 2009, Stern 2011, Haberl et al 2020, Wiedenhofer et al 2020). This relationship has played out strongly in developing regions, particularly in Asia, where a massive scale up of energy supply has accompanied economic growth—with average annual increases of energy demand between 3.5% and 4.8% in the past decade (figure 6). (We cover these drivers of electricity demand in the industry and building sectors). The key downward driver has been declining energy intensities in almost all regions, associated with ongoing improvements in generation and transmission efficiency. Carbon intensities of energy supply have had a neutral effect, remaining globally stable since the 1990s, albeit with regional variations.
Figure 6. Kaya decomposition of CO2 emissions drivers for the energy systems sector. The indicated growth rates are averaged across the years 2010–2018. Note that the energy term by itself is not part of the decomposition, but is depicted here for comparison with the Kaya factors. This figure is for fossil fuel CO2 emissions only, in order to ensure compatibility with underlying energy data.
Download figure:
Standard image High-resolution imageOn the energy production side, almost all regions have seen steady decreases in energy intensities, at a global average of −2.1% per year since 2010, and at a similar steady pace in most individual regions albeit at varying rates. Technology benchmarking studies show that power generation efficiencies vary widely between (and also within) regions—generally higher in Europe, Japan and the United states, and lower in Russia, China, India and Australia (Maruyama and Eckelman 2009, Oda et al 2012). In the case of coal these differences are mainly driven by fuel qualities (e.g. lignite vs black coal) and the level of plant thermal efficiency (subcritical vs supercritical vs combined heat and power plants). Since newly deployed plants have higher efficiencies and older inefficient plants are steadily retired or retrofitted—particularly following concerted policy efforts, as is the case in China—the electricity output for a given quantity of fuel tends to improve over time, hence leading to an aggregate energy intensity improvement (Li et al 2020).
Improvements in carbon intensity can be decomposed into two distinct drivers: fossil intensity (the emissions intensity of fossil fuels) and fossil share (the substitution of fossil fuels by renewables) (Peters et al 2017). In the United States fossil intensity improvements have had a larger overall effect since 2006, due to a widespread coal to gas switch driven by low gas prices following a shale gas boom, and federal tax credit incentives (Peters et al 2017, 2020, Feng 2019, Mohlin et al 2019). Nonetheless, the overall share of fossil fuels in electricity production has also recently declined in North America (from 66% in 2010 to 59% in 2018; figure 7), with renewable capacity expanding rapidly in Texas, California and across the Midwest (Mohlin et al 2019). Declining fossil intensities associated with a coal to gas switch also drove down emissions in Europe in the early 2000s (Rodrigues et al 2020). But since 2007, Europe's carbon intensity improvements have instead been driven the steady expansion of renewables in the share of electricity generation (Peters et al 2017, 2020, Le Quéré et al 2019, Rodrigues et al 2020), with a fossil share decrease of 57% in 2010 to 47% in 2018 (figure 7). Some studies attribute these effects to climate policies, such as the carbon floor price in the UK, the EU emissions trading scheme, and generous renewable energy subsidies across the continent (Dyrstad et al 2019, Wang et al 2020a). Asia-Pacific Developed stands out in contrast to other developed regions, with a dramatic increase of regional carbon intensity and fossil share since 2010. This was due to the ramp up of coal and gas capacity in Japan following the Fukushima nuclear accident (Kharecha and Sato 2019). Generally, the use of natural gas for electricity production is growing strongly in most countries and gas has contributed to the largest increase in global fossil CO2 emissions in recent years (Jackson et al 2019, Peters et al 2020).
Figure 7. The fossil share of electricity and heat production by region in selected years. Regional shares of fossil fuels in the electricity and heat sector are weighted by total electricity and heat output. Data from the IEA World Energy Balances (IEA 2020c).
Download figure:
Standard image High-resolution imageSteady or increasing carbon intensities can be observed in most other regions, indicating further deepening of fossil fuel based energy systems worldwide (figure 6). A major driver of these trends is the global 'renaissance of coal' that started in the 1990s, primarily driven by a huge increase of coal generation capacity in China (Steckel et al 2015, Jiang and Guan 2016). The growth of coal emissions slowed after 2010, primarily due to a slowdown of economic growth and fewer coal capacity additions in China, and even declined between 2011 and 2018 (Friedlingstein et al 2019, Peters et al 2020). Discussions of a global 'peak coal', however, may be premature, as further growth was observed in 2019 (Friedlingstein et al 2019, Peters et al 2020). In addition, the renaissance has not been limited to China alone, with large ongoing and planned capacity increases in India, Turkey, Indonesia, Vietnam, South Africa and others (UNEP 2017, Edenhofer et al 2018, Steckel et al 2020).
The declining competitiveness of coal-based generation relative to alternatives, particularly solar PV, as well as its short-term health and environmental impacts, begs the question why many countries have continued to invest in coal capacity (Creutzig et al 2017, Lelieveld et al 2019, Rauner et al 2020). Historically, coal powered generation has been perceived as a relatively low-cost, stable, and technologically accessible option to expand grid electricity and meet growing consumption demands. As private and public utilities have invested in these technologies they locked-in technological pathways and shaped institutional environments (e.g. supportive financial, legal and political structures) that increase the costs of transitioning to alternatives. Recent studies show that incumbent energy utilities have only in rare exceptions transitioned a sizable share of their portfolios towards renewable energy (Alova 2020, Green et al 2020). It is rather new actors and interests driving these investments, often against considerable opposition and backlash from interest groups, particularly if implemented policies do succeed in scaling up renewable technologies (Moe 2015, Stokes and Breetz 2018). Fossil-based development pathways may also be chosen to meet the narrow goals of national and international interest groups, such as rent extraction or energy independence, and are shaped by issues such as lobbying, political ideology, and corruption (Dorband et al 2020, Jakob et al 2020, Lamb and Minx 2020, Roy and Schaffartzik 2021).
Overall, global energy system emissions growth has slowed in recent years, due to a reduction of fossil capacity additions in China, a structural shift to gas and renewables in the United States (Feng et al 2016), and the increasing penetration of renewables in Europe. The worldwide share of fossil fuels shrank slowly, down from 73% in 1990 to 68% in 2018. Despite this, global oil and gas use is still growing (Jackson et al 2019). The switch of coal to gas brings the risk of increased CH4 emissions from fugitive sources, as well as large cumulative emissions over the lifetime of the new plants that may erase early carbon intensity reductions (Shearer et al 2020). The focus of decarbonisation efforts in the energy systems sector needs to be on rapidly shifting to zero-carbon sources and actively phasing out all fossil fuels, rather than relying on the short-lived effects of fuel switching (Jackson et al 2019, Peters et al 2020).
3.3. Industry
Direct and indirect GHG emissions in the industry sector steadily increased to 20.1 GtCO2eq in 2018. Based on direct emissions alone, the industry sector is the second largest contributor to total emissions in 2018 (25%), following energy systems. When indirect emissions from electricity and heat production are included, industry becomes the single highest emitting sector (35%). In addition, industry has a steadily increasing share of all direct emissions since 1990 and faces non-trivial technological bottlenecks to mitigation, particularly in steel and cement process emissions, making it a key sector that will shape global mitigation prospects going forward (Davis et al 2018, Crippa et al 2019, Rissman et al 2020).
Apart from indirect emissions from the power sector (5.9 GtCO2eq, 30% of total), industry emissions in 2018 are largely driven by 'other industry' (4.5 GtCO2eq, 23% of total). 'Other industry' comprises a multitude of emissions sources associated with the manufacture of pulp and paper, food and tobacco, glass and ceramics, and other generic manufacturing. It also includes the production and use of fluorinated gases for solvents, refrigerants and electrical equipment. Three further subsectors account for basic materials production: metals (3.1 GtCO2eq, 15% of total), chemicals (2.8 GtCO2eq, 14% of total) and cement (1.6 GtCO2eq, 8% of total). Finally, waste (2.1 GtCO2eq, 11% of total) includes the emissions from incineration and waste disposal on land, as well as industrial, domestic and commercial wastewater processing.
The main period of industry emissions growth occurred between 2000 and 2010, which saw a total increase of 5.5 GtCO2eq (+3.8%/yr). Growth has subsequently slowed down to 1.6% per year since then, with an approximately proportionate contribution from each subsector. At a regional level, Eastern Asia stands out as the main source of global industry emissions (8.7 GtCO2eq in 2018, 43% of total), as well as the primary driver of growth since 2010 (+1.3 GtCO2eq, 2%/yr). At the same time, industry emissions remained high in Europe (2 GtCO2eq in 2018), but have slowly declined at a rate of −0.7%/yr. North America, Asia-Pacific Developed and Latin America have had stable industry emissions since 2010 (at 1.9, 0.8 and 1.1 GtCO2eq each). In all other regions they are growing—most rapidly in Southern Asia (+4.3%/yr to 1.8 GtCO2eq in 2018), South-East Asia (+3.4%/yr to 0.9 GtCO2eq) and Eurasia (+2.5%/yr to 1.3 GtCO2eq).
Regions differ somewhat in their composition of different subsectors, with waste and cement featuring more heavily in developing regions like Africa, Southern Asia and Latin America, while chemicals and other industry play a larger role in Europe and North America. Emissions from metal production take place primarily in East Asia (1.8 GtCO2eq), followed by Southern Asia (0.33 GtCO2eq), Eurasia (0.28 GtCO2eq) and Europe (0.22 GtCO2eq).
The main global driver of industry emissions has been the massive rise in demand for basic materials, construction minerals and manufactured products. These are in turn driven by rising affluence and consumption, as well as an increase in urban populations and associated infrastructure development (Krausmann et al 2017, 2018). Similar to the energy sector, the industry sector generates products that are indirectly used in final sectors—namely the materials that make up the manufactured capital of the physical economy, such as cement, chemicals, steel, aluminium, wood, paper, plastics, lubricants, fertilisers, and so on. These materials are used to build and maintain stocks of manufactured capital, including buildings, roads, vehicles, electronics, and machinery (also known as 'material stocks'; Krausmann et al 2017). Material stocks, which will remain in use over decadal time periods, reached 928 Gt in 2014, with a growth of 3.9% per year since 2010 and a 26-fold increase since 1900 (Wiedenhofer et al 2019). Alone, their production and use accounted for 11 GtCO2eq of global emissions in 2011, according to Hertwich (2021).
There is strong evidence that the growth of concrete, steel and other construction material use is tightly coupled to economic growth, urbanisation, and associated infrastructure development (Pauliuk et al 2013, Cao et al 2017, Krausmann et al 2017, Plank et al 2018, Haberl et al 2020). Per-capita stocks of cement and steel show a typical pattern of rapid take-off as countries urbanise and industrialise, before slowing down to low growth at high levels of GDP. Selected wealthy countries even seem to stabilise at high per-capita levels of stocks, although it is unclear if these stabilisations persist and if they result in significant absolute reductions of material use (Liu et al 2013, Pauliuk et al 2013, Fishman et al 2016, Cao et al 2017). Hence, in countries that are recently industrialising and urbanising—i.e. Eastern, Southern and South-Eastern Asia—we observe a particularly strong increase of emissions from these subsectors (figure 8) and a strong overall relationship between GDP growth and industrial CO2 emissions (figure 9).
Figure 8. Global and regional GHG emissions trends for the industry sector. Panel (a) shows total global industry GHG emissions divided into major subsectors. Panel (b) shows regional emission trends in the years 1990, 2000, 2010, and 2018. Indirect emissions from the electricity and heat subsector are shown here in grey.
Download figure:
Standard image High-resolution imageFigure 9. Kaya decomposition of CO2 emissions drivers for the industry sector (direct emissions only). The indicated growth rates are averaged across the years 2010–2018. Note that the energy term by itself is not part of the decomposition, but is depicted here for comparison with the Kaya factors. This figure is for fossil fuel CO2 emissions only (indirect CO2 emissions and process-related and waste emissions are excluded) in order to ensure compatibility with underlying energy data.
Download figure:
Standard image High-resolution imageOnce buildings, roads, ports, vehicles and other physical stocks have been constructed, a continuous throughput of material flows is still required to maintain, renovate, replace and operate them (Wiedenhofer et al 2015, Krausmann et al 2017). Material consumption in wealthier countries therefore has shown only limited rates of decrease, even though their large existing and only slowly growing stocks open up (as yet unexploited) opportunities for prolonging lifetimes and improving end of life recycling, so as to achieve absolute reductions in extraction activities (Krausmann et al 2017, Zink and Geyer 2017). For a given level of economic development, material stock levels and associated material use also varies due a variety of contextual factors, such as differences in prevailing construction methods and building codes (e.g. steel vs timber framing), patterns of urbanisation and infrastructure development (e.g. compact cities vs sprawl), trends in dwelling space and cars per capita, and the overall lifetimes of buildings and infrastructure (Lin et al 2016, Hertwich et al 2019, Lanau et al 2019).
As with the AFOLU sector, industrial emissions are strongly linked to international trade. Materials, especially metals, chemicals, plastics and wood products, are routinely transported between different stages of extraction, refining and production along global supply chains (Schaffartzik et al 2016, Plank et al 2018). Owing to a series of socio-economic conditions including low priced labour, state-led industrial policy and agglomeration effects, China currently dominates global industrial production, particularly in the manufacture of steel and other basic materials (Reck et al 2010, Wang et al 2019a). The global shift of energy-intensive industries away from historical centres in the United States and Europe to developing regions explains, to some extent, reductions of industry GHG emissions in the former—even though they continue to consume manufactured products via trade.
On the production side, improvements in the energy efficiency of material extraction, processing and manufacturing have reduced industrial energy use per unit of output (Wang et al 2019a; figure 9). These measures, alongside improved material substitution, light-weight designs, extended product and servicing lifetimes, improved service efficiency and increased reuse and recycling could enable substantial emissions reductions in the future (Hertwich et al 2019). Switching to lower or zero carbon feedstocks and power further leads to industry sector decarbonisation. Indeed, figure 9 shows that the ratio of industrial energy use to GDP has steadily declined since 2010 in all regions. Absent these improvements in energy intensity, growth of population and GDP per capita would have driven industrial CO2 emissions to rise by more than 100% by 2017 compared with 1990s, instead of 56%. Nonetheless, many studies point to deep regional differences in efficiency levels and large globally unexploited potentials to improve industrial energy efficiency by adopting best available technologies and practices for metal, cement and chemical production (Gutowski et al 2013, Schulze et al 2016, Gonzalez Hernandez et al 2018, Talaei et al 2018). Yet, decarbonising process emissions by technological improvements alone is unlikely to outweigh growing demand, calling for additional demand-side mitigation options to curb emissions from the industry sector (Creutzig et al 2016).
Overall, demand for services driven by global affluence and population growth have led to an escalation of material use and associated industry GHG emissions. Recent growth has been driven by emerging economies, but also high-income countries where direct and indirect consumption remains high. The growing complexity of international supply chains makes governance hard and problem-shifting likely. Historically, energy efficiency provided the largest mitigation wedge, but still failed to prevent GHG emissions from increasing. Furthermore, efficiency potentials will decrease in the coming decades as technological options are exhausted. This puts increasing focus on historically weak drivers of decarbonisation, such as demand management in end-use sectors (i.e. more efficient delivery of services), material efficiency (product lightweighting, longer lifetimes, use of secondary materials), fuel switching and electrification, and the decarbonisation of power and feedstocks (IRP 2020).
3.4. Buildings
Global GHG emissions from the buildings sector reached 9.8 GtCO2eq in 2018, of which 66% (6.5 GtCO2eq) were upstream emissions from power generation and commercial heat (figure 10). The remaining 34% (3.4 GtCO2eq) of emissions were directly produced in buildings, for instance by gas and coal boilers, and cooking and lighting devices that burn kerosene, biomass and other fuels. Residential buildings accounted for the majority of this sector's emissions (64%, 6.3 GtCO2eq, including both direct and indirect emissions), followed by non-residential buildings (35%, 3.5 GtCO2eq). Some non-CO2 sources (CH4 and N2O) also contribute to building emissions, but these are almost negligible (0.03 GtCO2eq) compared to other subsectors.
The buildings sector accounts for almost 6% of all direct GHG emissions and 17% when indirect 'scope 2' emissions are included. Yet further emissions components could also be attributed to this sector under alternative accounting schemes, such as consumption-based emissions footprints. Embodied GHG emissions associated with building materials and components, as well as the construction, assembly and maintenance of buildings, make up an estimated additional 11% of global energy and process-related CO2 emissions (Ürge-Vorsatz et al 2020). Adding these to the building sector would further increase emissions by approximately 2.21 GtCO2eq in 2018 (IEA 2020d).
The buildings sector contributed 0.7 GtCO2eq to global emissions growth between 2010 and 2018, an increase of 0.9% per year, which was relatively lower than prior decades where growth rates averaged between 1.7%/yr and 2.1%/yr (figure 10). A more than proportional amount of growth came from the residential (direct and indirect) subsector (+0.5 GtCO2eq, 76% of growth), compared to non-residential buildings.
Figure 10. Global and regional GHG emissions trends for the buildings sector. Panel (a) shows total global industry GHG emissions divided into major subsectors. Panel (b) shows regional emission trends in the years 1990, 2000, 2010, and 2018. Indirect emissions from the electricity and heat subsector are shown here in grey.
Download figure:
Standard image High-resolution imageThe developed regions of North America, Europe, and Asia-Pacific Developed together accounted for 41% (4 GtCO2eq) of global building emissions in 2018. In all three regions emissions declined over the period 2010–2018, at rates of −2.2%/yr, −2.5%/yr and −0.4%/yr, respectively. In the case of North America, almost all of the reduction (99%) was from indirect emissions (i.e. the energy systems sector); while in Europe at least one-third (35%) also came from decarbonisation in direct residential building emissions.
The regions of the global South and Eurasia together accounted for 59% (5.9 GtCO2eq) of global buildings emissions in 2018. Starting from a much lower base, buildings emissions increased significantly in these regions between 2010 and 2018, at rates of 4.9%/yr in Eastern Asia, 4.8%/yr in Southern Asia, 4.3%/yr South-East Asia, 3.1%/yr in Africa, 1.7%/yr in the Middle East, 1.2%/yr in Latin America and 0.5%/yr in Eurasia. Indirect emissions tended to grow faster than direct emissions, accounting for 80% of growth in Eastern Asia, 75% in Southern Asia and 92% SE Asia. This again underlines the close link between the buildings sector and energy systems—which over the past decades has tended towards the expansion of fossil-based electricity generation in these regions (section 3.2).
Population and GDP per capita growth are broad drivers of building emissions trends (figure 11), which manifest more specifically in a growing demand for building stock, floor space per capita, and building energy services as countries develop and urbanise (Ürge-Vorsatz et al 2015). At the same time, declines in carbon and energy intensities can be observed both globally and regionally over the past decades (figure 11), hinting at the impact of steadily improving efficiencies and shifts in the composition of fuels used in buildings (Ürge-Vorsatz et al 2020). Changes in the upstream power sector also figure strongly as drivers of indirect building emissions (but are not included in figure 11).
Figure 11. Kaya decomposition of CO2 emissions drivers for the buildings sector (direct emissions only). The indicated growth rates are averaged across the years 2010–2018. Note that the energy term by itself is not part of the decomposition, but is depicted here for comparison with the Kaya factors. This figure is for fossil fuel CO2 emissions only (indirect CO2 emissions are excluded) in order to ensure compatibility with underlying energy data.
Download figure:
Standard image High-resolution imageThe global stock of residential and non-residential buildings has been steadily growing over the past decades, driving a huge demand for building energy use and construction materials (Deetman et al 2020). Global building stock was estimated at almost 200 billion m2 of floor space in 2018, more than double of what it was in 1990 (IEA 2020d). China currently dominates total new additions to building stock, with urban residential buildings almost tripling since 1990 (Huo et al 2019, Marinova et al 2020, IEA 2020d). The same occurred in Southern Asia, South-East Asia and developing pacific while it has doubled in the developed world, Eurasia, Latin America and Caribbean as well as Africa and the Middle East (IEA 2020d).
As countries increase in wealth, developers tend to construct larger properties and more floor space is required to service growing demand in the retail, office and hotel sectors (Daioglou et al 2012, Deetman et al 2020). At the same time, patterns of urbanisation and sprawl further shape the density and overall scale of a country's building stock. In the United States, the stock of floor space in single and multi-family homes has continuously grown over the 21st century, increasing tenfold between 1890 and 2010 (Moura et al 2015).
Beyond population and wealth, demographic and social factors drive a cross-national trend of increasing floor space per capita. As populations age and decrease in fertility, and as individuals seek greater privacy and autonomy, households decline in size (Ellsworth-Krebs 2020). At the same time, a lack of 1–2 bed dwellings and the tendency for developers to construct larger family-sized properties may lead to over occupancy (Huebner and Shipworth 2017). Together these factors lead to increased floor space per capita, even as populations stabilise (figure 12). Overall, there remains a stark but converging divide in global residential floor space, driven by differences in household and dwelling size—from an upper range of 40–60 m2 per person in Western European and North American countries, to approximately 32 m2 in China and 20 m2 in Mexico (Nie and Kemp 2014; figure 12).
Figure 12. Residential floor space per capita versus income. Data from the IEA Energy Efficiency Indicators database (IEA 2020d). All countries trend from the left (2000) to the right (2017).
Download figure:
Standard image High-resolution imageIncreasing floor space per capita is a key driver for building sector emissions, because building characteristics such as size and type, rather than occupant behaviour, tend to explain the majority of energy use within dwellings (Guerra Santin et al 2009, Huebner and Shipworth 2017). Smaller household sizes result in increased per-capita appliance and equipment ownership (i.e. fewer people share appliances such as fridges and cookers), while larger homes increase the surface area to be illuminated and maintained at comfortable temperatures (Ürge-Vorsatz et al 2015).
Energy activity levels further drive regional differences. In Eurasia, Europe and North America, thermal demands for space heating dominate building energy use, at 66%, 62% and 48% of residential energy demand, respectively (IEA 2020d). In contrast, cooking has a much higher share of building energy use in regions of the Global South, including China (Cao et al 2016, Serrano et al 2017). And despite temperatures being on average warmer in the Global South, electricity use for cooling is a more prominent factor in the Global North (Waite et al 2017). This situation is changing, however, as rapid income growth and demographic changes in the global South enable households to heat and cool their homes (Ürge-Vorsatz et al 2015, 2020). Cooling energy demand represented 8% out of total residential energy demand in North America and the Middle East in 2018 (IEA 2020d). It was 3%–4% in Eastern Asia, Latin America and the rest of Asia (growing from less than 1% in 2010) and remained below 1% in Europe, Eurasia and Africa in 2018 (IEA 2020d). Annual variations in temperatures and extreme weather are also important drivers of overall demand (Idahosa et al 2017, Eggimann et al 2020).
Energy demand for small and connected devices and appliances is one of the emerging trends in residential buildings. The highest shares of energy demand for small and connected devices were observed in developed Asia-Pacific, with 24% out of total energy demand in residential buildings, followed by 15% in North America, and 13% in Middle East countries (IEA 2020d).
Steady improvements in building energy intensities across regions can be attributed to policies and baseline improvements in building fabrics, appliance efficiencies, and fuel shifts. Many countries have adopted a mix of relevant policies, such as energy labelling, building energy codes and mandatory energy performance requirements (Nie and Kemp 2014, Nejat et al 2015). Efforts towards buildings refurbishments and retrofits have also been pursued in several nations, especially for historical buildings in Europe, but evidence suggests that the recent rates of retrofits have not made a significant dent on emissions (Corrado and Ballarini 2016, Kerr and Winskel 2020). Green building retrofit policies in China too have been relatively ineffective to date (Liu et al 2020a, 2020b). Still, one major global factor driving down energy intensities has been the global transition from inefficient coal and biomass use in buildings for heating and cooking, towards natural gas and electricity, in part led by concerted policy action in Asian countries (Ürge-Vorsatz et al 2015, Kerimray et al 2017, Thoday et al 2018).
Overall, emissions from the building sector are expected to continue rising, especially in much of the Global South as housing gaps are filled and demand for floor area increases. As developing countries construct new buildings, there is much potential to reduce and use less carbon-intensive building materials, and to adopt building designs and standards that lower life cycle buildings energy use and allow for passive comfort. A significant shift from the use of solid heating and cooking fuels to gas and electricity in recent years is a trend that will continue and help reduce emissions. However, increasing appliance penetration in regions just gaining access to electricity will increase electricity related emissions from the building sector, unless accompanied by improved standards, labelling, and the decarbonisation of the electricity sector. Within the Global North, significant untapped potential for increasing heating, building fabric and material efficiency exists, as do behavioural shifts towards low-carbon lifestyles.
3.5. Transport
Global transport GHG emissions reached 8.5 GtCO2eq in 2018 and accounted for 14% of all direct and indirect emissions. Road transport passenger and freight emissions represent by far the largest component and source of this growth (6.1 GtCO2eq, 73% of total sector emissions), followed by international shipping (0.7 GtCO2eq, 8%) and international aviation (0.6 GtCO2eq, 7%). National plus international shipping and aviation emissions together account for 1.8 GtCO2eq or 21% of the sector total. Emissions from rail, indirect N2O emissions, and indirect CO2 emissions from electricity and heat (e.g. from the electrification of rail and bus transport) are relatively small, totalling 0.5 GtCO2eq or 6% of the current transport total.
Since 1990, global transport emissions have grown at a constant rate of about 2% per year. The road subsector accounted for most growth since 2010 (+0.9 GtCO2eq, at 1.9% per year), but inland shipping, domestic aviation and international aviation were the fastest growing subsectors at +3.2%/yr, +2.8%/yr and +2.7%/yr, respectively. North America, Europe and Eastern Asia stand out as the main regional contributors to global transport emissions and together account for 60% of the total. Latin America (in 4th place) is also notable for its high transport emissions, since in all other sectors apart from AFOLU it tends to be one of the lowest regional contributors.
Three regions managed to hold transport emissions relatively stable since 2010—Asia Pacific Developed (+0%/yr), Europe (+0.4%/yr) and Eurasia (+0.4%/yr)—but all others continued to grow. Fastest growth since 2010 occurred in Eastern Asia (+5.6%/yr, +0.4 GtCO2eq), Southern Asia (+4.9%/yr, +0.1 GtCO2eq), South-East Asia (+4.5%/yr, +0.1 GtCO2eq) and Africa (+3.3%/yr, +0.08 GtCO2eq). The proportion of total final energy used in transport (28%) and its fast expansion over time weighs heavily on climate mitigation efforts as 92% of transport energy comes from oil based-fuels (IEA 2020c). These trends situate transport as one of the most challenging sectors for climate change mitigation—even wealthier countries have so far been unable to realise significant emissions reductions in the sector.
More so than any other sector, transport energy use has closely tracked GDP per capita growth (figure 14). Transport facilitates the movement of people and goods, enabling access to essential services and social interactions, and driving local and global economies. Developments since 1990 continue a historical trend of increasing travel distances and a shift from low- to high-speed transport modes that goes along with GDP growth (Schäfer et al 2009, Gota et al 2019). Only modest improvements in energy efficiency have been realised, averaging 1.3% per year globally, while carbon intensities have remained stable (figure 14). Overall, global increases in passenger and freight travel activity levels have outpaced energy efficiency and fuel economy improvements, continuing a long-term trend for the transport sector (Gucwa and Schäfer 2013, Grübler 2015, McKinnon 2016).
Figure 13. Global and regional GHG emissions trends for the transport sector. Panel (b) shows emissions at the years 1990, 2000, 2010, and 2018. Indirect emissions from electricity and heat consumed in transport are shown here, but are not added to the transport sector totals in figure 1 (total emissions for all sectors). International aviation and shipping is included in panel (a), but excluded from panel (b).
Download figure:
Standard image High-resolution imageFigure 14. Kaya decomposition of CO2 emissions drivers for the transport sector. The indicated growth rates are averaged across the years 2010–2018. Note that the energy term by itself is not part of the decomposition, but is depicted here for comparison with the Kaya factors. This figure is for fossil fuel CO2 emissions only (indirect CO2 emissions are excluded) in order to ensure compatibility with underlying energy data.
Download figure:
Standard image High-resolution imageWhile global passenger activity has expanded in all world regions, great disparities exist between low and high income regions, and within countries between urban and rural areas (ITF 2019). These disparities are visible comparing regional levels of transport emissions (figure 13), and are largely due to differences in modal split and motor vehicle use. Europe and North America underwent a rapid increase in private car use in the 20th century, which has come to dominate most passenger travel activity and emissions, accounting in 2017 for 70.9% of passenger-km by motorised transport in the EU-28, and 81.1% in the US (EC 2019). However, the growth of passenger-km in the OECD has considerably slowed, down to an increase of just 1% between 2000 and 2017 (SLoCaT 2018). It is not clear whether the so-called 'peak car' in developed economies is simply the result of economic stagnation and rising fuel prices or whether it reflects deeper lifestyle and demographic change (Goodwin and van Dender 2013, Kuhnimhof et al 2013, Bastian et al 2016). Meanwhile, emerging countries in the global South are becoming more car dependent, with rapidly growing motorisation and urban sprawl, and the emergence of local automotive production, while public transport struggles to provide adequate service (Dargay et al 2007, Hansen and Nielsen 2017, Pojani and Stead 2017). Between 2000 and 2017 global passenger travel increased in non-OECD countries by 169%, starting from a low baseline (SLoCaT 2018).
Passenger and freight mobility services have seen far reaching advances, aided by information and communication technologies tools that have fostered e-commerce and mobility sharing platforms. The easing of traveling and the lowering of cost conditions attained through use of innovative business models and information technology have contributed as drivers of increasing transport demand and have shaped the way people and goods travel around the globe (Smidfelt Rosqvist and Hiselius 2016). Freight travel activity grew across the globe by 68% in the last two decades driven by global GDP increases, together with the proliferation of online commerce and rapid (i.e. same-day and next-day) delivery (SLoCaT 2018). Growth has been particularly rapid in heavy-duty road freight transport.
The transport sector global energy intensity dropped by an average of 1.3% per year between 2010 and 2018, primarily driven by wealthier regions, but was relatively level or increasing in all other regions (figure 14). Some countries have mobilised policies to increase vehicle energy efficiency, stimulating technological improvements that steadily reduced the required energy use per passenger-km and per tonne-km of goods delivery for all vehicle applications (light duty, heavy duty and rail) (IEA 2019a). Yet progress in another key policy area, fuel price reform, has been more mixed: fuel taxes increased in 83 countries, but fell in 46 between 2003 and 2015, despite their importance in encouraging the purchase of more efficient vehicles and in limiting travel demand (Bastian et al 2016, Ross et al 2017).
The energy matrix of the global transport system remains to the present deeply rooted in fossil fuels, with powertrains depending on gasoline and diesel fuels accounting for 92% of final energy use in the light duty vehicle segment (Figueroa et al 2014, IEA 2019a). The carbon intensity of the transport sector has remained unchanged between 2010 and 2018 in all world regions (figure 14). In part this is due to the increasing adoption of larger, heavier combustion-based vehicles ('sports utility vehicles' or SUVs), which have tended to far outpace electric and hybrid vehicle sales. Historically this has taken place in the North American car market, but is increasingly the case in Europe and international markets, with 39% of vehicles sold globally in 2018 in the category of SUVs (IEA 2020d). In terms of car size and weight, stringent material efficiency and light-weight design of passenger vehicles alone could cumulatively reduce global GHG emissions until 2060 by 16–39 GtCO2eq (Pauliuk et al 2020). Since 2011, there has also been a steady and increasingly steep decline in the market share of more carbon-efficient diesel vehicles in EU-28 countries; starting in 2017 these developments reversed the previously downward trend of average fuel consumption for newly registered vehicles in Europe (IEA 2020b).
While accounting for a small share of total GHG emissions, national and international aviation and shipping play a fast-growing role, with annual growth rates of +4.2% and 3.1% respectively between 1990 and 2018. Energy efficiency improvements in aviation were considerably larger than in road transport, but were outpaced by even larger increases in activity levels (SLoCaT 2018, Lee et al 2021). In 2016, aviation accounted for 31% of tourist arrivals globally (up from 17% in 2005), and for 50% of transport-related CO2 emissions from tourism (UNWTO 2019). The long term impact of the Covid-19 pandemic is uncertain but it has severely impacted global passenger aviation in 2020, and to different extent the movement of goods and freight modes (Le Quéré et al 2020, Newman AO 2020).
Overall, transport trends reveal a steady increase of emissions, overwhelmingly driven by growing motorisation and road transport activity. This global trend is opposite to what is needed, with a shift from lower to higher-carbon modes only minimally offset by efficiency gains. In recent years, hybrid electric and fully battery electric vehicles however, are becoming increasingly popular (IEA 2019b) and electrification has progressed faster than previously expected just a few years ago, especially in scooters, buses and a variety of micromobility and light urban freight modes such as e-bikes and e-autorickshaws (Taiebat and Xu 2019). Yet while the electrification of road transport holds much promise, its impact has been hardly visible in the period up to 2018, and looking forward it is in danger of being offset by growing levels of travel activity and countervailing trends such as increasing vehicle size and weight. This suggests a key role for more stringent policies and efforts to tackle car dependence, including demand management policies alongside technological innovation (Creutzig et al 2018, Mattioli et al 2020, Milovanoff et al 2020). Demand management is even more crucial for subsectors like aviation where technological options for decarbonisation are currently very limited.
3.6. AFOLU
AFOLU sector emissions and removals reached 11.6 GtCO2eq globally in 2018, or approximately 21% of global GHG emissions. Reporting in the AFOLU sector is split into two major components: CO2 emissions and removals from land-use change and management (also known as LULUCF—land use, land use change and forestry); and emissions from agriculture, which are predominantly CH4 and N2O.
Land use change and management CO2 emissions and removals account for 47% of net emissions in the AFOLU sector and are one of the largest global subsectors at 5.4 Gt CO2eq. A variety of land-based carbon fluxes make up these emissions, including: (a) deforestation (e.g. the clearing of natural vegetation for agricultural purposes); (b) transformations between croplands and pasture; (c) peat drainage and burning; (d) wood harvesting; (e) the regrowth of forest and other natural vegetation after agricultural abandonment and harvest; and (f) soil CO2 flux due to grassland and cropland management (Hansis et al 2015, Houghton and Nassikas 2017, Gasser et al 2020).
Emissions from agriculture are mainly comprised of CH4 emissions from enteric fermentation (e.g. cattle and sheep digestion), at 2.8 GtCO2eq in 2018, or 25% of the sector total. Smaller emissions components include managed soils and pasture (1.3 GtCO2eq, 11% of total), rice cultivation (1 GtCO2eq, 9% of total), and manure management, biomass burning, and synthetic fertiliser application (together 1 GtCO2eq). These agricultural sources are mainly CH4 and N2O emissions. Like land-use and management CO2 emissions, they are subject to considerable uncertainty (section 2.5).
There has been a gradual upward trend of AFOLU emissions since 2000, at rates of 0.8%/yr. Regionally, this growth has played out via differing patterns of land use and ongoing agricultural transitions. Unlike all other sectors, AFOLU emissions are typically higher in developing compared to developed regions. In Africa, Latin America and South East Asia, CO2 emissions associated with land-use change and management predominate, dwarfing other AFOLU and non-AFOLU sources, and making AFOLU the single largest sector at more than 50% of emissions in these regions. Land-use and management emissions here is associated with the expansion of agriculture into carbon-dense tropical forest areas, where vast quantities of CO2 emissions are released due to the removal and burning of biomass, and draining of carbon rich soils (Pearson et al 2017, IPCC 2019, Hong et al 2021).
By contrast, in Europe and North America, the highest rates of land clearing occurred well before the 20th century, such that legacy land use CO2 emissions are now negligible (Pongratz and Caldeira 2012). In Europe, recent forest regrowth and regeneration on abandoned agricultural land has led to an increase of carbon stocks and CO2 removals since at least 2010 (figure 15). Agricultural N2O and CH4 emissions therefore have a substantially larger share of overall AFOLU emissions in these regions compared to tropical countries (Hong et al 2021).
Figure 15. Global and regional GHG emissions trends for the AFOLU sector. Panel (b) shows emissions at the years 1990, 2000, 2010, and 2018.
Download figure:
Standard image High-resolution imageLivestock rearing takes place on vast tracts of pasture land worldwide, contributing to large quantities of CH4 emissions from enteric fermentation in Latin America (0.7 Gt CO2eq in 2018), Southern Asia (0.5 GtCO2eq) and Africa (0.5 GtCO2eq), while also playing a sizable role in the total AFOLU emissions of most other regions. Other emissions components are more specific to regions, such as rice cultivation in East, Southern, and South East Asia; biomass burning in Africa and Asia-Pacific Developed; and manure management in Europe and North America.
Trends in AFOLU emissions from 2010 to 2017 have been driven by increases in population, in particular in Africa, the Middle East, Southern Asia and South East Asia and Developing Pacific, increases in agricultural production per capita in all regions but the Middle East, and increases in emissions per unit of land area. The main downward driver was reductions in the amount of land required per unit of agricultural and forestry production in all regions (−2.7%/yr globally), reflecting agricultural intensification and technological progress. Notable features since the 1990s are the collapse of the Soviet Union, temporarily increasing land intensity and reducing per-capita production (Schierhorn et al 2019), and the increase in the emissions intensity of land use where large areas of pristine rainforest were cleared (in particular Africa, Latin America and Caribbean). Overall, AFOLU emissions are either relatively stable or are increasing steadily across most regions.
Land is the central, limiting resource for the production of food, feed, timber, bioenergy and other biocrops, mediated by growing population numbers, dietary patterns, and production efficiency (Kastner et al 2012). Large and on-going land-use and management CO2 emissions are consistent with a significant global expansion of anthropogenic land-use between 1990 and 2018 (Hurtt et al 2020). Humans have expanded cropland areas (+1.38 million km2, a 9.1% increase), secondary forests (+5.73 million km2, 22.5%), and urban land (+0.24 million km2, 64.3%), and have simultaneously shrunk primary forest areas (−7.25 million km2, −12.9%) (figure 16). These land-use changes have been led especially by Africa, Latin America, and Southeast Asia (Hurtt et al 2020).
Figure 16. Area and trend of land-uses in the world and selected regions. Data from the Land-Use Harmonization 2 database (Hurtt et al 2020, Chini et al 2021).
Download figure:
Standard image High-resolution imageThe AFOLU sector and its emissions impacts are closely tied to global supply chains. Countries like Brazil and Argentina use about half or more of their cropland for products exported to other countries, mainly the EU and China (Yu et al 2013). Of the soybeans produced in Brazil—the number two country-product combination with respect to GHG emissions in the global AFOLU sector after Indonesian rice (Hong et al 2021)—more than half was exported to China in 2017 (zu Ermgassen et al 2020). Difficulties in tracking supply chains and limited corporate accountability result in such exports often being associated with illegal deforestation (Vasconcelos et al 2020). In South-East Asia, particularly Indonesia, expansions of cropland by 0.18 million km2 (28%) was largely driven by international demand for palm oil, rubber, and plantation products (Austin et al 2019, Xin et al 2021). Here, expansion often occurs on peatlands, leading to high emissions through peat draining (Conchedda and Tubiello 2020) and peat burning (van der Werf et al 2017). The strong increases in production per capita and associated GHG emissions seen in Latin America and South-East Asia (figure 17) are thus at least partly attributable to growing exports and not national dietary changes.
Figure 17. Kaya decomposition of GHG emissions drivers for the AFOLU sector. Reproduced from Hong et al (2021).The indicated growth rates are averaged across the years 2010–2017. In contrast to other Kaya figures, here we show total GHG emissions for the AFOLU sector.
Download figure:
Standard image High-resolution imageAt the same time, efforts to promote environmental sustainability in regions like the EU and the US (but also fast-growing emerging economies such as China) can take place at the cost of increasing land displacement elsewhere to meet their own demand (Meyfroidt et al 2010, Yu et al 2013). Creutzig et al (2019) emphasise these global connections via different country 'archetypes' that explain co-occurring land use changes: 'Consumers' such as Europe feature stagnating population, agricultural intensification, and a high reliance on imports. 'Producers' are regions with high biocapacity and low institutional capacity such as South America, Russia, Indonesia. In these countries, exports play a large role for land-use trends. Other regions of Asia and Africa belong to the 'movers', where high population growth is rather the main driver.
GHG emissions per unit of land also increased recently in tropical regions, as a portion of deforestation activities moved into carbon-dense rainforests. In Latin America, land-use and management CO2 emissions grew from 1990 to 2010 (figure 15) due to high continuous Amazon deforestation rates until the mid-2000s alongside accumulating legacy emissions (INPE 2020). However, deforestation rates subsequently decreased until 2018 following government initiatives and international moratoria (Nepstad et al 2014). This trend in emissions is likely to reverse past our time period of analysis, as the 2019 and 2020 seasons saw the highest Amazon deforestation rates since 2008 (Silva Junior et al 2021). In other regions, emission intensity increases are often due to an overall increase in agricultural intensification, such as fertiliser application. The higher emission intensity for both land use CO2 and agricultural emissions is the cost for requiring less land per unit of production, which becomes evident in almost all regions.
Global diets are a key driver of production per capita, and thus land pressure and AFOLU emissions. As per capita incomes rise and populations urbanise, traditional diets that emphasise starchy foods, legumes and vegetables transition towards energy-intensive products such as refined sugars and fats, oils and meat (Tilman and Clark 2014). At a certain point in national development, diets thus override population growth as the main driver of AFOLU emissions (Kastner et al 2012). Over the last few decades, low- and middle-income countries such as India, Brazil, Egypt, Mexico and South Africa have experienced such a rapid dietary 'westernisation' (Pathak et al 2010, Vermeulen et al 2012, De Carvalho et al 2013, Popkin 2015). Another driver of higher food requirements per capita is food waste, the amounts of which increased more or less continuously since the 1960s in all regions but Europe (Porter and Reay 2016). Globally, 25%–30% of food produced is lost, in particular at the consumer stage in developed countries, and in the production, harvesting and distribution stages in less developed countries (Porter and Reay 2016, IPCC 2019). In other words, a significant fraction of the world's agricultural area is used annually to produce food that is wasted.
Overall, the AFOLU sector contributes to one quarter of global GHG emissions and the Paris Agreement must include mitigation efforts from this sector. AFOLU emissions were rather constant between 1990 and the early 2000s, despite continuous population growth and higher agricultural production per capita in most regions. The key counteracting process was less land required per unit of agricultural production due to agricultural intensification. Starting in 2001, however, AFOLU emissions increased again due to the clearing of pristine, carbon-dense forests. Tropical regions across all continents cause the majority of AFOLU emissions, with increasing emissions in Latin America and Southeast Asia clearly linked to global supply chains. These trends do not appear to be stabilising, underlining the urgency of interventions at all scales. Per capita emissions have also not fallen below 0.5 tCO2eq in any region (Hong et al 2021), suggesting a current frontier of mitigation efforts. If there is a residual of AFOLU emissions that cannot be eliminated, carbon dioxide removal methods such as re/afforestation and biomass plantations with carbon capture and storage would be required to meet stringent climate targets, further putting pressure on global land resources (Allen et al 2018).
4. Conclusions
Global GHG emissions have continued their steady rise, following over a century and a half of growth. Emissions have risen across sectors and subsectors, in electricity production, from industrial sources, land-use and management, and transport, through to comparatively small sources such as fugitive emissions, waste burning and aviation. Among the major emitting regions, recent trends show a picture of stable emissions in North America and modest declines in Europe, as fuel switching from coal to gas and the ramp up of renewables start to take hold. The huge emissions growth in Eastern Asia (China) is now also slowing due to fewer coal power additions in recent years. Together these trends led to a dampening of growth in 2010–2018, compared to previous decades. As emerging regions—Africa, Southern Asia, and South East Asia—are now poised to accelerate economic growth and establish high emitting infrastructures, it remains unclear whether a global peak in greenhouse gases will be reached soon. Regardless, recent growth has locked in a large body of infrastructure that will continue driving emissions into the future, in the absence of stringent climate policy or early retirement.
Across sectors, there is a growing demand for the products and services that underpin global emissions. In part this is due to the physical expansion of economies worldwide, with more infrastructures, vehicles, buildings, supply chains and other material stocks being put into place that demand energy for their production and use. Unfortunately, prevailing consumption patterns have also tended to aggravate energy use and emissions, with the long-term trend led by developed regions. Residential buildings have grown larger, have fewer inhabitants, and are heated and cooled to higher comfort standards. Motor vehicles, too, have grown in size and are driven over greater distances. Diets rich in meat and refined products, internationally sourced, are globalising and replacing traditional and seasonal produce. Regional and local contexts account for much variation, and there remains a chasm between the consumption levels of the global North and the South, the poor and the rich (Hubacek et al 2017, Oswald et al 2020, Wiedmann et al 2020). Nonetheless, the overall trend tracks towards westernised and highly unsustainable norms and patterns of consumption, for a small but growing global population.
Decarbonisation gains from improvements in energy efficiency and switching towards lower-carbon services across different sectors have been largely wiped out by increases in demand (or in some cases a trend towards higher carbon intensities). One exception is energy systems emissions in Europe, where a combination of stable demand, energy efficiency improvements, fuel switching and a scale up of renewables has led to an absolute decline in emissions. Other regions and sectors have deepened their reliance on inefficient, carbon-intensive infrastructures and production patterns. The continued failure to decarbonise transport, and the recent expansion of anthropogenic land-use into tropical rainforests, are two notable examples. There are huge unexploited mitigation potentials to be gained from avoiding these and other highly carbon-intensive activities, including by adopting the best available technologies and practices across sectors.
The energy systems, industry, buildings, transport and AFOLU sectors are deeply interconnected. Important linkages are electrification and heating, which ties emissions in the building and industry sectors upstream to energy systems, and material use, which ties embodied emissions in buildings to the industry sector. Following the general trend of electrification, which has yet to occur at scale in the transport sector, energy system decarbonisation will further increase in importance. Yet if the industry sector lags behind in electrification and the decarbonisation of process emissions (Davis et al 2018), it may become the primary source of indirect emissions embodied in the capital stocks of other sectors. Indeed, GHG emissions embodied in buildings and infrastructure, machinery and transport equipment already exceed 50% of their present carbon footprint (Chen et al 2018). Multi-sector and integrated policies are therefore crucial for meeting the mitigation challenge.
Overall, sectoral trends reveal limited progress towards decarbonisation. The prominent global pattern is a continuation of underlying drivers with few signs of emerging limits to demand, nor of a deep shift towards the delivery of low and zero carbon services across sectors. The main gap in knowledge is how rapid technological transitions, demand management and alternative economic models could be implemented to mitigate these persistent and powerful upward drivers. Linked to this is the question of how the physical material throughput of economies can be reduced without jeopardising economic and social well-being. Above all, there is a neglect of research on transitions towards even more unsustainable technologies and practices (Antal et al 2020). Climate mitigation cannot be achieved if status-quo trends continue, nor if low-carbon technologies and practices are left to gradually phase-in on an uneven playing field. Meeting the Paris Agreement goals of 1.5 °C and 2 °C requires policies that ensure sustainable land-use practices, limit excessive demand, and actively phase out fossil fuels.
Acknowledgement
This work was supported by the Bundesministerium für Bildung und Forschung of Germany (IPCC-AR6-III- 2, Grant No. 01LG1910A & ARIADNE, Grant No. 03SFK5J0); the European Research Council (ERC) under the European Union's Horizon 2020 research and innovation program (MAT_STOCKS, Grant No. 741950); the Natural Environment Research Council (NERC) of the United Kingdom (OPTIMISM, Grant No. NE/S012834/1); the US National Aeronautics and Space Administration (NASA) Interdisciplinary Research in Earth Science (IDS) program (Grant No. 80NSSC17K0348); the US National Science Foundation and US Department of Agriculture (INFEWS Grant No. EAR 1639318); the National Natural Science Foundation of China (Grant No. 72073003); and the China Ministry of Science and Technology and National Climate Center (Grant No: 2018YFC1509008 & E003931901). The views expressed are purely those of the authors and may not in any circumstances be regarded as stating an official position of the European Commission.
Data availability statement
All data that support the findings of this study are included within the article (and any supplementary files).
Footnotes
- 30
This broad category includes the paper and pulp sector, food and tobacco processing, industrial sources of fluorinated gases, and other generic industries.