Abstract
This work demonstrates ultra-low power ozone sensors for real time, continuous, and portable monitoring. Atomic Layer Deposition (ALD) of SnO2 enables precise control of ultrathin film thickness on the order of the Debye length to enhance sensitivity at room temperature. Correlation between ozone concentration and the rate of resistance change is used to maintain fast response times and ultraviolet (UV) illumination hastens recovery. ALD SnO2 ultrathin film sensors realize room temperature operation with highly selective detection of 50 ppb ozone with average power consumption of 150 μW making them well suited for real time, portable environmental monitoring systems.
Export citation and abstract BibTeX RIS

This is an open access article distributed under the terms of the Creative Commons Attribution Non-Commercial No Derivatives 4.0 License (CC BY-NC-ND, http://creativecommons.org/licenses/by-nc-nd/4.0/), which permits non-commercial reuse, distribution, and reproduction in any medium, provided the original work is not changed in any way and is properly cited. For permission for commercial reuse, please email: oa@electrochem.org.
High levels of ozone (O3) have been shown to contribute to respiratory symptoms such as chronic cough, wheeze, and shortness of breath and chest colds with phlegm.1 Individuals suffering with respiratory diseases such as asthma are particularly sensitive to O3 which can trigger an asthma attack hours after exposure.2 According to the Environmental Protection Agency, O3 concentrations are higher near urban areas, highways and in general outside on hot sunny days highlighting the need for continuous, portable, real time monitoring of an individual's exposure levels in order to correlate personal health with surrounding environments. Sensors used for these applications must have high sensitivity, appropriate selectivity against other gases, low total power consumption, stability, accuracy, reliability and low cost. Among several sensing materials, SnO2 has been shown to be sensitive toward gases in a variety of papers however metal oxide sensors typically require high operating temperatures to achieve good sensitivity and fast recovery resulting in mW of power consumption making them unsuitable for portable monitoring.3–6 Several reports exist utilizing metal oxides at room temperature but they typically display response and recovery times greater than 10 minutes which also renders them unsuitable for real time monitoring.7–9
Our work utilizes Atomic Layer Deposition of SnO2 to tailor film thickness to twice the Debye length which has been shown to provide maximum sensitivity due to electron mobility modulation.10–12 We correlate the derivative of the response to ozone concentration and utilize ultraviolet light to compensate for slow response and recovery times, respectively. These methods enable detection of 10s of ppb of ozone with room temperature operation for average power consumption of just 150 μW. Additionally, our sensors demonstrate selectivity over interfering gases NO2 and CO at typical atmospheric levels making them good candidates for continuous, portable monitoring.
Experimental
The fabrication of sensor device started with 500nm thermal oxidation of Si (100) substrate for electrical isolation. After oxidation, the SnO2 sensing material was deposited in an ALD system (Cambridge Nanotech Savannah 100 model). Tetrakis(dimethylamino)tin precursor and O3 reactants were used to deposit SnO2 at 200°C. The films were then annealed at 400°C in N2 for 30 minutes to achieve a crystalline phase. Finally, interdigitated electrodes were defined using conventional UV lithography and lift off with 10 nm of titanium for an adhesion layer followed by 200 nm of gold.
The ALD film was characterized by aberration corrected Scanning Tunneling Electron Microscopy (STEM) with Energy Dispersive X-ray Spectrometry (EDS) to verify film thickness and elemental mapping and X-ray diffraction (XRD) to evaluate the crystallinity of the film.
Sensor response was measured in a custom built testing chamber to NO2 and CO using cylinder sources diluted with zero grade air and ozone generated from a NIST certified Teledyne T700U gas calibrator and ozone generator system. Sensor response in the form of resistance change was measured at room temperature in dry air using a Keithley 4200 semiconductor parameter analyzer to apply a voltage bias of 50 mV and measure current response from gas exposure.
Results and Discussion
Figure 1 illustrates the sensor structure. An optical image and cross-sectional schematic of fabricated sensor are shown with STEM image of ALD SnO2 on SiO2/Si substrate with EDS elemental mapping verifying the laminar stack structure and film thickness.
Figure 1. (Left) Optical image and 3D model of device structure and (right) STEM-EDS analysis of device stack.
Grazing angle X-ray diffraction patterns of the SnO2 film are shown in Figure 2. The As Deposited (AD) film appears amorphous or nanocrystalline but after annealing above 400°C for 30 minutes the film restructured to the rutile crystal phase as evidenced by the presence of the main (110) peak with other planes being visible as well.
Figure 2. Grazing angle XRD patterns for AD and annealed SnO2 films.
The fabricated sensors were tested for response to ozone in a humidity and temperature controlled testing chamber. All tests were performed at 23°C in dry air although preliminary data suggests that the sensor still displays strong response under 80% relative humidity conditions. Further exploration of temperature and humidity effects will be published at a later date. Figure 3 shows dynamic transient response of 7 nm thick ALD SnO2 sensor that was repeatedly exposed to 100 ppb of O3. Since SnO2 is an n-type semiconductor and ozone is oxidizing gas, the electrical resistance of the sensor is increased when exposed to ozone. It is noted that the testing was performed at room temperature to achieve low power consumption and only 125 nW of power is dissipated by the sensing film. Sensor recovery time is expected to be slow at low temperatures which we were able to overcome using ultraviolet illumination to desorb ozone molecules from the surface. With a 1% duty cycle of the UV LEDs we were able to reset the sensor resistance to the baseline value and the total power consumption including sensor power and LED power is 150 μW. The LEDs used emitted peak intensity of light with a wavelength of 385 nm and it was found that increasing the flux of light incident on the sensor resulted in a faster recovery. Optimized packaging will allow improved recovery efficiency and further reduce power levels.
Figure 3. The transient response of ALD SnO2 gas sensor repeatedly exposed to 100 ppb ozone measured at room temperature. UV LED was used to reset the sensor resistance to the base value.
It is known that the sensitivity and response time is related to the Debye length of the sensing material.10 The Debye Length can be calculated as
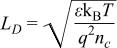
Where ε is the dielectric constant (=13.5 × 8.85 × 10−12 F/m in SnO210), kB is the Boltzmann's constant (=1.38 × 10−23 J/K), T is the temperature (K), q is the electric charge (=1.6 × 10−19 C) and nc is the carrier concentration at the channel. Ogawa, et al. determined nc to equal 3.6 × 1018 cm−3 at 250°C. This assumption yields ∼4.6 nm for twice the Debye Length. The temperature dependence above indicates a smaller length at room temperature however we can also assume that fewer carriers are available at room temperature extrapolating plots in Ogawa's work. Therefore, we hypothesize 7 nm ALD films to be well sized to maximize this effect enabling room temperature operation but further work is required to verify the responsible sensing mechanism and film thickness effect.
Figure 3 shows the transient response to various ozone concentrations at room temperature and it was obtained that the rate of change in resistance is dependent on ozone concentration. By evaluating the derivative of the resistance, the target gas concentration can be determined rapidly as shown in literature.14,15 Figure 4 shows the relationship between rate of change (dR/dt) and ozone concentration is approximately linear. We were able to achieve a fast response time of 100 seconds by this method.
Figure 4. Top dR/dt vs. time for 50, 75 and 100 ppb ozone exposure and (bottom) dR/dt vs concentration.
The stability of the sensor response is defined as the reproducibility or repeatability as a function of time. Figure 5 shows the stability of ALD SnO2 sensors where 100 ppb exposure cycles are repeated three times in four different tests showing consistent response. On the top plot in Figure 5 the measured resistance R is plotted vs. time while on the bottom the derivative (dR/dt) displays a consistent magnitude for the exposures.
Figure 5. (Top) Resistance plotted vs. time for 100 ppb ozone exposures and (bottom) dR/dt vs time.
Finally, Selectivity of the sensor was evaluated by exposing it to interfering gases CO and NO2 in typical atmospheric concentrations. In Figure 6, the sensor has been exposed to 50 ppm CO, 100 ppb NO2 and 100 ppb O3 with purges of clean air between the exposures. No response is registered during the CO and NO2 exposures but strong response is seen for the ozone exposure indicating good selectivity vs. these gases at atmospheric concentrations. This is due to ozone gas being much more reactive than the other species making it detectable at lower concentrations. Most reports in literature of NO2 detection using SnO2 indicate ppb level detection is challenging at room temperature.16 In the case of CO, measurement of gas in low ppm concentrations typically requires a noble metal catalyst or high temperature.14,17
Figure 6. Selectivity measurements exposing the sensor to 50 ppm CO, 100 ppb NO2 and 100 ppb O3.
Conclusions
In this paper we have reported on the fabrication of low power ultrathin gas sensors capable of detecting ozone concentrations of 50–100 ppb at room temperature. ALD technique enables a control of thin film thickness resulting in maximizing the sensor response at low operating temperature. Using duty cycled UV light for sensor recovery, average power consumption is held below 150 μW and the resistance derivative with respect to time is evaluated and correlated to the ozone concentration to maintain fast response times of 100 seconds. The ALD SnO2 sensor is very selective toward ozone over common interfering gases like CO or NO2 in the environment. This ALD SnO2 based sensor is feasible for wearable sensor system.
Acknowledgments
This work was funded by the National Science Foundation Nanoscience Engineering Research Center for Advanced Self-Powered Systems of Integrated Sensors and Technologies (EEC-1160483).