Abstract
The impact of gas evolution on the electrochemical characteristics of planar electrodes and microwire array electrodes has been analyzed using modeling and simulation. The impacts can mainly be broken into three phenomena: a) a shift in the local reversible hydrogen electrode potential; b) hyperpolarization; and c) an increase in the solution resistance of the electrolyte. The local reversible hydrogen electrode potential shift was found to play the most important role, constituting >40% of the total potential drop between the cathode and reference electrode, following correction for cell resistance. Compared to planar electrodes, a microwire array structure reduces the impact of bubbles on the solution conductance, but the shift in the local reversible hydrogen electrode potential varies with distance from the actual electrode surface.
Export citation and abstract BibTeX RIS
A common physical characteristic of integrated solar-driven photoelectrochemical water-splitting systems is the continuous production of H2(g) in the cathodic chamber and O2(g) in the anodic chamber of the system. 1–3 Although substantial effort has been devoted to development and integration of the various materials involved in solar-driven water-splitting prototypes, including the light absorbers, catalysts, and membranes, relatively little attention has been given to the impact 1,2 of H2(g) and O2(g) bubbles on the performance of functional water-splitting constructs. 4,5
Recent experimental observations have been made of hydrogen or oxygen bubbles on either upward-facing or downward-facing electrodes and photoelectrodes in a planar or microwire array geometry. 6 Unlike a planar electrode, in which a single large bubble can deflect incident photons and can substantially reduce the area of the solid/liquid contact at the electrode surface, bubbles on microwire arrays can instead enhance mass transport, lead to a low ohmic resistance loss, promote forward optical scattering of incident illumination on upwards-facing electrodes, and generally improve the performance of microwire array photoelectrodes regardless of their orientation with respect to gravity. 6
The focus of this work is to present a theoretical underpinning of the behavior of gas bubbles in such systems and to quantify the impact of hydrogen bubbles on the electrochemical characteristics of such electrodes. We explored the effect of the bubbles on the overpotential of hydrogen evolving cathodes that utilize planar or microwire electrode morphologies. 1,2 Specifically, we analyzed the increase of the potential drop associated with different causes, i.e. ohmic losses, kinetic polarization, and shifts in the local reversible hydrogen potential, based on previous work by Leistra and Sides. 1 This work evaluates quantitatively the various impacts of bubbles on the electrode performance, and reveals that the shift in the local reversible hydrogen electrode potential is the most important component of the total potential drop derived from bubble production. The behavior of planar electrode systems has not yet been analyzed with regard to the overpotentials as a function of nominal current densities and electrode roughness. Our model provides a detailed and comprehensive understanding of the electrochemical behavior of such systems. We provide guidance for the future design of the electrode configuration, especially in cases where the extra potential drop produced by bubble formation must be considered.
Initially, the gases produced electrochemically are in a dissolved state in the liquid phase. Due to the constant production of these gases by the chemical reactions,


the liquid electrolyte rapidly becomes supersaturated, and consequently bubbles nucleate at cavities on the electrode surface. The bubbles keep growing on the electrode surface due to continuous electrolysis until the surface tension balances the buoyancy, at which point the bubble is released from the surface. Large bubbles are released from the electrode, and the system is then described by two-phase flow. Rising bubbles promote flow circulation inside the anodic or cathodic chamber, but hinder mass transport in the bulk electrolyte and can cause optical losses at gas-evolving photoelectrodes. 7 Bubbles can also reduce the contact between the liquid electrolyte and the electrode surface. 8,9 From formation to eventual bursting, the bubbles undergo nucleation, growth, detachment from the electrode surface, ascent through the electrolyte phase, and bursting at the electrolyte free surface. Each phase of the life cycle can be influenced by the design of the photoelectrochemical system including the wettability, roughness, structure and catalytic activity of the electrode surface.
The chemical reactions associated with the electrochemical kinetics; production of dissolved and dispersed gas; species transport within the bulk solution; and two-phase fluid flow have been studied separately. However, their interactions require a multi-physics framework to comprehensively understand the system associated with electrochemically induced gas evolution. Various mathematical models as well as experimental measurements have been developed and used for this purpose. The growth and release of bubbles at an electrode surface has been investigated using the volume of fluid (VOF) approach. 10 The physics of electrolytic gas evolution, including mass transfer and its effect on the electrical conductivity of bubble-containing electrolytes, has also been evaluated to provide insight into the relationship between the volume fraction of bubbles in the solution and the conductance of the electrolyte. 11 El-Askary et al. numerically simulated the hydrodynamic characteristics of electrolytic hydrogen evolution and confirmed the validity of the Euler-Euler model in two-phase bubble flow simulations. 12
The effects of bubble coverage have also been evaluated experimentally for non-planar electrode morphologies, including specifically the electrochemical and photoelectrochemical behavior of Si microwire arrays. 6 Under nonconcentrated sunlight, the photocurrent density for hydrogen evolution was 24 mA cm−2 with an optimized dual-junction tandem light absorber, and gas evolution had a minor impact on the resistance of the system. 13 However, under concentrated sunlight, the photocurrent densities can reach hundreds, even thousands of mA cm−2, and gas bubbles can occupy a large fraction of the volume of the bulk electrolyte. 14 An accurate description of the effects of gas bubbles in the design of such photoelectrochemical devices should assist efforts to increase the energy conversion efficiency through reducing the voltage drop in the cell, increasing the two-phase mass-transfer rate, and maintaining a large effective electrode surface area.
We report herein a quantitative analytical evaluation of the impact of hydrogen bubbles in the cathodic chamber of a 1.0 M H2SO4 electrolysis solution on the local reversible hydrogen electrode potential, the hyperpolarization, and the solution resistance of the electrolyte. These effects are compared to the sum of all of these bubble-associated potential drops under different nominal current densities and for electrodes having planar or microstructured morphologies as well as differing surface roughness characteristics. The bubble break-off radius has been parameterized as a function of the current density and the size of the surface cavities of the electrode. The size of the bubbles released from the electrode surface is crucial to calculate the two-phase mass transfer rates, the dissolved gas concentration at the electrode-electrolyte interface, and the shift in the local potential of the reversible hydrogen electrode.
The initial pH value has also been varied to investigate effects on the bubble-related ohmic drop in the bulk solution and on the contribution of the ohmic drop to the total potential drop in the system. Furthermore, two microwire electrode array configurations, 6 μm diameter and 14 μm center-to-center pitch, μW 6 ∣ 14, and 3μm diameter and 11 μm pitch, μW 3 ∣ 11, microwire arrays have been investigated to evaluate the effects of electrode morphology on the electrolyte conductance as a result of a reduction in the local current density and consequently a reduction in the bubble volume fraction in the bulk solution. 6 The dissolved gas concentration at the electrode-electrolyte interface has been evaluated and the shift in the local reversible hydrogen electrode potential at microwire array electrodes has been compared to the behavior of a planar electrode configuration having the same nominal current density and electrode surface roughness as the microwire array electrodes. The analysis provides a comprehensive understanding of the role of bubbles on the total potential drop in these photoelectrochemical systems, as well as of the relationship between the bubble properties and the nominal current density, electrode surface and electrode configuration.
Methods
This section explains how the electrochemical impact of the hydrogen gas in the cathodic chamber was evaluated analytically and computationally. The modeled system consisted of a bulk liquid-electrolyte domain evolving hydrogen gas (Fig. 1). Our work focuses on the area below the boundary layer. The diffusion layer is a thin liquid layer at the surface of the electrode. Beyond this diffusion layer, the solution is assumed to be well stirred and thus the concentration of all species was assumed to be uniform throughout the electrolyte. In the diffusion layer, mass transport occurs via both diffusion and migration. The thickness of the diffusion layer can vary typically between 100 and 10 μm depending on the rate of stirring. The upper value of 100 μm was utilized in this work. The green region in the illustration is the electrode, covered by a Pt catalyst (in purple). The orange circles represent the gaseous phase (H2), with H2 bubbles released from the electrode surface, rising in the liquid electrolyte (in light blue), and moving across the outer boundary layer. For the microwire configuration, some bubbles nucleate on the side walls of the wires, grow in size but get disturbed by the fluid nearby, and readily detach from the surface (see Fig. 1b). Other bubbles nucleate on the top of the wire, keep growing until the surface tension and buoyancy balance, and then detach (see Fig. 1c). When bubbles detach from the top of the wire, the break-off radius can be larger than the wire radius. The electrolytic evolution of hydrogen produces a two-phase fluid (gas and liquid) flow in the presence of mass transport between the phases, in addition to chemical reaction in the solution.
Figure 1. Schematic illustration of a cathodic gas-evolving chamber with: (a) a planar photoelectrode configuration, (b) a microwire array electrode configuration (bubbles nucleate on the side of the wire), and (c) a microwire array electrode configuration (bubbles nucleate on the top of the wire).
Download figure:
Standard image High-resolution imageSimulation of the bubble columns can be performed either by using a Eulerian-Eulerian (E–E) model, a Eulerian-Lagrangian (E–L) model or Direct Numerical Simulation (DNS). 15 An E–L model tracks each bubble individually using Newton's second law. Direct numerical simulation models are generally performed to obtain microscale data such as tracking the growth of the bubbles within the solution. DNS solves the Navier–Stokes equations without prior assumptions, therefore providing a high degree of accuracy but at great computational expense and thus can only be performed for a small number of bubbles. The DNS approach consequently is typically limited to treating micro-scale data. 15
The Eulerian-Eulerian E–E model is a general, macroscopic model for two-phase fluid flow. The E–E approach considers both phases as interoperating liquids and uses a continuum approach to the gas bubble and liquid phases. On the electrode-electrolyte interface the produced dissolved hydrogen rate and gaseous hydrogen rate over the surface area are averaged. 12 One velocity field is associated with each phase, and a momentum balance equation as well as a continuity equation describe the dynamics of each of the phases. The E–E model has been well developed for application to gas-evolving electrodes. For example, Liu et al. used the E–E model to simulate the electrochemical oxidation of p-methoxyphenol, and an E–E model was used by El-Askary et al. to model hydrogen production in an electrochemical cell. 12 Moreover, the E–E model is economic and does not consume an extraordinary amount of computation time. The E–E model is well suited for the systems of interest herein because the product gas, hydrogen, has a negligible density compared to that of the liquid. Moreover, the motion of the gas bubbles relative to the liquid is determined by a balance between viscous drag and pressure forces, and the gas phase and liquid phase share the same pressure field. 16
Governing equations
The E–E Model can be slightly simplified in the situation of interest, because the momentum and continuity equations for the two phases can be combined, while retaining a gas phase transport equation to track the volume fraction of the bubbles.
The momentum equation is:
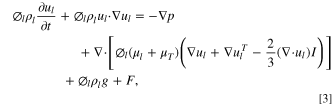
where u represents the velocity vector, p and ρ are the pressure and the density, respectively, ϕ is the phase volume fraction, μl is the dynamic viscosity of the liquid, and μT is the turbulent viscosity. The turbulent viscosity is neglected herein because the flows can be assumed to be laminar due to the low Reynolds numbers. The subscripts "l" and "g" denote quantities related to the liquid phase and gas phase, respectively.
The continuity equation is:

and the gas-phase transport equation is:

where mgl represents the rate of mass transfer the gas to the liquid. The gas velocity ug is calculated as the sum of the liquid phase velocity, ul , the relative velocity between the phases, uslip , and the drift velocity, udrift :

The drift velocity is associated with turbulence and thus was neglected in our treatment.
In the bulk electrolyte, the sum of the forces on the bubbles is composed of gravity, buoyancy, viscous drag force, shear-induced lift force, and virtual mass force. The virtual mass force accounts for the contribution of the changing volume of the bubbles. 17 A comparison of the size of the different terms allows for a simplifying assumption that the pressure forces on a bubble are balanced by the viscous drag force, fD , with the resulting simplified treatment commonly designated as the pressure-drag balance model:


where db represents the bubble diameter and Cd represents the viscous drag coefficient.
Hadamard and Rybczynski have proposed a model to calculate the drag coefficient for small spherical bubbles with a diameter less than 2 mm and with a bubble Reynolds number (Re,b) less than 1:


The gas density ρg is obtained using the ideal gas law:

where M is the molecular weight of the gas, R is the ideal gas constant (8.314 J mol−1 K−1), pref is a reference pressure, which in our work is 1 atm, and T is the absolute temperature. In this work, room temperature was taken to be T = 298.15 K.
For two-phase flow,

The mass transport rate from the gas phase to the liquid phase, mgl , can also be specified through two-film theory as:

where k represents the mass transfer coefficient, a is the interfacial area per unit volume, c is the local dissolved gas concentration, and c* is the equilibrium concentration of the gas dissolved in liquid, as given by:

where H is the Henry's law constant.
The interfacial area per volume can be calculated through the number density, n, and the volume fraction of gas, ϕg :

Simulation of the transport of electrolyte species was performed using the Nernst-Plank equation:

where Di , ci , zi and um,i are the diffusion coefficient, concentration, charge number, and mobility, respectively, of species i within the electrolyte. Because the Euler-Euler approach considers both phases as interoperating liquids and uses a continuum approach to the gas bubble and liquid phases, the diffusion coefficient of the dissolved hydrogen was decreased in the simulations to account for the impact of the existing bubbles. Specifically, the diffusion coefficient was multiplied by the volume fraction of bubbles in the solution. Ri indicates the reaction and mass transfer rate of species i in the electrolyte. The species of concern are dissolved hydrogen (H2), H+ , and OH−. For H+ and OH−, the reaction rate is calculated through water dissociation. For dissolved hydrogen, although no chemical reaction is involved, mass transfer rates are important due to the difference between the equilibrium concentration and the local dissolved gas concentration. The mass transfer rate is calculated through Eq. 13.
The value of um,i can be calculated by Nernst-Einstein relation:

The only chemical reaction considered herein was water dissociation:

The dissolved gas has an additional rate, which is related to the mass transfer rate, mgl :

Initial conditions and model input parameters
The electrolyte was assumed to be 0.1 M to 1.0 M H2SO4 that was saturated with dissolved hydrogen. The initial pressure in the solution was set as ρl gh + pref , where h is the distance from the outer boundary layer. The other parameters were the same as those specified by Glas and Westwater (Table I).
Table I. Kinematic and operating parameters used in the modeling.
Diffusion coefficient of H2, ![]() | 7.38 × 10−9 m2 s−1 |
Diffusion coefficient of H+, ![]() | 9.31 × 10−9 m2 s−1 |
Diffusion coefficient of OH−, ![]() | 5.26 × 10−9 m2 s−1 |
Surface tension, σ | 0.075 N m−1 |
Solution density, ρl | 1000 kg m−3 |
Hydrogen gas density, ρg | 0.09 kg m−3 |
Saturation concentration of H2 in solution, ![]() | 0.78 mM |
Operating temperature, T | 298.15 K |
Operating pressure, p | 1 atm |
Boundary conditions
Two-phase fluid model
At the electrolyte-electrode interface, a slip condition was assumed for the liquid phase, whereas the gas mass flux and the number density flux were fixed:

A slip condition was assumed for the liquid phase at the boundary, allowing for the (initial) tangential motion of the liquid but restricting the (initial) normal velocity to zero. At the other end of the bulk electrolyte layer, no slip condition was assumed for the liquid phase, and the gaseous phase was considered as the outlet. At the vertical wall, no slip condition was assumed for the liquid phase, whereas no flux was set for the dispersed phase. The no slip condition means that the fluid will have zero velocity relative to the boundary.
The break-off radius of the bubbles, Rr , is required to deduce the mass flux and the number density flux of the gas. Many factors determine Rr during electrolysis, including the electrolyte; surface roughness and wettability; applied current density; operating temperature and pressure; gas and liquid density; dissolved gas diffusion coefficient in the solution; saturation concentration of dissolved gas in the solution; and the surface tension between the gas and the electrode surface as well as between the gas and the solution. The value of Rr is also affected by disturbances due to adjacent bubbles, which can result in premature bubble departure from the electrode surface. Experimental observations indicate that the bubble break-off radius is primarily influenced by the applied nominal current densities and the roughness of the electrode. Data and analysis have been presented by Vogt describing the change in the break-off bubble radius as a function of the nominal current density for different electrode roughness factors. 18 In our work, this relationship was used to provide approximate parameterized values for Rr (Fig. 2).
Figure 2. Bubble break-off radius vs nominal current density based on experimental investigations of two different electrode surface cavity radii.
Download figure:
Standard image High-resolution imageThe bubble break-off radius can be related to the fractional bubble coverage on the electrode surface:

where Rf denotes the bubble radius at zero current as calculated in the Fritz equation, 18

The following two assumptions are inherent to this treatment:
- 1)Bubbles nucleate as a gas pocket in the cavities on the electrode, the electrode surface contains surface defects in the form of cavities. These cavities can be considered to be shaped geometrically as a conical void with a rounded bottom near the electrode surface that narrows to a sharp tip within the material. Rsite = 20 μm (radius of the flat surface of the conical void) represents a rougher surface than Rsite = 4 μm. Figure S5 (available online at stacks.iop.org/JES/169/066510/mmedia) presents a schematic illustration of the mechanism of gas bubble evolution at the electrode surface. Molecular diffusion governs the bubble growth, therefore, even if a conical cavity is close by, another bubble will not necessarily be generated and thus the number of the cavities on the electrode surface is not an important factor. According to Vogt, 18 the fractional bubble coverage can be estimated by parameterization using the bubble break-off radius and the bubble radius at zero current. This relationship is restricted to slow motion of the electrolyte liquid, which is in accord with the assumptions made herein. Scratches and pits on the electrode surface are the active nucleation sites for oxygen or hydrogen bubbles during the electrolysis process. 19
- 2)A force balance between buoyancy and surface tension is achieved when bubbles on the electrode reach the break-off radius.
The total amount of product gas transferred into the gaseous phase adhering to the electrode surface, fG , is determined by the fractional bubble coverage, Θ, of the electrode. At very small values of the current density (<1 mA cm−2) with nearly no bubbles adhering to the electrode surface, Θ → 0, and all the generated product gas crosses the electrolyte-electrode interface as dissolved gas, fG → 0. When the electrode surface is overcrowded by adhering bubbles, Θ → 1, and almost 100% of the dissolved gas is transferred into the gaseous phase, fG → 1. This relationship has been studied using various mathematical models, the outcomes of which do not differ substantially and can approximately be described by: 20–22

The gas mass flux and number density flux are then given through fG :


where J represents the nominal cathodic current density and Vg represents the volume of a single releasing bubble from the electrode surface.
Nucleation was treated in accord with conventional approaches used in modeling of bubbles on electrode surfaces. Observations of gas evolution on the microscopic scale reveal that the electrode surface is the site of frequent nucleation, growth, and detachment of bubbles. The total amount of the produced hydrogen through the electrochemical reaction transferred into the gaseous phase is determined by the fractional bubble coverage of the electrode, which can be estimated through the bubble break-off radius and the cavity/cone radius. 18 The effects of the bubbles on the overpotential of the cathode were determined by assessing the ohmic losses associated with physical obstruction of bubbles within the electrolyte, as well as the concentration overpotential and kinetics effects associated with increases the effective current density due to bubbles masking the electrode surface. 9
Transport of electrolyte species model
At the outer bulk electrolyte layer, constant concentrations of all electrolyte species at the initial conditions were assumed, due to the high convective fluxes beyond the boundary layer. At the electrode-electrolyte interface, the inward flux of H+ as well as dissolved hydrogen across the boundary can be specified as:


On the vertical wall, no flux for each species was assumed in the simulations.
In our work, electroneutrality has been used due to the high ionic strengths assumed. The electrode-electrolyte interface was taken as the reference boundary for the electrolyte potential.
The standard finite element method (FEM) solver in the COMSOL multi-physics package was used to model the two-phase flow as well as the electrochemical behavior. The maximum element size, the maximum element growth rate and curvature factor for this 2-D axisymmetric model were 1.3 μm, 1.08 and 0.25, respectively. For all simulations, a relative tolerance of the corresponding variable of 0.001 was applied as the convergence criterion.
Results
Figure 3 is the ideal current vs overpotential behavior for a planar Pt cathode surface, exhibiting a Tafel slope of 29 mV dec−1 in the absence of mass-transport limitations for the low overpotential region, based on experimental results. 23 "a" and "b" in Eq. 28 are both empirical Tafel parameters and were taken to be a = 89 mV and b = 29 mV, respectively.

where J is the nominal cathodic current density in A cm−2.
Figure 3. Ideal current density vs overpotential behavior of a planar Pt surface with a Tafel slope of 29 mV dec−1.
Download figure:
Standard image High-resolution imageDukovic and Tobias
24
pointed out the effects of bubbles attached to the surface of a gas-evolving electrode, with emphasis on the influence of bubbles on the local current distribution and on the potential drop at the electrode. The mathematical model accounts for the combined influence of (i) ohmic obstruction within the electrolyte, (ii) geometric masking of the electrode surface, which increases the overpotential by increasing the effective current density, and (iii) decreased local supersaturation, which decreases the concentration overpotential. Leistra and Sides use a similar approach.
9
The total bubble-derived potential drop difference between the cathode and the reference electrode, after correction for the cell resistance, is composed of which is the potential drop within the electrolyte; ηh
, which is the local concentration overpotential due to supersaturation of the electrolyte near the electrode with product gas and from depletion of the reaction ion at the electrode; and ηC
, which is the local surface overpotential for chemical reactions and/or charge-transfer reactions. Dukovic and Tobias also calculated the concentration distribution of dissolved gas in the boundary layer for a single bubble and calculated the supersaturation level of dissolved gas at the electrode surface vs the distance from the center of that attached bubble. Our work used the Euler-Euler approach to evaluate the impact of all the bubbles in the cathodic chamber on the electrochemical behavior of the entire cathode.
Impact of gas evolution on the electrolyte resistance
Based on the data in Fig. 2, when the radius of the surface cavity decreases, the break-off bubble radius decreases as well, resulting in an increase in the bubble volume fraction in the bulk electrolyte domain. For a planar electrode with a relatively rough surface Rsite = 20 μm, the generating bubble volume fraction within the 100 μm boundary layer varied from 0.74% to 12.7% as the nominal current density was varied from 100 to 300 mA cm−2 (Fig. 4). In contrast, for a planar electrode with surface cavity radius of 4 μm, the H2 gas volume fraction is 1.9% at a current density of 100 mA cm−2 but the generating bubble volume fraction increases to 57.8% when the current density increases to 300 mA cm−2 (Fig. 4a).
Figure 4. (a) Hydrogen gas bubble volume fraction (black lines with dots) in the bulk electrolyte and its impact on the solution conductivity (red lines with triangles); (b) the resulting ohmic drop at various current densities on a planar cathode, with two different values of the surface roughness and thus surface cavity radii.
Download figure:
Standard image High-resolution imageHydrogen bubbles block some of the pathways of ion transport within the solution, so the conductivity of the bulk electrolyte decreases as the bubble volume fraction increases. Maxwell's equation describes the relationship between the ratio of the conductance with dispersed a phase present to the conductance in the absence of the dispersed phase, Km , and the void fraction, fvoid , 25

Zhao et al. 25 summarized the numerical method to calculate the potential drop within the electrolyte. Maxwell's equation has been shown to fit the experimental data well when the volumetric fraction of gas adhering to the electrode surface bubble monolayer is less than 50%. 26 For a planar electrode with a surface cavity radius equal to 20 μm, Km decreases from 0.99 to 0.82 when the nominal current density is increased from 100 to 300 mA cm−2. Under such conditions, bubbles therefore do not have a substantial impact on the conductance of the electrolyte. In contrast, if the cavity radius on the electrode surface is 4 μm, Km is > 0.97 at a current density of 100 mA cm−2, but due to the high volume fraction of the hydrogen gas bubbles in the solution, Km decreases to 0.33 as the nominal current density increases to 300 mA cm−2.
Based on the calculated value of Km
, Eq. 29 can then be applied to evaluate the additional ohmic drop during gas evolution
9


where the second term on the right-hand side of Eq. 30 represents the ohmic drop in the absence of gas bubbles. represents the remaining ohmic drop after removal of this term, and κ is the solution conductivity, which can be defined as:
27

For a planar electrode with a surface cavity radius of 20 μm, is 0.036 mV at a nominal current density of 100 mA cm−2, with
increasing slightly to 2.12 mV at a current density of 300 mA cm−2 (Fig. 4b). In contrast, when the surface cavity radius is reduced to 4 μm,
is 0.095 mV at a current density of 100 mA cm−2, but
increases to 19.99 mV at a current density of 300 mA cm−2, primarily due to the substantial reduction in the conductance of the solution.
Impact of gas evolution on the local potential of the reversible hydrogen electrode
For a planar electrode system with a boundary layer of 100 μm and a surface cavity radius equal to 4 μm, the dissolved H2 concentration at the electrode-electrolyte interface decreases from 33.76 mM to 9 mM when the nominal current density increases from 100 mA cm−2 to 300 mA cm−2 (Fig. 5). At a surface cavity radius of 20 μm, the dissolved H2 concentration at the electrode-electrolyte interface initially increases as the nominal current density increases to 200–250 mA cm−2 and then deceases as the current density increases further. The Euler-Euler approach utilized considers both liquid and gas phases as interoperating liquids and uses a continuum approach to both phases, therefore, a single value was used to represent the surface concentration for the planar electrode configuration (average concentration on the surface).
Figure 5. Dissolved H2 concentration at the cathode/electrolyte interface (black dots and triangles) and the resulting shift in the local reversible hydrogen electrode potential (red dots and triangles) vs current density on a planar cathode having different electrode surface roughness values that produce different surface cavity radii.
Download figure:
Standard image High-resolution imageAn accumulation of dissolved H2 at the electrode-electrolyte interface will shift the local reversible hydrogen electrode potential in accord with the Nernst equation:

The value of ηC is calculated based on the dissolved H2 concentration at the electrode-electrolyte interface (Fig. 5). With a surface cavity radius equal to 4 μm, ηC decreases from 48 mV to 31 mV as the nominal current density increases from 10 to 300 mA cm−2. When the surface cavity radius increases to 20 μm, ηC reaches 49 mV at a current density of 100 mA cm−2, and ηC increases with the nominal current density to 55 mV at 250 mA cm−2 and then decreases as the applied current density increases further. The values of ηC and ηTafel have mutually the same order of magnitude and therefore ηC is a non-negligible term with regard to the total overpotential in the system.
Impact of gas evolution on the hyperpolarization
When electrode surfaces are covered with adhering hydrogen bubbles, an additional overpotential is produced due to an increase in effective current density at sites that are not covered by bubbles. This additional overpotential is given by:

where ηh represents the hyperpolarization. The value of ηh is determined by the Tafel behavior as well as by the ratio of the surface area A to the remaining active area A'. The value of A/A' is related to the fractional bubble coverage on the electrode surface, Θ (Fig. 6). The value of Θ increases as the nominal current density increases. When the surface cavity radius is 20 μm, at a nominal current density of 100 mA cm−2, Θ is 12.9%, but Θ is 36.8% at a current density of 300 mA cm−2. The fractional bubble coverage is not substantially affected by the surface cavity radius at the electrode, because the bubble departure radius and the Fritz radius are correlated. Hence, the surface roughness of the electrode does not substantially impact the hyperpolarization. The value of ηh varies slightly, from 1.4 mV to 5.9 mV, under nominal current densities between 100 mA cm−2 and 300 mA cm−2. The value of ηh is one order of magnitude less than ηTafel , so a change in ηh does not substantially affect the total overpotential in the cathodic side of the cell.
Figure 6. (a) Hydrogen gas bubble break-off radius (black dots and triangles) coverage (red dots and triangles) and (b) hyperpolarization vs current density on a planar cathode with two different surface cavity radii.
Download figure:
Standard image High-resolution imageTotal bubble related potential drop between the cathode and reference electrode
The total potential drop between the cathode and reference electrode, after correction for the cell resistance, can be expressed by Eq. 35:

Figure 7 shows that for a planar electrode with a surface cavity radius of 20 μm, the overpotential related to gas bubbles reaches 51 mV under a nominal current density of 100 mA cm−2, and under such conditions constitutes 46% of the total potential drop between the cathode and reference electrode. This overpotential increases as the nominal current density increases, and reaches 62 mV at 300 mA cm−2, but makes a slightly lower relative contribution of 45% to the total potential drop, because ηTafel increases logarithmically as the current density increases. The effect of the hydrogen bubbles in the bulk on the total potential drop, ηT , is not changed when the surface cavity radius decreases to 4 μm. With smaller cavities, bubbles have a larger influence on the solution conductance, especially at current densities > 200 mA cm−2, but their impact on the local reversible hydrogen electrode potential is smaller due to the lower dissolved H2 concentration at the electrode-electrolyte interface.
Figure 7. Percentage of total potential drop between the cathode and reference electrode associated with existing hydrogen gas bubbles as a function of current density on a planar cathode having two different surface cavity radii.
Download figure:
Standard image High-resolution imageEffects of gas evolution on the potential drop of microwire array electrodes
The computations described above indicate that for planar electrodes, the main impact of the hydrogen gas on the system resistance is due to the accumulation of dissolved H2 at the electrode-electrolyte interface. In this section, the behavior of microwire array electrodes is described and ηC is determined under the same nominal current densities as for the planar electrodes. A Pt catalyst was coated over the microwire array electrode surface (Figs. 1b and 1c). Array geometries having 6 μm diameter microwires on a 14 μm center-to-center pitch, μW 6 ∣ 14, and 3 μm diameter microwires on an 11 μm pitch, μW 3 ∣ 11, were investigated.
When the surface cavity radius is set at 4 μm under a nominal current density of 100 mA cm−2, the volume fraction of the gaseous hydrogen appears to be low near the bottom of the wires, and then increases along the z—axis. The H2 gas volume fraction in the solution is 1.9% for the planar electrode but is only 0.33% for the μW 6 ∣ 14 electrode. Due to the increased electrode surface area of the microwire array structure, at the same nominal current density, the local current density is lower than for the planar electrode, resulting in smaller break-off bubble radii and less dissolved hydrogen gas transferring into the bubbles. Hence, the H2 gas volume fraction in the solution is only 0.3% for μW 3 ∣ 11 array electrodes.
Lateral and radial concentrations gradients exist for the non-planar electrode configuration. Figure 8a indicates that the dissolved H2 concentration varies at different locations on the surface of the microwire array electrode. On the tops of the microwires, the dissolved H2 concentration is lower than on the planar electrode, leading to a smaller local shift in the reversible hydrogen electrode potential, ηC . On the sides of the microwires, the dissolved H2 concentration decreases from 39.69 mM near the bottom to 30.45 mM near the top of the microwires, and the calculated ηC consequently decreases from 50.42 mV to 47.02 mV. Under the same conditions, the dissolved H2 concentration at the electrode-electrolyte interface for the planar electrode is 33.76 mM and ηC is 48.35 mV. On the rest of the surface of a μW 6 ∣ 14 microwire array electrode, the dissolved H2 concentration is approximately 6 mM higher than for the planar configuration, increasing ηC by 2 mV.
Figure 8. Dissolved hydrogen concentration (black solid and dashed lines) (a) in the bulk electrolyte; (b) on the top end of the microwires; (c) on the surface of the microwire sides; and (d) on the remainder of the electrode surface, as well as the resulting shift in the local reversible hydrogen electrode potential (red solid and dashed lines) for a microwire array with 6 μm diameter and 14 μm center-to-center pitch under a nominal current density of 100 mA cm−2. The electrode surface cavity radius was 4 μm. The solid lines indicate the corresponding values for a planar electrode under the same conditions.
Download figure:
Standard image High-resolution imageFigure 9 indicates that the dissolved H2 at the electrode-electrolyte interface for a μW 3 ∣ 11 microwire array is very similar to that of a μW 6 ∣ 14 microwire array. Use of this microwire array instead of a planar electrode increases the solution conductance due to the lower bubble volume fraction, but does not substantially reduce the local shift in the reversible hydrogen electrode potential.
Figure 9. Dissolved hydrogen concentration (black solid and dashed lines) (a) in the bulk electrolyte; (b) on the top end of the microwires; (c) on the surface of the microwire sides; and (d) on the remainder of the electrode surface, as well as the resulting shift in the local reversible hydrogen electrode potential (red solid and dashed lines) for a microwire array with 3 μm diameter and 11 μm center-to-center pitch at a nominal current density of 100 mA cm−2. The electrode surface cavity radius was 4 μm. The solid lines indicate the corresponding values for a planar electrode under the same conditions.
Download figure:
Standard image High-resolution imageOn the μW 6 ∣ 14 microwire array, when the nominal current density increases to 150 mA cm−2, the bubble volume fraction in the electrolyte solution increases to 0.82%. At nominally the same current density on the planar electrode, the bubble volume fraction is 7.2%, so compared to the planar electrode the microwire array configuration reduces the bubble volume fraction by 9 times, whereas at a current density of 100 mA cm−2, the microwire array reduces the bubble volume fraction by 5.7 times. The microwire array configuration thus advantageously decreases losses associated with changes in the solution conductance as the nominal current density increases. Moreover, when the nominal current density increases from 100 mA cm−2 to 150 mA cm−2, the dissolved H2 concentration on the top end of the microwires increases by 12 mM, resulting in a 3 mV increase in ηC . In contrast with the planar electrode, the dissolved H2 concentration at the electrode-electrolyte interface is reduced very slightly, from 33.76 mM to 33.42 mM. The dissolved H2 accumulates even more on the other surface regions of the microwire array electrodes, because with a surface cavity radius of 4 μm and a nominal current density of 150 mA cm−2, the bubble break-off radius is 102 μm for the μW 6∣14 microwire array electrode, whereas the bubble break-off radius is only 48 μm for the planar electrode. This change leads to a much higher mass transfer rate of hydrogen from the liquid phase to the gas phase and substantially less accumulation of dissolved hydrogen at the electrode-electrolyte interface.
Impact of the electrolyte initial pH values
The initial H2SO4 concentration was 1 M for all the simulations discussed above. Based on Eq. 32, the solution conductivity will decrease linearly as the H2SO4 concentration decreases. For a given current density, the ohmic drop in the solution thus increases as the H2SO4 concentration decreases. For a planar electrode, the bubble related ohmic drop decreases linearly as the initial H2SO4 concentration is increased (Fig. 10). With the same boundary layer thickness, the limiting current density will also linearly decrease as the H2SO4 concentration is decreased. For example, with a boundary layer thickness of 100 μm, the limiting current density is 159 mA cm−2 for 0.5 M H2SO4, and is 318 mA cm−2 for 1 M H2SO4.
Figure 10. Ohmic drop due to bubbles as a function of H2SO4 concentration for a planar electrode system at a nominal current density of 150 mA cm−2.
Download figure:
Standard image High-resolution imageDiscussion
Impact of the surface roughness on the solution conductivity
At the same nominal current density, the bubble break-off radius is smaller on a smoother electrode surface (Fig. 2), which leads to more dissolved hydrogen converting into the gaseous form and consequently causes an increased gas volume fraction in the solution. Gaseous H2 will partially block the pathways for ion transport in the electrolyte, and thus result in an increase in the bubble associated ohmic drop. As shown in Fig. 4, the ohmic drop at a smoother electrode surface is six times higher than for a rough electrode surface under a nominal current density of 200 mA cm−2. Moreover, this difference becomes more pronounced as the nominal current density increases.
Impact of the increased nominal current densities on the dissolved hydrogen concentration
As shown in Fig. 5, for rough electrode surfaces, at low current densities the increased amount of dissolved H2 in the electrolyte is not large enough to compensate for the increasing dissolved H2 flow from the electrode. As a result, the dissolved H2 concentration at the electrode-electrolyte interface increases with increases in the nominal current density. In contrast, at current densities that produce a large amount of gaseous H2 bubbles from the dissolved H2 gas in the bulk, more dissolved hydrogen flows into the bulk solution, and the dissolved H2 concentration at the electrode-electrolyte interface decreases accordingly. For smooth electrode surfaces, the amount of dissolved hydrogen in the bulk is always larger than the hydrogen produced by the electrochemical reaction, so the dissolved H2 concentration at the electrode-electrolyte interface decreases as the nominal current density increases. An accumulation of dissolved H2 at the electrode-electrolyte interface will shift the local reversible hydrogen electrode potential, and based on Eq. 33, the local reversible hydrogen electrode potential shift follows the same trend as the dissolved H2 concentration at the electrode-electrolyte interface (Fig. 5).
Impact of the electrode geometry
As compared to a planar electrode, due to the decrease in the local current density, a microwire array electrode will reduce the bubble associated ohmic drop within the bulk solution but is not necessarily beneficial in terms of reducing the shift in the local reversible hydrogen electrode potential. The dissolved hydrogen flow from the bottom of the electrode and from the lateral area of the microwires will accumulate hydrogen, even though the local current density is lower than for the planar configuration. Consequently, the dissolved hydrogen concentration at the electrode-electrolyte interface will still increase, which will increase the local shift in the reversible hydrogen electrode potential.
Conclusions
The bubble related ohmic drop in the solution increases with the nominal current density and flatness of the electrode surface. With a surface cavity radius of 20 μm, even when the nominal current density reaches 300 mA cm−2, the bubble associated ohmic drop, does not constitute a substantial contribution to the total bubble related potential drop in the cathodic chamber. However, when the surface cavity radius is reduced to 4 μm, at nominal current densities in excess of 200 mA cm−2,
constitutes at least 10% of ηTafel
, due to the increased bubble volume fraction and reduced conductance in the solution. Decreasing the initial H2SO4 concentration would increase
and the solution conductance is proportional to the initial electrolyte concentration.
An accumulation of dissolved H2 at the electrode-electrolyte interface shifts the local reversible hydrogen electrode potential and represents the major contribution to the bubble associated potential drop between the cathode and reference electrode. When the electrode surface is relatively flat, with a cavity radius of 4 μm, the shift in the local reversible hydrogen electrode potential due to existing bubbles, ηC
, decreases with reductions in the nominal current density, due to the decreases in the dissolved H2 concentration at the electrode-electrolyte interface. In contrast, when the electrode surface is relatively rough, with a cavity radius equal to 20 μm, ηC
does not depend monotonically on changes in the nominal current density, due to shifts in the local reversible hydrogen electrode potential. For a planar electrode, at low current densities ηC
increases as the current density increases, and reaches a peak at 250 mA cm−2, gradually decreasing with further increases in current density. The shift in the local reversible hydrogen electrode potential constitutes at least 85% of the total increase in the potential drop associated with bubbles in the cathodic chamber. Bubbles adhering to the electrode surface will cause an additional overpotential increase due to the hyperpolarization, ηh
. However, when the nominal current density is less than 300 mA cm-2, ηh
contributes less than 10% of the total increase in potential drop related to bubbles in the cathodic chamber.
ηC
and ηh
produce the total additional potential drop between the cathode and reference electrode due to bubbles. For a planar electrode configuration, the sum of these terms is comparable to the ideal overpotential, ηTafel
, predicted for a planar Pt surface under the same current density, and is greater than 75% of ηTafel
.
Due to lower the bubble volume fraction in the electrolyte, microwire arrays can increase the solution conductance by decreasing the blockage of ion transfer pathways in the electrolyte. However, relative to a planar electrode at the same nominal current density, microwire array electrodes increase the accumulation of dissolved H2 on parts of the electrode surface and consequently increase in the shift in the local reversible hydrogen electrode potential, but these changes do not exceed an order of magnitude.
Acknowledgments
This work was supported in part by the U. S. Department of Energy, Office of Science, Office of Basic Energy Sciences, under Award number DE-SC0022087. This work was also supported in part by the Joint Center for Artificial Photosynthesis, a DOE Energy Innovation Hub, U. S. Department of Energy, Office of Science, Office of Basic Energy Sciences, under Award number DE-SC0004993. We are grateful to participate in this special issue to acknowledge on his 100th birthday the seminal contributions and extraordinary collegiality of Prof John Goodenough over decades of inspirational service to electrochemical societies, research, technology and electrochemists.