Application of the Nano-Drug Delivery System in Treatment of Cardiovascular Diseases
- 1The Eighth Affiliated Hospital, Sun Yat-sen University, Shenzhen, China
- 2Guangdong Provincial Key Laboratory of Tropical Disease Research, Department of Nutrition and Food Hygiene, School of Public Health, Southern Medical University, Guangzhou, China
Cardiovascular diseases (CVDs) have become a serious threat to human life and health. Though many drugs acting via different mechanism of action are available in the market as conventional formulations for the treatment of CVDs, they are still far from satisfactory due to poor water solubility, low biological efficacy, non-targeting, and drug resistance. Nano-drug delivery systems (NDDSs) provide a new drug delivery method for the treatment of CVDs with the development of nanotechnology, demonstrating great advantages in solving the above problems. Nevertheless, there are some problems about NDDSs need to be addressed, such as cytotoxicity. In this review, the types and targeting strategies of NDDSs were summarized, and the new research progress in the diagnosis and therapy of CVDs in recent years was reviewed. Future prospective for nano-carriers in drug delivery for CVDs includes gene therapy, in order to provide more ideas for the improvement of cardiovascular drugs. In addition, its safety was also discussed in the review.
Introduction
Cardiovascular diseases (CVDs) have become a serious worldwide public health problem, and the morbidity and mortality rank first above other diseases in the world (Gaurav et al., 2015). Faced with such a severe situation, developing drugs for the treatment of CVDs has become a top priority. Owing to the rapid development of nanoscience and outstanding performance of nanomaterials, nanotechnology has become a new solution to overcome the bottleneck of cardiovascular disease treatment. Nano-drug delivery systems (NDDSs) are a class of nanomaterials that have abilities to increase the stability and water solubility of drugs, prolong the cycle time, increase the uptake rate of target cells or tissues, and reduce enzyme degradation, thereby improve the safety and effectiveness of drugs (Quan et al., 2015; Gupta et al., 2019). NDDSs can be administered by various routes including inhalation, oral administration, or intravenous injection, remaining better bioavailability. In recent years, more scholars have started to develop nano-drug carrier system for the diagnosis and therapy of CVDs.
Additionally, as the application of nanomaterials increases, the exposure hazard of nanomaterials in clinical application also raises, resulting in the consequence that nanomaterials will have more opportunities to interact with blood vessels, blood, and their components, which will have an important impact on the human health. Therefore, this article mainly introduced the different types of NDDSs, their targeting strategies and application in CVDs, and the safety of nanomaterials was discussed as well.
Types of the NDDSs
NDDSs refer to material in which at least one dimension is in the range of nanometer scale (1–100 nm) or composed of them as basic units in three-dimensional space (Cooke and Atkins, 2016; Zhou et al., 2018). As an effective means to optimize the drug delivery, NDDSs have become a research hotspot in the field of pharmacy and modern biomedicine (Matoba et al., 2017). The investigation of NDDSs has been for more than 40 years, creating a mass of nano-drug carriers. According to the composition of the materials, the nanomaterials used in NDDSs can be divided into organic, inorganic and composite materials. The following is a description of several common NDDSs and their features (Figure 1, Table 1).
Liposomes
In general, liposomes are lipid vesicles formed by ordered phospholipid bilayer with cell-like structure (Landesman-Milo et al., 2013). As a type of drug carrier, liposomes show many advantages, such as non-toxic, non-immunogenicity, sustained-release drugs, prolonging drug action time, changing drug distribution in vivo, improving drug treatment index, reducing drug side effects, and so on (Yingchoncharoen et al., 2016). Liposomes can not only be easily developed for the entrapment of hydrophilic and ionic molecules, but compatible with hydrophobic drug (Chandrasekaran and King, 2014). Hydrophobic drugs can be surrounded by the bimolecular structure of phospholipids, and hydrophilic drugs, especially those containing genes, can be attached to the hydrophilic region of liposomes. The particle size, potential, and surface chemistry can be adjusted by modification of different lipid materials. Among various type of liposomes, cationic liposomes are positively charged, indicating that they may result in dose-dependent cytotoxicity and inflammatory responses, and as a kind of complexes, they may interact non-specifically with negatively charged serum proteins. Neutral lipids (Chapoy-Villanueva et al., 2015) and pH sensitive liposomes (Fan et al., 2017) are two ways to solve the above problems.
Polymer Micellar Co-delivery System
Polymer nanoparticles, another carriers for the delivery of drug, can be classified into non-biodegradable materials and biodegradable materials (Shi et al., 2019a,b). The synthetic polymer materials mainly include poly(lactic-co-glycolic acid) (PLGA), polyvinyl imine (PEI), polycaprolactone (PCL), polyvinyl alcohol (PVA), and so on (Danhier et al., 2012; Wei et al., 2018). These polymers exhibit biocompatibility, non-toxicity and no teratogenicity. Its degradation products, including oligomerization and final products, have no toxic effect on cells, and can coexist stably with most drugs. Natural polymers are mainly categorized into polysaccharides, peptides (Li et al., 2012), Chol and cyclodextrin inclusion complexes. Polymer nanoparticles usually formed by self-assembly of Amphiphilic block copolymers, are stable in the core and can be used to intercept insoluble drugs (Afsharzadeh et al., 2018). The stable structure of polymer nanoparticles is beneficial to the uniformity of particle size and the controlled release of drugs (Wang et al., 2011), and can effectively overcome the influence of gastrointestinal environment during oral administration. Their nanoscale and large surface area are conducive to uptake of drugs in cells and better bioavailability. Unfortunately, some polymer nanoparticles, have some drawbacks. For example, Chitosan, a natural polymer, is incompatible with biologic fluids, which can lead to particle degradation and reduce the working efficiency. Structural changes can be taken to solve its deficiency. Combining chitosan with polyethylene glycol, the conjugate has a unique endocytosis and macrophage phagocytosis mechanism (Yang et al., 2017). In addition, the modification of chitosan with a polypeptide can improve its working efficiency (Ping et al., 2017).
Dendritic Macromolecules
Macromolecules are synthetic, various-shaped and usually branched. Macromolecules shaped as sphere can be arranged in monodisperse space and mostly used as nano-carriers to be used for the administration and dissolution of insoluble targeted drugs. Dendritic macromolecules with unique branch structure, are also monodispersion and their molecular weight can be controlled. Besides, a large number of ready-made surface functional groups and hydrophobic environment are exist in the packaging, which make them an excellent drug delivery material (Kesharwani et al., 2012). Because of their excellent biological properties, dendritic macromolecules are widely used in biomedical and pharmaceutical fields, but the existence of surface cationic charge also limits their clinical application.
Metal Nanomaterials
The most commonly used metal nanomaterials are gold and silver nanomaterials, shaped in different structures that can be divided into/like nanoparticles, nanorods, nanocapsules, nanocuboid, and nanowire (Baeza et al., 2017). In addition to being used as nano-contrast agent for CT and surface-enhanced Raman spectroscopy, gold nanomaterials are also used in photothermal treatment of tumors and rheumatoid arthritis. As many studies shown, the application fields of silver nanomaterials mainly involved antibacterial, anti-infection and anti-tumor (Pietro et al., 2016). Moreover, some therapeutic drugs can be physically loaded into hollow gold or silver nanostructures (Liang et al., 2014), or chemically bonded to the surface of nanoparticles to achieve targeted delivery of the drugs. However, the removal of gold nanomaterials in human body is too slow, and the toxicity of silver ions in vivo limits the application of these metal nanomaterials in the treatment of chronic diseases.
Inorganic Non-metallic Nanomaterials
Inorganic non-metallic nanomaterials mainly include quantum dots, iron oxide, silicon, grapheme, and so on (Khafaji et al., 2019). Quantum dots (QDs), that is, semiconductor nanocrystals, are particularly focused on fluorescence imaging because of their unique luminous properties, while iron oxide nanoparticles are chiefly lay on the study of new MRI contrast agents (Jayagopal et al., 2009; Hauser et al., 2016; Su et al., 2017; Wei H. et al., 2017). Among them, mesoporous silicon nanomaterials have attracted more and more attention in the therapy of diseases in recent years due to its large surface area and porous structure (Wang W. et al., 2016). Those Inorganic nanomaterials can be used to improve the transport efficiency of drugs and genes in mammal cells through the integration of different functional groups. Meanwhile, they are suggested to be a kind of joint carrier with development potential. However, the bio-safety of inorganic non-metallic nanomaterials would be a considerable obstacle to their application in clinic (Perioli et al., 2019).
Composite Nanomaterials
In addition to the above nanomaterials, the preparation of composite nanomaterials with different properties is also under exploration in many studies. For example, metal or inorganic non-metallic nanomaterials are introduced into polymer or lipid nanomaterials to prepare multifunctional NDDSs containing both therapeutic drugs and contrast agents. Metal and inorganic nanomaterials are decorated or modified by organic materials to improve their physical and chemical properties, in vivo kinetic behavior and biocompatibility; and some NDDSs with special structure and diversified functions can be prepared by the combination of different metals and inorganic materials.
Targeting Strategy of the NDDSs
The targeted design of NDDSs focuses on the diagnosis and therapy of cancer in the early stages of development, but recent researches argued that lesion cells or tissues of CVDs can also be targeted, even easier to targeted than tumor tissues with multiple physiological barriers. Compared with conventional preparations, the metabolic time of nano-transporter drugs in the blood circulation may be prolonged. By regulating pH value (Gao et al., 2018; Yi et al., 2018), temperature (Wei L. et al., 2017), light (Ding et al., 2011), ultrasound or biological enzyme (Zhang et al., 2019), the rate of those targeted nano-transporter drugs can be controlled to function longer.
Passive Target Transfer
Enhanced Vascular Permeability
Passive targeted transport mainly utilizes high permeability and high retention (EPR) effects (Figure 2) (Holback and Yeo, 2011). EPR refers to the fact that some molecules or particles tend to accumulate in tumor tissues (Dinarvand et al., 2011). The microvascular endothelial cell space in normal tissue is dense and intact, and NDDSs loaded with drug, generally in high molecular weight, are not easy to pass through the vascular wall. The tumor tissue is rich in blood vessels and poor in structural integrity (Torchilin, 2011). Those drug-loaded NDDSs in high molecular weight can selectively pass through the vascular wall and remain in the tumor tissue. A large number of studies have shown that nano-drug carriers with particle size <100 nm can be located and targeted to solid tumor tissues by EPR. Compared with the direct administration method, the nano-drug carrier can increase the accumulation of the drug in the tumor tissue by more than 10 times, greatly improving the bioavailability (Maeda et al., 2013). But it is discovered that EPR effect can also be used in various CVDs, not only for tumors. In some course of CVDs, for example, the occurrence and development of AS is a chronic inflammatory process, where vascular permeability is often increased, which is very similar to that of solid tumors. Vascular endothelial permeability provides an effective means for NDDSs to deliver from the lumen side to the interior of the plaque. The nano-drug carriers entering the circulation are also ingested by inflammatory cells (monocytes or macrophages), and these drug-carrying cells migrate to plaque inflammation, allowing drugs to be delivered in another way (Flogel et al., 2008).
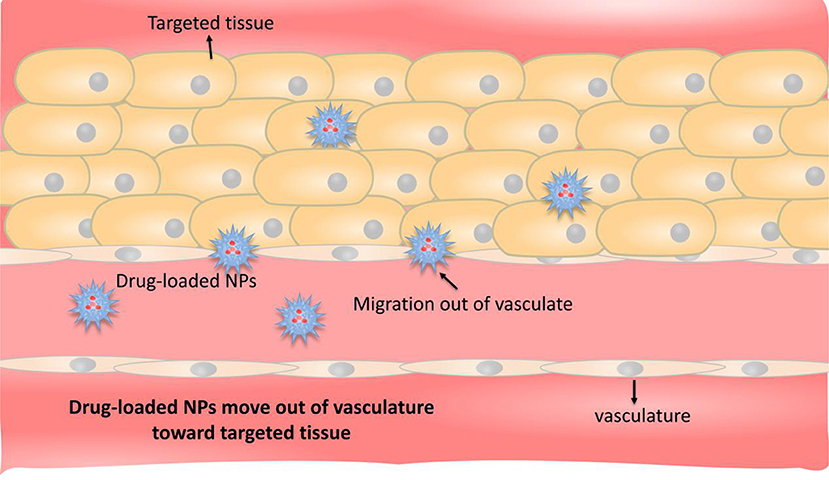
Figure 2. Schematic representation of passive targeting. The occurrence and development of CVDs are chronic inflammatory processes in which vascular permeability is usually increased, and nanoparticles of appropriate size pass directly through the blood vessels and release the drug at the site of the disease.
Due to the size and surface characteristics of a portion of nanomaterials, they are rapidly cleared in the blood during intravenous injection, making nanomaterials unsuitable for drugs that require long cycle times. In this case, nano-coating technology can be applied to the nano-system for certain concealment, and the rate of administration of the coating agent can be also controlled and adjusted. This technology is particularly suitable for NDDSs in the treatment of CVDs. Developers on NDDSs have employed poly (ethylene glycol) (PEG) in particle design. In fact, PEG is a flexible hydrophilic polymer that can form a hydrated layer when grafted onto the surface, effectively reducing the adsorption of proteins on the surface (Jokerst et al., 2011). The tissue plasminogen activator is encapsulated in the nanoparticles, making the nanosystem concealed in some degree, thus protecting the tissue plasminogen activator from inactivation by plasma inhibitors and prolonging the half-life (Hemmati and Ghaemy, 2016).
Shear-Induced Targeting
Studies have shown that as the intima grew outward (toward the lumen) in CVDs, such as advanced AS or myocardial infarction, thrombosis or microthrombus occurs, stenosis of the blood vessels follows and blood flow rate through the plaque increases, and thus the fluid shear force increases. The mean blood fluid shear force in the normal vasculature is <70 dyne·cm−2, while the blood fluid shear force in the AS plaque stenosis is up to 1,000 dyne·cm−2 (Korin et al., 2012). Therefore, the design of blood fluid shear-sensitive nanoparticles can achieve physicochemical targeting by utilizing the difference of blood fluid shear force between AS plaque and normal blood vessels. Holme et al. (2012) prepared a lenticular lipid nanoparticle vesicle with two sides convex. The drug-loaded nanometer can maintain structural stability in normal blood vessels, and the configuration change can be utilized to release the drug under the action of high blood fluid shear force through the blood circulation to the AS plaque. Inspired by the activation of platelets under the action of local high blood fluid shear forces in AS plaques and adhesion to plaque blood vessels, the researchers constructed a nanoparticle aggregate that can be assembled locally in plaques (Korin et al., 2012). First, the authors prepared PLGA nanoparticles with a particle size of about 180 nm and entrapped tissue plasminogen activator, and then obtained a PLGA nanoparticle aggregate with a particle size of 3.8 nm through spray drying. When the nanoparticles were exposed to the local high fluid shear stress of the AS plaque, they could be decomposed into 180 nm PLGA nanoparticles, and relied on the strong penetrability of the small particle size nanoparticles to enter the local thrombus of the plaque. The thrombolytic effect maximized the efficacy, significantly reduced the dose required for thrombolysis and the side effects of thrombolysis. In ischemic cardiomyopathy, the endothelial gap in ischemic myocardium widened, thus altering the shear of blood flow, and the concentration of polysaccharide from Ophiopogon japonicus in ischemic myocardium was twice as high as that in normal rats (Lin et al., 2010). Tan et al. found that both shear stress and blood flow shear rate of vascular wall could affect the aggregation of nanoparticles (Tan et al., 2011).
Magnetically Guided
Magnetically guided nanoparticle is an interesting “pseudo-passive” targeting method. Theoretically, the application of an external magnetic field can direct magnetic nanoparticles to the disease site (Prijic and Sersa, 2011). Recent evidence suggests that this strategy is beneficial for CVDs (Chandramouli et al., 2015). Alam et al. (2015) compared the effects of several nano drug carriers on atherosclerotic plaque imaging. Those Nanoparticles include iron oxide particles, superparamagnetic iron oxide nanoparticles, ultra-small superparamagnetic iron oxide nano-carrier, and very small superparamagnetic iron oxide nanoparticles. The results showed that the ultra-small superparamagnetic iron oxide nanoparticles have better vascular wall penetration ability and plaque retention than other groups. Some researchers have pointed out that the external magnetic field helps to transport particles from the cell-free layer which lacks red blood cells to the vessel wall (Freund and Shapiro, 2012).
Active Targeted Transhipment
On the basis of passive targeting, using the special pathological features of CVDs to develop an active targeting strategy for CVDs can improve the targeted delivery efficiency of drugs to the lesions of CVDs, which aroused researchers strong interest. Active targeting is primarily directed to functional modification of NDDSs with one or more targets to allow the drug to reach a particular site (Figure 3) (Matoba and Egashira, 2014). That is to say, introducing a functional group or active substance that specifically interacts with diseased tissues or cells into the surface of the nano-drug carrier will enhance carriers targeting (Lee et al., 2006; Gullotti and Yeo, 2009). Some active targets are discussed in detail below (Table 2).
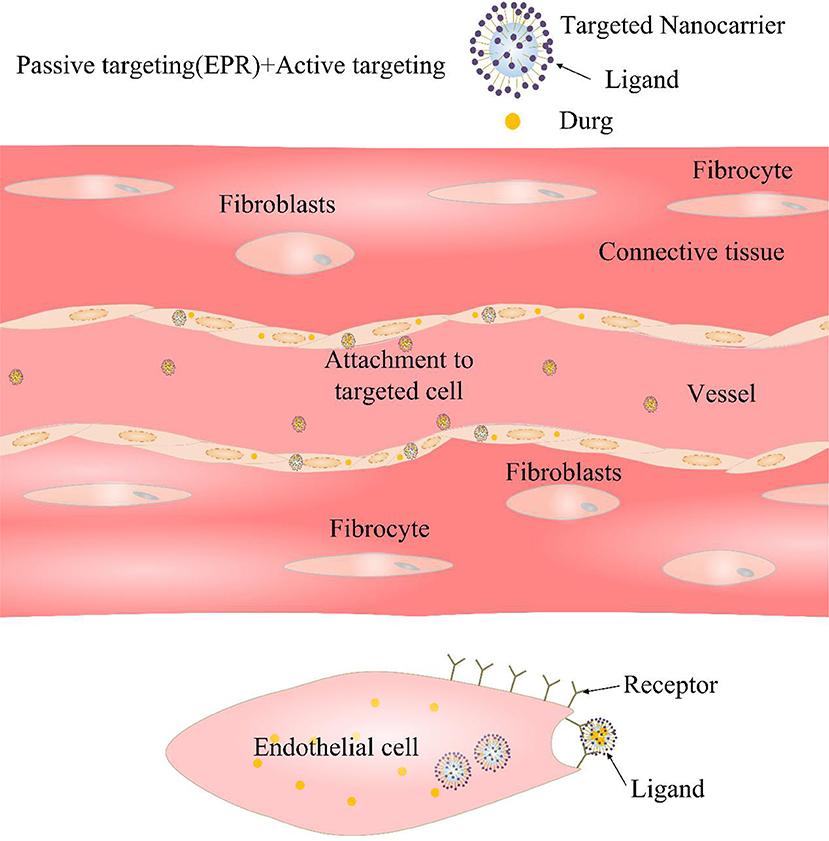
Figure 3. Diagrammatic sketch of active targeting. The surface of the nano-carrier is grafted with a targeting ligand, which is strongly bound to the selective cell surface by ligand-receptor binding.
Active Targeting of Vascular Endothelial Cells
At different stages of CVDs, vascular endothelial cells are in an inflammatory activation state. Compared with normal vascular endothelial cells, some small molecules including intercellular adhesion molecule-1 (ICAM-1), vascular adhesion molecule-1 (VCAM-1), integrins, selectins, and so on, are often overexpressed, which provides the active target for NDDSs (Glass and Witztum, 2001). It is showed that the conjugation of lung-targeted single-stranded variable fragment/liposome together with platelet endothelial cell adhesion molecule-1 (PECAM-1) antibody increases liposome transport to the pulmonary vascular system and strengthen its anti-inflammatory effects (Hood et al., 2018). In 2013, Yang et al. decorated the surface of silica nanoparticles with anti-VCAM-1 monoclonal antibody. The nanoparticles were able to bind to sites of inflammation before they were taken up by endothelial cells (Yang et al., 2013).
Based on the pathological features of high expression of ICAM-1 in early vascular endothelial cells of AS, Paulis et al. (2012) modified the antibody anti-ICAM-1 which actively targets ICAM-1 on the surface of liposomes and used it to load contrast agents (gadolinium). Studies have shown that the liposome could achieve the activated targeting of vascular endothelial cells and AS plaques through the specific action of anti-ICAM-1 and ICAM-1. However, competitive binding of circulating white blood cells to the ICAM-1 site and blood flow shearing could reduce the targeting function of liposomes to AS plaques. The authors optimized the binding degree of liposome to ICAM-1 by screening liposome particle size, antibody and lipid ratio, and obtained higher active targeting efficiency.
E-selectin is a surface glycoprotein of endothelial cells, which can promote the attachment of monocytes/macrophages and lymphocytes to induce inflammatory response, and eventually cause the occurrence and development of CVDs, such as AS (Ma et al., 2016). E-selectin can also be used as a target for nano-transport drugs. Functional liposomes carrying mouse H18/7 mAb (specific antibody to E-selectin) were used to act on interleukin (IL)-1β-activated human umbilical vein endothelial cells and non-interleukin (IL)-1β-activated human umbilical cord Vein endothelial cells. It was found that the ability of functional liposomes to target activated human umbilical vein endothelial cells is 275 times that of the non-activated type (Flaht-Zabost et al., 2014).
AT1 rises in myocardial when myocardial infarction or heart failure happened. Dvir et al. (2011) designed a polyethylene glycol liposomes (142 ± 8 nm), that could carry therapeutic payloads (such as growth factors, cytokines, etc.) and released them in a controlled manner. The ligand attached on these liposomes is a string of amino chain sequenced Gly-Asp-Arg-Val-Tyr-Ile-His-Pro-Phe (binding sequence of AT1 receptor), which could direct the nanoparticles to the infarction heart.
Active Targeting of Macrophages or Foam Cells
Macrophages or foam cells play a key role in the development of AS. In the early stage of AS, mononuclear/macrophages were recruited to activate vascular endothelial cells, and overexpressed some inflammation-related receptor molecules in an inflammatory environment, such as CD44 and interleukin-4 (IL-4) receptors, etc. Imaging and drug delivery for macrophages or foam cells using NDDSs will facilitate monitoring of disease progression and drug treatment in AS.
For example, Lee et al. (2015) linked 5β-cholic acid and fluorescent dye Cy5.5 to the carboxyl group of the HA skeleton by chemical bonding and formed nanoparticles (HA-NPs) by self-assembly. Compared with nanoparticles (HGC-NPs) constructed with chitosan backbones that did not target CD44 receptors, HA-NP could significantly increase the uptake of activated macrophages, and the plaque site of ApoE−/− mouse (AS model) was more targeted. Fluorescence co-localization studies indicated HA -NP was mainly distributed in macrophages in plaques.
Park et al. (2008) used phage library screening technology to optimize the amphiphilicity of the target IL-4 receptor peptide (CRKRLDRNC) which was modified on amphiphilic chitosan (with ethylene glycol chitosan as the backbone and 5β-cholate bonded) by chemical bond. Then nanoparticles with the function of targeted macrophages in AS plaque are obtained in a self-assembled method.
Targeting Vascular Basement Membrane Collagen
It has been reported that the vascular basement membrane of damaged blood vessels and inflammation sites is rich in collagen IV (Col IV) (Duner et al., 2015). In 2013, Kamaly et al. (2013) ligated the 7 amino acid oligopeptide molecule KLWVLPK (PLEA-β-PEG-Col IV) targeting collagen IV at the PEG end of the PLGA-β-PEG block copolymer and used it to package Act-26 (With anti-inflammatory and inhibition of leukocyte extravasation), thus nanoparticles (Ac2-26 Col IV NPs) targeting damaged blood vessels and collagen sites of inflammation sites were prepared. The results showed that Act-26 Col IV NPs reduced the migration and adhesion of neutrophils to the inflammation site and inhibited the development of inflammation. Further, in 2016, some researchers prepared nanoparticles (Col-IV IL-10 NPs) containing anti-inflammatory factor IL-10 by self-assembly using PLGA-p-PEG-Col IV and PDLA-PEG-OMe targeting collagen LV (Kamaly et al., 2016). After intravenous administration of Ldlr−/− mice, it was found that Col-IV IL-10 NP significantly increased the content of IL-10 in the plaque, and had better AS treatment effect than free IL-10.
In addition, multi-target nano-carriers with multiple inflammatory cell characteristics have been studied. PLNs incorporated these often ignored biophysical design criteria of platelet-mimetic discoid morphology and flexibility, then integrated these design parameters with the platelet-mimetic biochemical heteromultivalent interactive functions by dendritic presentation of multiple peptides that bind simultaneously to both activated natural platelets and injured endothelial sites (Anselmo et al., 2014).
Whether it is passive targeting or active targeting, the final targeting efficiency depends on the biological and physical properties of nanoparticles. The biological and physical properties includes particle size and distribution, targeting unit types, surface chemistry, morphology and density (Morachis et al., 2012). For the body, the development stage, type as well as location of CVDs and tumor, vascular wall shear rate, blood composition and its fluid type, together with other factors will greatly affect the targeting efficiency (Charoenphol et al., 2011). Although the application of active targeting NDDSs in clinical diagnosis and therapy is extremely attractive, its development is still facing great challenges. Those challenges are mainly reflected in two aspects: one is the limitation of the discovery of ideal target; the other is that there are still many bottleneck problems in the design and preparation of effective targeting nanosystem.
Multifunctional Responsiveness NDDSs
Multifunctional responsive NDDSs is a kind of drug carrier with better targeting ability, which is developed on the basis of the above two targeting modes of nano-drug carrier. In addition to having the previous targeting ability, this kind of carrier is generally composed of stimulating responsive materials, which can be released under the stimulation of the special environment of the focus site, thus reducing the release in the normal tissue and increasing the drug accumulation of the lesion tissue. At the same time, diagnostic molecules can be assembled or labeled on nano-carriers to compose an integrated diagnosis and therapy system.
Application of the NDDSs in the Diagnosis of CVDs
Early, rapid and accurate detection is important for effective prevention and treatment of CVDs. The application of molecular imaging in the diagnosis of CVDs has been paid more and more attention in recent years. In addition to the constant innovation of various imaging technologies, new contrast agents are the key to real-time, fast, high sensitivity and high resolution diagnostics. Compared with conventional contrast agents, nano-contrast agents have the following advantages: (1) in vivo stabilization, regulable distribution, and prolonging the half-life of contrast agents or drugs; (2) controllable physical and chemical properties (such as chemical composition, size) and imaging performance; (3) specific identification of certain biomolecules; (4) ability of multimodal imaging realization; (5) values in individualized diagnosis and therapy are expected to be realized (Attia et al., 2016). By designing specific nano-probes with the unique chemical signal molecules of diseased tissues determined by pathological studies, the contrast agent can be directed to the lesion area in the early stage of the disease for magnetic resonance imaging (MRI), X-ray imaging, fluorescence imaging, and contrast-enhanced ultrasound (US) imaging (Figure 4).
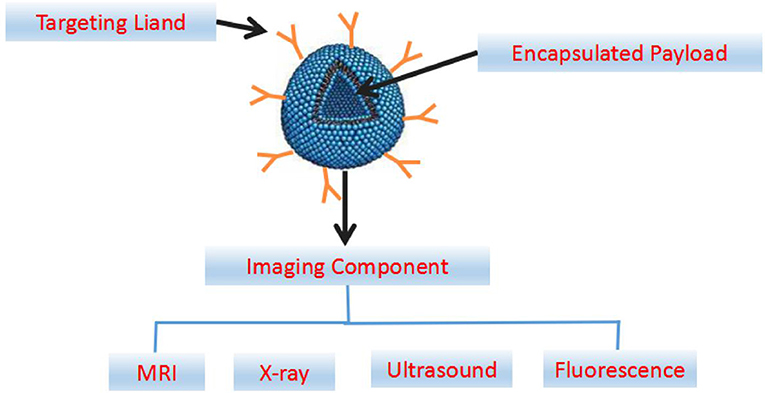
Figure 4. Abridged general view map of targeted nanoparticles engineered for imaging and drug delivery. The components of a multifunctional nanocarrier include a ligand for cellular targeting, and an encapsulated payload for delivery of the therapeutic agents. The imaging components can be incorporated in the interior payload, on the targeting ligand or associated with the nanoparticle shell.
Magnetic Resonance Imaging
In many imaging methods, magnetic resonance imaging is non-invasive, safe, and high resolution, and it is good for soft tissue imaging. However, the sensitivity of MRI is not high (10−3-10−9 M). The complexes of gadolinium commonly used in clinical practice are used as T1-weighted imaging contrast agents, and gadolinium has certain nephrotoxicity. Fe3O4 nanoparticles are considered to be non-toxic T2-weighted imaging contrast agents (Corot et al., 2006). Compared with tinctures, they have high sensitivity, good tissue compatibility and superparamagnetism (Kim et al., 2007). Targeted contrast agents are used to accumulate MRI probes at a sufficiently high concentration (in micrograms to milligrams) in the target tissue to achieve a high signal to noise ratio.
It is discovered that vascular imaging can be performed in the early stage of cardiovascular disease formation, and drugs can be administered for treatment after the magnetic nanoparticles are injected into the body. Yoo et al. (2016) loaded the hydrophilic lipid (amphiphilic) gadolinium chelating agent diethylenetriamine pentaacetic acid (DTPA) into a dendritic polymer and then wrapped it in the kernel of amphiphilic micelles and connected with fibrin binding agent. Thus, its targeting to atherosclerotic plaque was enhance, and can be used for early detection of thrombus. Winter et al. chose paramagnetic nanoparticles targeting integrin αvβ3 to inject intravenously into high fat fed New Zealand white rabbits to detect neovascularization in plaques in the early stage of AS (Winter et al., 2008).
X-Ray Imaging
Imaging with radionuclides plays a crucial role in the field of nuclear medicine (Mottu et al., 1999, 2002). Radionuclides are not only sensitive but also quantifiable. Positron emission tomography (PET) and single photon emission computed tomography (SPECT) are the most common types (Alie et al., 2015). At present, radionuclide-labeled nanomaterials can be used to monitor the embolization process and the distribution of nanomedicine to achieve targeted imaging (Mottu et al., 2002; Okamura et al., 2002; Torchilin, 2002; James et al., 2006). For example, the researchers used 186Re-BMEDA (Bao et al., 2003) and 99mTc-PEGylate-labeled (Bao et al., 2004) doxorubicin liposomes to perform SPECT, which can trace the distribution of drugs in the body, and also promote drug release. The nanoparticles can be used to detect the formation of atherosclerotic plaques by CT and to judge the prognosis as well. Galperin et al. injected iodine nanoparticles contrast agent (N1177) into mice via vein. It was found that the contrast agent gathered in macrophage rich tissue, and the signal of atheromatous plaques could be significantly enhanced, and the enhancement time could last for more than 30 min (Galperin et al., 2007). In 2016, Chhour et al. (2016), used 11 mercaptoundecanoic acid (11-MUDA) to encapsulate gold nanoparticles, found that gold nanoparticles could accumulate in foam cells of atherosclerotic plaques and increase the contrast of imaging.
Fluorescence Imaging
Optical imaging is a powerful imaging method with the advantages of no radiation, no invasion, high resolution and good controllability, but its penetration is poor. Fluorescence imaging is usually performed by using fluorescein to generate fluorescence signals. Near-infrared fluorescence (NIRF) probes are widely used because of their strong penetrating power and safety. They have been used in small animal living imaging systems and clinical tumor transformation. At present, a large number of nano-drug carriers, such as liposomes, metal, or non-metallic nanoparticles can enclose NIRF to achieve optical imaging of blood vessels (Weissleder and Ntziachristos, 2003; Setua et al., 2010; Sevick-Muraca, 2012). Its application in cardiovascular disease imaging has been paid more and more attention. McCarthy et al. bound the group of near infrared light activated therapeutic (NILAT) with macrophage-targeted magnetic nanoparticles(MNP) and prepared a kind of diagnostic and therapeutic nanoparticles (McCarthy et al., 2006). The experiment results shown that within 24 h of administration, the nanoparticles were reached in area. Wang Y. et al. (2016) injected profilin-1 magnetic iron oxide nanoparticles (PF1-Cy5.5-DMSA-Fe3O4-NPs) focusing on profilin-1 into the vein of atherosclerotic mice. It was found that the magnetic iron oxide nanoparticles were aggregated in carotid atherosclerotic plaques. There was a good correlation between the MRI signal of the animals injected with PC-NPs and the fluorescence intensity of NIRF imaging in vitro.
Ultrasound Imaging
Compared with fluorescence imaging, ultrasound imaging has natural advantages in medical imaging including safe, convenient, and real-time. Nano-ultrasound imaging materials that can be targeted to vascular-related markers have been developed. For example, vascular ultrasound nanoparticles that can be targeted to high expression of the vascular endothelial growth factor receptor 2 (VEGFR2) not only provide a more clear ultrasound imaging of tumor blood vessels, but also promote drug localization in blood vessels (Rojas et al., 2018). Marsh et al. had developed perfluorocarbon nanoparticles targeting blood fibrin, carrying the thrombus drug streptokinase for the diagnosis and therapy in thrombus (Marsh et al., 2007). The drug-loaded particles are synthesized by evaporation/dispersion technique with a diameter of about 250 nm and can be used for ultrasonic imaging.
Multi-Modal Bioimaging
At present, multi-modal imaging technology using a combination of different types of imaging methods can integrate different imaging methods to produce synergistic effects, providing more comprehensive and accurate image information for accurate diagnosis and precise treatment of CVDs. For instance, it was found that 64Cu-labeled SPIO-loaded doxorubicin nanoparticles could be used for MRI and PET (Yang et al., 2011). It has been reported that Cy5, sputum, and folic acid can be embedded in gold nanoparticles to achieve trimodal optical imaging, MRI and CT imaging in mice (Chen et al., 2016). This multimodal imaging and integration of diagnosis and treatment will be a new direction for the development of cardiovascular nanomedicine in the future.
Application of the NDDSs in the Treatment of CVDs
The NDDSs in AS
AS is the most common type of CVDs, often leading to a stroke or heart attack. The formation of AS begins with endothelial dysfunction. Plaque-induced coronary artery stenosis can cause ischemic cardiomyopathy, while plaque rupture can cause acute myocardial infarction (Nabel and Braunwald, 2012; Wall, 2013). Mechanisms of plaque instability include enhanced vascular permeability, Platelet endothelial cell adhesion molecules (PECAM) expression, macrophage aggregation, and expression of proteases, which can be targets for intervention. The drug can be delivered to atherosclerotic plaques by nano-drug carrier, to effectively prolong the half-life of drug plasma, increase the concentration of lesions and reduce side effects. The treatment strategies of these nano-drug carriers including regulating lipoprotein level, reducing the degree of inflammation, inhibiting of neovascularization, preventing coagulation, and so on (Table 3). These treatment strategies are used as interventions to development of AS, reduce plaque area or stabilize vulnerable plaques (Chetprayoon et al., 2015; Bejarano et al., 2018).
The NDDSs in Hypertension
At present, many kinds of drugs are applied for the treatment of hypertension, including angiotensin converting enzyme inhibitors, vascular angiotensin antagonists, central sympathetic nerve drugs, adrenergic receptor blockers, diuretics and vasodilators (Sharma et al., 2016). However, all these antihypertensive therapeutic drugs have obvious defects, including short plasma half-life, low bioavailability, toxic and side effects (upper respiratory tract abstraction, angioedema, reflex tachycardia, extreme hypotensive effect, and so on) (Alam et al., 2017; Martin et al., 2017; Niaz et al., 2017). Conversely, nano-drug carriers can provide prominent advantages mentioned above (Table 4) (Kimura et al., 2009). Some researchers have made olmesartan into a nanoemulsion system. Compared with the conventional dose, the nanoemulsion group has better blood pressure lowering effect, longer maintenance time, and can produce nearly three times the dose reduction (Alam et al., 2017).
The NDDSs in Pulmonary Hypertension
Pulmonary hypertension, a progressive highly dangerous disease, is characterized by increased pulmonary vascular resistance and elevated pulmonary artery pressure. Prostaglandin I, Endothelin receptor antagonist, type 5 phosphodiesterase inhibitor, etc. are common vasodilators for pulmonary hypertension. These vasodilators have shown some effects, but the overall therapeutic ability is limited. For solving this problem, nano-mediated drug delivery system has gradually become an important alternative strategy (Table 5). Bosentan is a selective and competitive Endothelin receptor antagonist, which is loaded into nanoparticles and has a solubility of seven times as much as that of unprocessed bosentan (Ghasemian et al., 2016).
The NDDSs in Myocardial Infarction
Reperfusion is mainly used in the early stage of myocardial infarction, but it can cause apoptosis, calcium overload and reactive oxygen species. These factors cause the opening of the mitochondrial membrane permeability transition pore (MPTP) and the increase of mitochondrial outer membrane permeability, thereby promoting cardiomyocyte apoptosis and necrosis (Hausenloy and Yellon, 2013). Clinically, the drug therapy for myocardial ischemia mainly depends on growth factors, cytokines and some small molecular compounds. These drugs have the same disadvantages of the above traditional drugs. The high permeability of blood vessels and enrichment of monocytes in ischemic myocardium can be harnessed to deliver drugs by targeting ability of nano-drug carriers (Table 6).
The NDDSs in Other CVDs
As a new drug delivery platform, nano-drug delivery system also performs well in other CVDs. Coronary artery allogeneic angiopathy is an inflammatory proliferation process that undermines the long-term success of heart transplantation. Lipid nanoparticles coated with methotrexate or paclitaxel were injected intravenously into rabbits which fed cholesterol-rich diet and received an ectopic heart transplant, both of which reduced macrophage infiltration in the graft (Barbieri et al., 2017). Myocardial ischemia is mainly due to the decrease of aortic perfusion in the heart, resulting in insufficient oxygen supply and unstable myocardial energy metabolism, thus forming a pathological state that cannot support the normal work of the heart. Liposomes coated with phenytoin (PHT, a non-selective VGSC inhibitor) were prepared by thin film dispersion. The results showed that PHT-encapsulated liposomes partially inhibited I/R injury-induced CD43+ inflammatory monocyte expansion and reduced infarct size and left ventricular fibrosis after intravenous injection of the rat myocardial I/R injury model (Zhou et al., 2013).
Vascular restenosis is the process of stenosis and obstruction after the interventional treatment of the blood vessels, such as angioplasty, arteriotomy, implantation of an endovascular stent, and so on (Wang et al., 2018). Some scientists (Banai et al., 2005; Kamath et al., 2006; Nakano et al., 2009; Schröder et al., 2018; Xi et al., 2018) have proposed that in the site of angioplasty, catheter-intervention techniques are used to infuse the drug-loaded nanoparticles into the injury site, enabling angioplasty, and topical administration in one step. The nanoparticles can enter the arterial wall through the damaged endothelium, localize, reside in and between cells, and then slowly release the drug (Wu et al., 2019). Therefore, the lesion vessel can be maintained at a relatively high concentration for a long period of time, which is beneficial to fully exerting the drug effect, and finally effectively prevents and treats vascular restenosis.
Application of the Co-loaded Nano-System in the CVDs
Drug combination therapy (including genes) is the treatment of two or more drugs to patients at the same time. In clinical practice, this therapy has been widely used for disease treatment. The purpose of this combination therapy is often due to the synergistic effect between drugs, or the therapeutic effect of multiple drugs is greater than that of a single drug. In recent years, many co-loaded nano-systems have been developed to carry common drugs and/or genes, especially siRNA to treat CVDs.
Application of RNAi in the Treatment of CVDs
RNA interference (RNAi) is a gene-specific silencing mechanism present in eukaryotic cells and an important measure for resisting foreign genes and infections during biological evolution. RNAi was first discovered in Caenorhabditis elegans (Braukmann et al., 2017), then in 2001, it was demonstrated to occur in mammalian cells (Lendeckel et al., 2001). RNA interference includes micro RNA (miRNA), small interfering RNA (siRNA), Piwi-interacting RNA, and long non-coding (lncRNA). RNAi technology, also known as gene silencing, introduces double-stranded RNA (dsRNA) consisting of sense and antisense RNAs corresponding to a certain mRNA sequence into cells, degrading mRNA homologously complementary thereto, and inhibiting the expression of cell-specific genes. The rapid development of RNAi research has driven it from experimental technology to therapeutic development tools (Katyayani et al., 2017), and RNAi has potential value in the treatment of CVDs (Kwekkeboom et al., 2014; Tadin-Strapps et al., 2015; Hoelscher et al., 2017). At the same time, RNA interference therapy also has challenges in the treatment of CVDs, including the toxicity, targeting, time-effect, and effective delivery system of RNA, which limits its widespread use in the clinic and is urgently needed to be solved and improved (Cotten et al., 1992; Sioud, 2015; Kasner et al., 2016; Navickas et al., 2016; Zhou et al., 2016). Table 7 indicated the future direction of cardiovascular RNA interference.
Co-loaded Gene and Drug Nano-System
For overcoming the problems in the delivery process and realizing the broad potential of RNAi-based therapeutics, safe and efficient nano delivery systems are needed. The apolipoprotein B (ApoB) siRNA was encapsulated into the liposome vector. After 48 h, the ApoB mRNA of the macaque liver decreased, and the maximum silencing rate exceeded 90%. ApoB protein, serum cholesterol, and low-density lipoprotein levels began to decrease 24 h after treatment and continued until day 11 (Zimmermann et al., 2006). Some researchers have used chitosan nanoparticles to construct and package small interfering RNA (siRNA) against PDGF-B mRNA expression vector, and then transfected into vascular smooth muscle cells (vSMC) of rabbit arterial wall damaged by balloon catheter, using therapeutic ultrasound for gene delivery. The results showed that the nanoparticles significantly inhibited the expressions of PCNA and PDGF-B mRNA in intimal vSMCs while the local intimal thickness and area were also reduced remarkably (Xia et al., 2013). Nox2-NADPH expression is significantly increased in the infarcted myocardium. Somasuntharam et al. (2013) demonstrated acid-degradable polyketal particles for Nox2-siRNA to the post-MI heart, which not only reduced siRNA degradation, but also inflammation.
Some pharmaceutical companies have developed new nano-dosages that deliver siRNA to the right cells at the right time (Hayden, 2014). Healthy volunteers (serum LDL levels of 3 mmol/L or higher) were injected intravenously with ALN-PCS or placebo developed by Alnylam Pharmaceuticals (Fitzgerald et al., 2014). ALN-PCS is a siRNA that inhibits the synthesis of PCSK9 and is assembled in lipid nanoparticles. PCSK9 protein in the human body cycle was reduced 70%, and LDL was reduced by 40% after intravenous injection of ALN-PCS.
The co-loaded gene and drug nano-system combined with nanotechnology and gene interference technology, the packaged substances have a synergistic effect, and the therapeutic effect is much better than the single treatment (Figure 5). Carvedilol, a kind of anti-hypertrophic drug that simultaneously blocks β-adrenergic receptors non-specifically in various organs, is widely used and effective. The non-specific genome-wide downregulation of p53 expression by specific siRNA efficiently abrogates cardiac hypertrophy. However, it can cause extensive tumorigenesis affecting bystander organs. Rana et al. (2015) encapsulated these bioactive molecules with stearic acid modified carboxymethyl chitosan (CMC) nanopolymers conjugated to a homing peptide for delivery in vivo to hypertrophied cardiomyocytes, resulted in effective regression of cardiac hypertrophy.
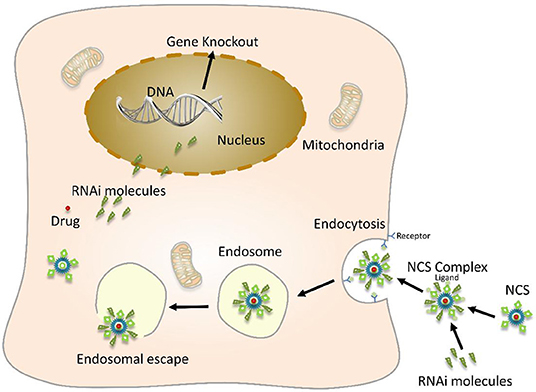
Figure 5. Schematic diagram of nanoparticles-mediated gene and drug delivery. Nano-carrier system (NCS) encapsulates or adsorbs a drug, a therapeutic foreign gene molecule on its surface, and also couples a specific targeting molecule to the surface of the nano-carrier and then binds to a cell surface-specific receptor through a targeting molecule. This enables safe and effective targeted genes and drug treatment.
Safety of the NDDSs
As the researches of nanomaterials go further, an increasing number of nanomaterials are prepared as NDDSs, but the unclear toxicity and the lack of systematically study of materials themselves restrict their further application. When the particle size enters the nanometer scale, it will show strong surface effect, small scale effect, quantum scale effect and macroscopic quantum tunneling effect (Gatoo et al., 2014).
Relatively few studies discuss about toxicity of NDDSs, particular in cardiovascular toxicity. But the tissue of cardiovascular system is considered to be the key site of NDDSs induced toxicity, which can produce great impact on the disease prognosis. Studies have revealed that nanomaterials could enter the blood circulation through respiratory tract, digestive tract, skin and other mucous membranes, and inevitably interacted with the blood system, immune system and other organs or tissues including plasma proteins and immune proteins, blood cells and immune cells, and so on.
The safety evaluation of the NDDSs is mainly focused on the toxicological study of the health effect. At present, the cardiovascular toxicity of nanomaterials based on animal and cell level shown that the toxicity was closely related to a series of undesirable effects induced by nanomaterials, including oxidative stress, inflammation apoptosis, blood aggregation and cardiac signal transduction (Donnini et al., 2000; Savic et al., 2003; Chen and Von, 2005; Qinghua et al., 2005). Among them, inflammatory reaction and oxidative stress are recognized as the main mechanisms of cardiovascular toxicity of nanomaterials.
Inflammatory response can affect the occurrence and development of CVDs including hypertension, myocarditis, AS, acute myocardial infarction and heart failure. Some researchers have found that if nano-carriers have not been removed in time, they could reach all organs through blood, stimulate the body to produce a series of inflammatory cytokines, and eventually lead to cytotoxicity, which increases the risk of cardiovascular events (Suwa et al., 2002).
Nanomaterials have a large number of surface atoms and are highly reactive, which can generate free radicals and stimulate the formation of ROS, thereby interfering with antioxidant systems (Chen and Von, 2005). Oxidative stress can induce oxidative damage to macromolecular substances, such as DNA and proteins, which leads to cell growth inhibition, cell cycle abnormalities, and cell death.
The study on the toxic mechanism of cardiovascular system damage caused by nanomaterials in global is still in its infancy. There is very few relevant research evidence on the biological endpoints to determine the relationship between the physicochemical parameters (shape, size, size distribution, surface structure, electrochemical properties, etc.) of the nanoparticles and the toxic effects of the cardiovascular system. Therefore, scientists need to carry out more researches on the cardiovascular system toxic effects and mechanisms of typical nanomaterial exposure, which can make better use of the positive effects of nanomaterials to prevent, reduce or eliminate the possible adverse effects on health. Furthermore, it would provide theoretical and technical basis for the establishment of nanomaterial safety evaluation technology and standards.
Summary and Perspective
In conclusion, the nano-carrier, as an efficient, specific and controllable intracellular drug delivery method, has shown unique advantages in the diagnosis and therapy of CVDs. It can effectively solve the problems of targeting, local drug delivery, controlled release, sustained release, and reducing toxicity while it is developing toward the multifunctional and integrated direction of diagnosis and therapy. With the innovation of nanotechnology and the deepening studies on molecular pathological mechanism of CVDs, the application of NDDSs will be promoted, and new techniques and methods will be provided for clinical diagnosis and therapy. In addition, since the study on these nano-carriers is in its infancy, many problems still remain unclear. The main challenge is how to solve the biocompatibility of nano-drug-loaded particles themselves or their degradation products, which is need to be solved in the field of nano-biomedicine in the future.
Author Contributions
WL proposed and developed the research outline, MY contributed to the concept and content framework, YD wrote the first draft and XZ prepared the drawings and contributed to improving the draft. HS and QH polished the article. ZW modified the format.
Funding
This review was supported by National Natural Science Foundation of China (Nos. 81972488, 81701836, 81973013), Guangdong Key R&D Program (No. 2019B020210002), Guangdong Natural Science Foundation (C1051164), The Eighth Affiliated Hospital of Sun Yat-sen University Outstanding Youth Reserve Talent Science Fund (FBJQ2019002).
Conflict of Interest
The authors declare that the research was conducted in the absence of any commercial or financial relationships that could be construed as a potential conflict of interest.
References
Afsharzadeh, M., Hashemi, M., Mokhtarzadeh, A., Abnous, K., and Ramezani, M. (2018). Recent advances in co-delivery systems based on polymeric nanoparticle for cancer treatment. Artif. Cells Nanomed. Biotechnol. 46, 1095–1110. doi: 10.1080/21691401.2017.1376675
Ahad, A., Aqil, M., Kohli, K., Sultana, Y., and Mujeeb, M. (2016). Nano vesicular lipid carriers of angiotensin II receptor blocker: anti-hypertensive and skin toxicity study in focus. Artif. Cells Nanomed. Biotechnol. 44, 1002–1007. doi: 10.3109/21691401.2015.1008509
Alam, S. R., Stirrat, C., Richards, J., Mirsadraee, S., Semple, S. I., Tse, G., et al. (2015). Vascular and plaque imaging with ultrasmall superparamagnetic particles of iron oxide. J. Cardiovasc. Magn. Reson. 17:83. doi: 10.1186/s12968-015-0183-4
Alam, T., Khan, S., Gaba, B., Haider, M. F., Baboota, S., and Ali, J. (2017). Nanocarriers as treatment modalities for hypertension. Drug Deliv. 24, 358–369. doi: 10.1080/10717544.2016.1255999
Alie, N., Eldib, M., Fayad, Z. A., and Mani, V. (2015). Inflammation, atherosclerosis, and coronary artery disease: PET/CT for the evaluation of atherosclerosis and inflammation. Clin. Med. Insights Cardiol. 8, 13–21. doi: 10.4137/CMC.S17063
Anselmo, A. C., Modery-Pawlowski, C. L., Menegatti, S., Kumar, S., Vogus, D. R., Tian, L. L., et al. (2014). Platelet-like nanoparticles: mimicking shape, flexibility, and surface biology of platelets to target vascular injuries. ACS Nano 8, 11243–11253. doi: 10.1021/nn503732m
Attia, M. F., Anton, N., Akasov, R., Chiper, M., Markvicheva, E., and Vandamme, T. F. (2016). Biodistribution and toxicity of X-ray iodinated contrast agent in nano-emulsions in function of their size. Pharm. Res. 33, 603–614. doi: 10.1007/s11095-015-1813-0
Baeza, A., Ruiz-Molina, D., and Vallet-Regi, M. (2017). Recent advances in porous nanoparticles for drug delivery in antitumoral applications: inorganic nanoparticles and nanoscale metal-organic frameworks. Expert Opin. Drug Deliv. 14, 783–796. doi: 10.1080/17425247.2016.1229298
Banai, S., Chorny, M., Gertz, S. D., Fishbein, I., Gao, J., Perez, L., et al. (2005). Locally delivered nanoencapsulated tyrphostin (AGL-2043) reduces neointima formation in balloon-injured rat carotid and stented porcine coronary arteries. Biomaterials 26, 451–461. doi: 10.1016/j.biomaterials.2004.02.040
Bao, A., Goins, B., Klipper, R., Negrete, G., and Phillips, W. T. (2003). 186Re-liposome labeling using 186Re-SNS/S complexes: in vitro stability, imaging, and biodistribution in rats. J. Nucl. Med. 44, 1992–1999. Available online at: http://apps.webofknowledge.com/full_record.do?product=UA&search_mode=GeneralSearch&qid=14&SID=5AxlSYBEVr5fqZXGOKg&page=1&doc=1
Bao, A., Goins, B., Klipper, R., Negrete, G., and Phillips, W. T. (2004). Direct 99mTc labeling of pegylated liposomal doxorubicin (Doxil) for pharmacokinetic and non-invasive imaging studies. J. Pharmacol. Exp. Ther. 308, 419–425. doi: 10.1124/jpet.103.059535
Barbieri, L. R., Lourenço-Filho, D. D., Tavares, E. R., Carvalho, P. O., Gutierrez, P. S., Maranhão, R. C., et al. (2017). Influence of drugs carried in lipid nanoparticles in coronary disease of rabbit transplanted heart. Ann. Thorac. Surg. 104, 577–583. doi: 10.1016/j.athoracsur.2016.12.044
Beck-Broichsitter, M., Kleimann, P., Gessler, T., Seeger, W., Kissel, T., and Schmehl, T. (2012). Nebulization performance of biodegradable sildenafil-loaded nanoparticles using the Aeroneb Pro: formulation aspects and nanoparticle stability to nebulization. Int. J. Pharm. 422, 398–408. doi: 10.1016/j.ijpharm.2011.10.012
Bejarano, J., Navarro-Marquez, M., Morales-Zavala, F., Morales, J. O., Garcia-Carvajal, I., Araya-Fuentes, E., et al. (2018). Nanoparticles for diagnosis and therapy of atherosclerosis and myocardial infarction: evolution toward prospective theranostic approaches. Theranostics 8, 4710–4732. doi: 10.7150/thno.26284
Braukmann, F., Jordan, D., and Miska, E. (2017). Artificial and natural RNA interactions between bacteria and C. elegans. RNA Biol. 14, 415–420. doi: 10.1080/15476286.2017.1297912
Cabrales, P., Han, G., Roche, C., Nacharaju, P., Friedman, A. J., and Friedman, J. M. (2010). Sustained release nitric oxide from long-lived circulating nanoparticles. Free Radic. Biol Med. 49, 530–538. doi: 10.1016/j.freeradbiomed.2010.04.034
Cagel, M., Tesan, F. C., Bernabeu, E., Salgueiro, M. J., Zubillaga, M. B., Moretton, M. A., et al. (2017). Polymeric mixed micelles as nanomedicines: achievements and perspectives. Eur. J. Pharm. Biopharm. 113, 211–228. doi: 10.1016/j.ejpb.2016.12.019
Chandramouli, S., Sanjana, S., and Swathi, S. (2015). Use of super paramagnetic iron-oxide nanoparticles in the treatment of atherosclerosis. IFMBE Proc. 46, 67–70. doi: 10.1007/978-3-319-11776-8_17
Chandrasekaran, S., and King, M. R. (2014). Microenvironment of tumor-draining lymph nodes: opportunities for liposome-based targeted therapy. Int. J. Mol. Sci. 15, 20209–20239. doi: 10.3390/ijms151120209
Chang, M. Y., Yang, Y. J., Chang, C. H., Tang, A. C., Liao, W. Y., Cheng, F. Y., et al. (2013). Functionalized nanoparticles provide early cardioprotection after acute myocardial infarction. J. Control Release 170, 287–294. doi: 10.1016/j.jconrel.2013.04.022
Chapoy-Villanueva, H., Martinez-Carlin, I., Lopez-Berestein, G., and Chavez-Reyes, A. (2015). Therapeutic silencing of HPV 16 E7 by systemic administration of siRNA-neutral DOPC nanoliposome in a murine cervical cancer model with obesity. J. Buon. 20, 1471–1479. Available online at: http://apps.webofknowledge.com/full_record.doproduct=UA&search_mode=GeneralSearch&qid=3&SID=5AxlSYBEVr5fqZXGOKg&page=1&doc=1
Charoenphol, P., Mocherla, S., Bouis, D., Namdee, K., Pinsky, D. J., and Eniola-Adefeso, O. (2011). Targeting therapeutics to the vascular wall in atherosclerosis–carrier size matters. Atherosclerosis 217, 364–370. doi: 10.1016/j.atherosclerosis.2011.04.016
Chen, J., Sun, Y., Chen, Q., Wang, L., Wang, S. H., Tang, Y., et al. (2016). Multifunctional gold nanocomposites designed for targeted CT/MR/optical trimodal imaging of human non-small cell lung cancer cells. Nanoscale 8, 13568–13573. doi: 10.1039/c6nr03143a
Chen, M., and Von, M. A. (2005). Formation of nucleoplasmic protein aggregates impairs nuclear function in response to SiO2 nanoparticles. Exp. Cell Res. 305, 51–62. doi: 10.1016/j.yexcr.2004.12.021
Chetprayoon, P., Matsusaki, M., and Akashi, M. (2015). Three-dimensional human arterial wall models for in vitro permeability assessment of drug and nanocarriers. Biochem. Biophys. Res. Commun. 456, 392–397. doi: 10.1016/j.bbrc.2014.11.094
Chhour, P., Naha, P. C., O'Neill, S. M., Litt, H. I., Reilly, M. P., Ferrari, V. A., et al. (2016). Labeling monocytes with gold nanoparticles to track their recruitment in atherosclerosis with computed tomography. Biomaterials 87, 93–103. doi: 10.1016/j.biomaterials.2016.02.009
Cooke, J. P., and Atkins, J. (2016). Nanotherapeutic solutions for cardiovascular disease. Methodist. Debakey Cardiovasc. J. 12, 132–133. doi: 10.14797/mdcj-12-3-132.
Corot, C., Robert, P., Idee, J. M., and Port, M. (2006). Recent advances in iron oxide nanocrystal technology for medical imaging. Adv. Drug Deliv. Rev. 58, 1471–1504. doi: 10.1016/j.addr.2006.09.013
Cotten, M., Wagner, E., Zatloukal, K., Phillips, S., Curiel, D. T., and Birnstiel, M. L. (1992). High-efficiency receptor-mediated delivery of small and large (48 kilobase gene constructs using the endosome-disruption activity of defective or chemically inactivated adenovirus particles. Proc. Natl. Acad. Sci. U.S.A. 89, 6094–6098. doi: 10.1073/pnas.89.13.6094
Danhier, F., Ansorena, E., Silva, J. M., Coco, R., Le Breton, A., and Preat, V. (2012). PLGA-based nanoparticles: an overview of biomedical applications. J. Control Release 161, 505–522. doi: 10.1016/j.jconrel.2012.01.043
Dinarvand, R., Sepehri, N., Manoochehri, S., Rouhani, H., and Atyabi, F. (2011). Polylactide-co-glycolide nanoparticles for controlled delivery of anticancer agents. Int. J. Nanomedicine 6, 877–895. doi: 10.2147/IJN.S18905
Ding, J., Zhuang, X., Xiao, C., Cheng, Y., Li, Z., He, C., et al. (2011). Preparation of photo-cross-linked pH-responsive polypeptide nanogels as potential carriers for controlled drug delivery. J. Mater. Chem. 21, 11383–11391. doi: 10.1039/C1JM10391A
Dongkyu, K., Jueun, H., Hyung-Ho, M., Hye Yeong, N., Hyejung, M., Hoon, J. J., et al. (2013). Anti-apoptotic cardioprotective effects of SHP-1 gene silencing against ischemia-reperfusion injury: use of deoxycholic acid-modified low molecular weight polyethyleneimine as a cardiac siRNA-carrier. J. Control Release 168, 125–134. doi: 10.1016/j.jconrel.2013.02.031
Donnini, D., Perrella, G., Stel, G., Ambesi-Impiombato, F. S., and Curcio, F. (2000). A new model of human aortic endothelial cells in vitro. Biochimie 82, 1107–1114. doi: 10.1016/s0300-9084(00)01195-0
Dou, Y., Chen, Y., Zhang, X., Xu, X., Chen, Y., Guo, J., et al. (2017). Non-proinflammatory and responsive nanoplatforms for targeted treatment of atherosclerosis. Biomaterials 143, 93–108. doi: 10.1016/j.biomaterials.2017.07.035
Dou, Y., Guo, J., Chen, Y., Han, S., Xu, X., Shi, Q., et al. (2016). Sustained delivery by a cyclodextrin material-based nanocarrier potentiates antiatherosclerotic activity of rapamycin via selectively inhibiting mTORC1 in mice. J. Control Release 235, 48–62. doi: 10.1016/j.jconrel.2016.05.049
Duner, P., Goncalves, I., Grufman, H., Edsfeldt, A., To, F., Nitulescu, M., et al. (2015). Increased aldehyde-modification of collagen type IV in symptomatic plaques–a possible cause of endothelial dysfunction. Atherosclerosis 240, 26–32. doi: 10.1016/j.atherosclerosis.2015.02.043
Duong, H. T., Dong, Z., Su, L., Boyer, C., George, J., Davis, T. P., et al. (2015). The use of nanoparticles to deliver nitric oxide to hepatic stellate cells for treating liver fibrosis and portal hypertension. Small 11, 2291–2304. doi: 10.1002/smll.201402870
Dvir, T., Bauer, M., Schroeder, A., Tsui, J. H., Anderson, D. G., Langer, R., et al. (2011). Nanoparticles targeting the infarcted heart. Nano. Lett. 11, 4411–4414. doi: 10.1021/nl2025882
Elsabahy, M., and Wooley, K. L. (2012). Design of polymeric nanoparticles for biomedical delivery applications. Chem. Soc. Rev. 41, 2545–2561. doi: 10.1039/c2cs15327k
Fan, Y., Chen, C., Huang, Y., Zhang, F., and Lin, G. (2017). Study of the pH-sensitive mechanism of tumor-targeting liposomes. Colloids Surf. B Biointerfaces 151, 19–25. doi: 10.1016/j.colsurfb.2016.11.042
Fitzgerald, K., Frank-Kamenetsky, M., Shulga-Morskaya, S., Liebow, A., Bettencourt, B. R., Sutherland, J. E., et al. (2014). Effect of an RNA interference drug on the synthesis of proprotein convertase subtilisin/kexin type 9 (PCSK9) and the concentration of serum LDL cholesterol in healthy volunteers: a randomised, single-blind, placebo-controlled, phase 1 trial. Lancet 383, 60–68. doi: 10.1016/S0140-6736(13)61914-5
Flaht-Zabost, A., Gula, G., Ciszek, B., Czarnowska, E., Jankowska-Steifer, E., Madej, M., et al. (2014). Cardiac mouse lymphatics: developmental and anatomical update. Anat. Rec. (Hoboken) 297, 1115–1130. doi: 10.1002/ar.22912
Flogel, U., Ding, Z., Hardung, H., Jander, S., Reichmann, G., Jacoby, C., et al. (2008). In vivo monitoring of inflammation after cardiac and cerebral ischemia by fluorine magnetic resonance imaging. Circulation 118, 140–148. doi: 10.1161/CIRCULATIONAHA.107.737890
Freund, J. B., and Shapiro, B. (2012). Transport of particles by magnetic forces and cellular blood flow in a model microvessel. Phys. Fluids 24:51904. doi: 10.1063/1.4718752
Galperin, A., Margel, D., Baniel, J., Dank, G., Biton, H., and Margel, S. (2007). Radiopaque iodinated polymeric nanoparticles for X-ray imaging applications. Biomaterials 28, 4461–4468. doi: 10.1016/j.biomaterials.2007.06.032
Gao, W., Hu, Y., Xu, L., Liu, M., Wu, H., and He, B. (2018). Dual pH and glucose sensitive gel gated mesoporous silica nanoparticles for drug delivery. Chin. Chem. Lett. 12, 1795–1798. doi: 10.1016/j.cclet.2018.05.022.
Gatoo, M. A., Naseem, S., Arfat, M. Y., Dar, A. M., Qasim, K., and Zubair, S. (2014). Physicochemical properties of nanomaterials: implication in associated toxic manifestations. Biomed. Res. Int. 2014:498420. doi: 10.1155/2014/498420
Gaurav, C., Saurav, B., Goutam, R., and Goyal, A. K. (2015). Nano-systems for advanced therapeutics and diagnosis of atherosclerosis. Curr. Pharm. Des. 21, 4498–4508. doi: 10.2174/1381612821666150917094215
Ghasemian, E., Motaghian, P., and Vatanara, A. (2016). D-optimal design for preparation and optimization of fast dissolving Bosentan nanosuspension. Adv. Pharm. Bull. 6:211. doi: 10.15171/apb.2016.029
Glass, C. K., and Witztum, J. L. (2001). Atherosclerosis. the road ahead. Cell 104, 503–516. doi: 10.1016/s0092-8674(01)00238-0
Gullotti, E., and Yeo, Y. (2009). Extracellularly activated nanocarriers: a new paradigm of tumor targeted drug delivery. Mol. Pharm. 6, 1041–1051. doi: 10.1021/mp900090z
Guo, T., Wang, J., Yang, J., Chen, W., An, G., Fan, L., et al. (2013). Lentivirus-mediated RNA interference of chymase increases the plaque stability in atherosclerosis in vivo. Exp. Mol. Pathol. 95, 51–56. doi: 10.1016/j.yexmp.2013.05.005
Gupta, N., Al-Saikhan, F. I., Patel, B., Rashid, J., and Ahsan, F. (2015). Fasudil and SOD packaged in peptide-studded-liposomes: properties, pharmacokinetics and ex-vivo targeting to isolated perfused rat lungs. Int. J. Pharm. 488, 33–43. doi: 10.1016/j.ijpharm.2015.04.031
Gupta, P., Garcia, E., Sarkar, A., Kapoor, S., Rafiq, K., Chand, H. S., et al. (2019). Nanoparticle based treatment for cardiovascular diseases. Cardiovasc. Hematol. Disord. Drug Targets 19, 33–44. doi: 10.2174/1871529X18666180508113253
Hausenloy, D. J., and Yellon, D. M. (2013). Myocardial ischemia-reperfusion injury: a neglected therapeutic target. J. Clin. Invest. 123, 92–100. doi: 10.1172/JCI62874
Hauser, A. K., Mitov, M. I., Daley, E. F., McGarry, R. C., Anderson, K. W., and Hilt, J. Z. (2016). Targeted iron oxide nanoparticles for the enhancement of radiation therapy. Biomaterials 105, 127–135. doi: 10.1016/j.biomaterials.2016.07.032
He, H., Yuan, Q., Bie, J., Wallace, R. L., Yannie, P. J., Wang, J., et al. (2018). Development of mannose functionalized dendrimeric nanoparticles for targeted delivery to macrophages: use of this platform to modulate atherosclerosis. Transl. Res. 193, 13–30. doi: 10.1016/j.trsl.2017.10.008
Hemmati, K., and Ghaemy, M. (2016). Synthesis of new thermo/pH sensitive drug delivery systems based on tragacanth gum polysaccharide. Int. J. Biol. Macromol. 87, 415–425. doi: 10.1016/j.ijbiomac.2016.03.005
Hoelscher, S. C., Doppler, S. A., Dreßen, M., Lahm, H., Lange, R., and Krane, M. (2017). MicroRNAs: pleiotropic players in congenital heart disease and regeneration. J. Thorac. Dis. 9, S64–S81. doi: 10.21037/jtd.2017.03.149
Holback, H., and Yeo, Y. (2011). Intratumoral drug delivery with nanoparticulate carriers. Pharm. Res. 28, 1819–1830. doi: 10.1007/s11095-010-0360-y
Holme, M. N., Fedotenko, I. A., Abegg, D., Althaus, J., Babel, L., Favarger, F., et al. (2012). Shear-stress sensitive lenticular vesicles for targeted drug delivery. Nat. Nanotechnol. 7, 536–543. doi: 10.1038/nnano.2012.84
Hood, E. D., Greineder, C. F., Shuvaeva, T., Walsh, L., Villa, C. H., and Muzykantov, V. R. (2018). Vascular targeting of radiolabeled liposomes with bio-orthogonally conjugated ligands: single chain fragments provide higher specificity than antibodies. Bioconjug. Chem. 29, 3626–3637. doi: 10.1021/acs.bioconjchem.8b00564
Hu, J., Sheng, Y., Shi, J., Yu, B., Yu, Z., and Liao, G. (2018). Long circulating polymeric nanoparticles for gene/drug delivery. Curr. Drug Metab. 19, 723–738. doi: 10.2174/1389200219666171207120643
Huang, M., and Wu, J. C. (2011). Molecular imaging of RNA interference therapy targeting PHD2 for treatment of myocardial ischemia. Methods Mol. Biol. 709, 211–221. doi: 10.1007/978-1-61737-982-6_13
Jain, A., and Jain, S. K. (2018). Stimuli-responsive smart liposomes in cancer targeting. Curr. Drug Targets 19, 259–270. doi: 10.2174/1389450117666160208144143
James, N. R., Philip, J., and Jayakrishnan, A. (2006). Polyurethanes with radiopaque properties. Biomaterials 27, 160–166. doi: 10.1016/j.biomaterials.2005.05.099
Jayagopal, A., Su, Y. R., Blakemore, J. L., Linton, M. F., Fazio, S., and Haselton, F. R. (2009). Quantum dot mediated imaging of atherosclerosis. Nanotechnology 20:165102. doi: 10.1088/0957-4484/20/16/165102
Jokerst, J. V., Lobovkina, T., Zare, R. N., and Gambhir, S. S. (2011). Nanoparticle PEGylation for imaging and therapy. Nanomedicine (Lond). 6, 715–728. doi: 10.2217/nnm.11.19
Kamaly, N., Fredman, G., Fojas, J. J., Subramanian, M., Choi, W. I., Zepeda, K., et al. (2016). Targeted interleukin-10 nanotherapeutics developed with a microfluidic chip enhance resolution of inflammation in advanced atherosclerosis. ACS Nano 10, 5280–5292. doi: 10.1021/acsnano.6b01114
Kamaly, N., Fredman, G., Subramanian, M., Gadde, S., and Farokhzad, O. C. (2013). Development and in vivo efficacy of targeted polymeric inflammation-resolving nanoparticles. Proc. Natl. Acad. Sci. U.S.A. 110, 6506–6511. doi: 10.1073/pnas.1303377110
Kamath, K. R., Barry, J. J., and Miller, K. M. (2006). The Taxus™ drug-eluting stent: a new paradigm in controlled drug delivery. Adv. Drug Deliver. Rev. 58, 412–436. doi: 10.1016/j.addr.2006.01.023
Kasner, M., Gast, M., Galuszka, O., Stroux, A., Rutschow, S., Wang, X., et al. (2016). Circulating exosomal microRNAs predict functional recovery after MitraClip repair of severe mitral regurgitation. Int. J. Cardiol. 215, 402–405. doi: 10.1016/j.ijcard.2016.04.018
Katyayani, T., Samaresh, S., Sushil, K., and Arun, I. (2017). siRNA delivery strategies: a comprehensive review of recent developments. Nanomaterials (Basel) 7:77. doi: 10.3390/nano7040077
Kesharwani, P., Gajbhiye, V., and Jain, N. K. (2012). A review of nanocarriers for the delivery of small interfering RNA. Biomaterials 33, 7138–7150. doi: 10.1016/j.biomaterials.2012.06.068
Khafaji, M., Zamani, M., Golizadeh, M., and Bavi, O. (2019). Inorganic nanomaterials for chemo/photothermal therapy: a promising horizon on effective cancer treatment. Biophys. Rev. 11, 335–352. doi: 10.1007/s12551-019-00532-3
Kim, E. H., Ahn, Y., and Lee, H. S. (2007). Biomedical applications of superparamagnetic iron oxide nanoparticles encapsulated within chitosan. J. Alloys Comp. 434–435:636. doi: 10.1016/j.jallcom.2006.08.311
Kimura, S., Egashira, K., Chen, L., Nakano, K., Iwata, E., Miyagawa, M., et al. (2009). Nanoparticle-mediated delivery of nuclear factor kappaB decoy into lungs ameliorates monocrotaline-induced pulmonary arterial hypertension. Hypertension 53, 877–883. doi: 10.1161/HYPERTENSIONAHA.108.121418
Korin, N., Kanapathipillai, M., Matthews, B. D., Crescente, M., Brill, A., Mammoto, T., et al. (2012). Shear-activated nanotherapeutics for drug targeting to obstructed blood vessels. Science 337, 738–742. doi: 10.1126/science.1217815
Kwekkeboom, R. F. J., Lei, Z., Doevendans, P. A., Musters, R. J. P., and Sluijter, J. P. G. (2014). Targeted delivery of miRNA therapeutics for cardiovascular diseases: opportunities and challenges. Clin. Sci. 127, 351–365. doi: 10.1042/CS20140005
Landesman-Milo, D., Goldsmith, M., Leviatan, B. S., Witenberg, B., Brown, E., Leibovitch, S., et al. (2013). Hyaluronan grafted lipid-based nanoparticles as RNAi carriers for cancer cells. Cancer Lett. 334, 221–227. doi: 10.1016/j.canlet.2012.08.024
Lee, D., Lockey, R., and Mohapatra, S. (2006). Folate receptor-mediated cancer cell specific gene delivery using folic acid-conjugated oligochitosans. J. Nanosci. Nanotechnol. 6, 2860–2866. doi: 10.1166/jnn.2006.465
Lee, G. Y., Kim, J. H., Choi, K. Y., Yoon, H. Y., Kim, K., Kwon, I. C., et al. (2015). Hyaluronic acid nanoparticles for active targeting atherosclerosis. Biomaterials 53, 341–348. doi: 10.1016/j.biomaterials.2015.02.089
Lee, Y., Pai, S. B., Bellamkonda, R. V., Thompson, D. H., and Singh, J. (2018). Cerivastatin nanoliposome as a potential disease modifying approach for the treatment of pulmonary arterial hypertension. J. Pharmacol. Exp. Ther. 366, 66–74. doi: 10.1124/jpet.118.247643
Lendeckel, W., Tuschl, T., Harborth, J., Weber, J. K., Yalçin, A. S., Elbashir, L. M., et al. (2001). Duplexes of 21-nucleotide RNAs mediate RNA interference in cultured mammalian cells. Nature 411, 494–498. doi: 10.1038/35078107
Li, Z., Ding, J., Xiao, C., Pan, H., Tang, Z., Xuan, P., et al. (2012). Glucose-sensitive polypeptide micelles for self-regulated insulin release at physiological pH. J. Mater. Chem. 22, 12319–12328. doi: 10.1039/c2jm31040f
Liang, J. J., Zhou, Y. Y., Wu, J., and Ding, Y. (2014). Gold nanoparticle-based drug delivery platform for antineoplastic chemotherapy. Curr. Drug Metab. 15, 620–631. doi: 10.2174/1389200215666140605131427
Lin, X., Wang, Z., Sun, G., Shen, L., Xu, D., and Feng, Y. (2010). A sensitive and specific HPGPC-FD method for the study of pharmacokinetics and tissue distribution of radix ophiopogonis polysaccharide in rats. Biomed. Chromatogr. 24, 820–825. doi: 10.1002/bmc.1369
Lobatto, M. E., Fayad, Z. A., Silvera, S., Vucic, E., Calcagno, C., Mani, V., et al. (2010). Multimodal clinical imaging to longitudinally assess a nanomedical anti-inflammatory treatment in experimental atherosclerosis. Mol. Pharm. 7, 2020–2029. doi: 10.1021/mp100309y
Ma, S., Tian, X. Y., Zhang, Y., Mu, C., Shen, H., Bismuth, J., et al. (2016). E-selectin-targeting delivery of microRNAs by microparticles ameliorates endothelial inflammation and atherosclerosis. Sci. Rep. 6:22910. doi: 10.1038/srep22910
Maeda, H., Nakamura, H., and Fang, J. (2013). The EPR effect for macromolecular drug delivery to solid tumors: improvement of tumor uptake, lowering of systemic toxicity, and distinct tumor imaging in vivo. Adv. Drug Deliv. Rev. 65, 71–79. doi: 10.1016/j.addr.2012.10.002
Majmudar, M. D., Keliher, E. J., Heidt, T., Leuschner, F., Truelove, J., Sena, B. F., et al. (2013). Monocyte-directed RNAi targeting CCR2 improves infarct healing in atherosclerosis-prone mice. Circulation 127, 2038–2046. doi: 10.1161/circulationaha.112.000116
Mansukhani, N. A., Peters, E. B., So, M. M., Albaghdadi, M. S., Wang, Z., Karver, M. R., et al. (2019). Peptide amphiphile supramolecular nanostructures as a targeted therapy for atherosclerosis. Macromol. Biosci. 19:e1900066. doi: 10.1002/mabi.201900066
Maranhao, R. C., Guido, M. C., de Lima, A. D., Tavares, E. R., Marques, A. F., Tavares, D. M. M., et al. (2017). Methotrexate carried in lipid core nanoparticles reduces myocardial infarction size and improves cardiac function in rats. Int. J. Nanomedicine 12, 3767–3784. doi: 10.2147/IJN.S129324
Marsh, J. N., Senpan, A., Hu, G., Scott, M. J., Gaffney, P. J., Wickline, S. A., et al. (2007). Fibrin-targeted perfluorocarbon nanoparticles for targeted thrombolysis. Nanomedicine (Lond). 2, 533–543. doi: 10.2217/17435889.2.4.533
Martin, G. V., Kassuha, D. E., and Manucha, W. (2017). Nanomedicine applied to cardiovascular diseases: latest developments. Ther. Adv. Cardiovasc. Dis. 11, 133–142. doi: 10.1177/1753944717692293
Matoba, T., and Egashira, K. (2014). Nanoparticle-mediated drug delivery system for cardiovascular disease. Int. Heart J. 55, 281–286. doi: 10.1536/ihj.14-150
Matoba, T., Koga, J. I., Nakano, K., Egashira, K., and Tsutsui, H. (2017). Nanoparticle-mediated drug delivery system for atherosclerotic cardiovascular disease. J. Cardiol. 70, 206–211. doi: 10.1016/j.jjcc.2017.03.005
McCarthy, J. R., Jaffer, F. A., and Weissleder, R. (2006). A macrophage-targeted theranostic nanoparticle for biomedical applications. Small 2, 983–987. doi: 10.1002/smll.200600139
Morachis, J. M., Mahmoud, E. A., and Almutairi, A. (2012). Physical and chemical strategies for therapeutic delivery by using polymeric nanoparticles. Pharmacol. Rev. 64, 505–519. doi: 10.1124/pr.111.005363
Mottu, F., Rufenacht, D. A., and Doelker, E. (1999). Radiopaque polymeric materials for medical applications. Current aspects of biomaterial research. Invest. Radiol. 34, 323–335. doi: 10.1097/00004424-199905000-00001
Mottu, F., Rüfenacht, D. A., Laurent, A., and Doelker, E. (2002). Iodine-containing cellulose mixed esters as radiopaque polymers for direct embolization of cerebral aneurysms and arteriovenous malformations. Biomaterials 23, 121–131. doi: 10.1016/s0142-9612(01)00087-4
Nabel, E. G., and Braunwald, E. (2012). A tale of coronary artery disease and myocardial infarction. N. Engl. J. Med. 366, 54–63. doi: 10.1056/NEJMra1112570
Nafee, N., Makled, S., and Boraie, N. (2018). Nanostructured lipid carriers versus solid lipid nanoparticles for the potential treatment of pulmonary hypertension via nebulization. Eur. J. Pharm. Sci. 125, 151–162. doi: 10.1016/j.ejps.2018.10.003
Nakano, K., Egashira, K., Masuda, S., Funakoshi, K., Zhao, G., Kimura, S., et al. (2009). Formulation of nanoparticle-eluting stents by a cationic electrodeposition coating technology: efficient nano-drug delivery via bioabsorbable polymeric nanoparticle-eluting stents in porcine coronary arteries. JACC Cardiovasc. Interv. 2, 277–283. doi: 10.1016/j.jcin.2008.08.023
Navickas, R., Gal, D., Laucevi Ius, A., Taparauskait, A., Zdanyt, M., and Holvoet, P. (2016). Identifying circulating microRNAs as biomarkers of cardiovascular disease: a systematic review. Cardiovasc. Res. 111, 322–337. doi: 10.1093/cvr/cvw174
Niaz, T., Hafeez, Z., and Imran, M. (2017). Prospectives of antihypertensive nano-ceuticals as alternative therapeutics. Curr. Drug Targets 18, 1269–1280. doi: 10.2174/1389450117666160711163119
Niaz, T., Shabbir, S., Manzoor, S., Rehman, A., Rahman, A., Nasir, H., et al. (2016). Antihypertensive nano-ceuticales based on chitosan biopolymer: physico-chemical evaluation and release kinetics. Carbohydr. Polym. 142, 268–274. doi: 10.1016/j.carbpol.2016.01.047
Oduk, Y., Zhu, W., Kannappan, R., Zhao, M., Borovjagin, A. V., Oparil, S., et al. (2018). VEGF nanoparticles repair the heart after myocardial infarction. Am. J. Physiol. Heart Circ. Physiol. 314, H278–H284. doi: 10.1152/ajpheart.00471.2017
Okamura, M., Yamanobe, T., Arai, T., Uehara, H., Komoto, T., Hosoi, S., et al. (2002). Synthesis and properties of radiopaque polymer hydrogels II: copolymers of 2,4,6-triiodophenyl- or N-(3-carboxy-2,4,6-triiodophenyl)- acrylamide and p-styrene sulfonate. J. Mol. Struct. 602, 17–28. doi: 10.1016/S0022-2860(01)00710-4
Park, K., Hong, H., Moon, H. J., Lee, B., Kim, I., Kwon, I. C., et al. (2008). A new atherosclerotic lesion probe based on hydrophobically modified chitosan nanoparticles functionalized by the atherosclerotic plaque targeted peptides. J. Control Release 128, 217–223. doi: 10.1016/j.jconrel.2008.03.019
Paulis, L. E., Jacobs, I., and van den Akker, N. M. (2012). Targeting of ICAM-1 on vascular endothelium under static and shear stress conditions using a liposomal Gd-based MRI contrast agent. J. Nanobiotechnol. 10:25. doi: 10.1186/1477-3155-10-25
Pechanova, O., Barta, A., Koneracka, M., Zavisova, V., Kubovcikova, M., Klimentova, J., et al. (2019). Protective effects of nanoparticle-loaded aliskiren on cardiovascular system in spontaneously hypertensive rats. Molecules 24:2710. doi: 10.3390/molecules24152710
Perioli, L., Pagano, C., and Ceccarini, M. R. (2019). Current highlights about the safety of inorganic nanomaterials in healthcare. Curr. Med. Chem. 26, 2147–2165. doi: 10.2174/0929867325666180723121804
Pietro, P. D., Strano, G., Zuccarello, L., and Satriano, C. (2016). Gold and silver nanoparticles for applications in theranostics. Curr. Top Med. Chem. 16, 3069–3102. doi: 10.2174/1568026616666160715163346
Ping, S., Wei, H., Lin, K., Mingji, J., Bo, F., Hongyan, J., et al. (2017). siRNA-loaded poly(histidine-arginine)6-modified chitosan nanoparticle with enhanced cell-penetrating and endosomal escape capacities for suppressing breast tumor metastasis. Int. J. Nanomed. 12, 3221–3234. doi: 10.2147/IJN.S129436
Prijic, S., and Sersa, G. (2011). Magnetic nanoparticles as targeted delivery systems in oncology. Radiol. Oncol. 45, 1–16. doi: 10.2478/v10019-011-0001-z
Qinghua, S., Aixia, W., Ximei, J., Alex, N., Damon, D., Brook, R. D., et al. (2005). Long-term air pollution exposure and acceleration of atherosclerosis and vascular inflammation in an animal model. JAMA. 294:3003. doi: 10.1001/jama.294.23.3003
Quan, X., Rang, L., Yin, X., Jin, Z., and Gao, Z. (2015). Synthesis of PEGylated hyaluronic acid for loading dichloro(1,2-diaminocyclohexane)platinum(II) (DACHPt) in nanoparticles for cancer treatment. Chin. Chem. Lett. 26, 695–699. doi: 10.1016/j.cclet.2015.04.024
Qumbar, M., Ameeduzzafar, Imam, S. S., Ali, J., Ahmad, J., and Ali, A. (2017). Formulation and optimization of lacidipine loaded niosomal gel for transdermal delivery: in-vitro characterization and in-vivo activity. Biomed. Pharmacother. 93, 255–266. doi: 10.1016/j.biopha.2017.06.043
Rana, S., Datta, K., Reddy, T. L., Chatterjee, E., Sen, P., Pal-Bhadra, M., et al. (2015). A spatio-temporal cardiomyocyte targeted vector system for efficient delivery of therapeutic payloads to regress cardiac hypertrophy abating bystander effect. J. Control Release 200, 167–178. doi: 10.1016/j.jconrel.2015.01.008
Rojas, J. D., Fanglue, L., Yun-Chen, C., Anna, C., Chong, C. D., Bautch, V. L., et al. (2018). Ultrasound molecular imaging of VEGFR-2 in clear-cell renal cell carcinoma tracks disease response to antiangiogenic and notch-inhibition therapy. Theranostics 8, 141–155. doi: 10.7150/thno.19658
Savic, R., Luo, L., Eisenberg, A., and Maysinger, D. (2003). Micellar nanocontainers distribute to defined cytoplasmic organelles. Science 300, 615–618. doi: 10.1126/science.1078192
Schröder, J., Vogt, F., Burgmaier, M., Reith, S., and Almalla, M. (2018). Long-term clinical outcomes after treatment of stent restenosis with two drug-coated balloons. Coronary Artery Dis. 29:1. doi: 10.1097/MCA.0000000000000664
Scott, R. C., Rosano, J. M., Ivanov, Z., Wang, B., Chong, P. L., Issekutz, A. C., et al. (2009). Targeting VEGF-encapsulated immunoliposomes to MI heart improves vascularity and cardiac function. FASEB J. 23, 3361–3367. doi: 10.1096/fj.08-127373
Setua, S., Menon, D., Asok, A., Nair, S., and Koyakutty, M. (2010). Folate receptor targeted, rare-earth oxide nanocrystals for bi-modal fluorescence and magnetic imaging of cancer cells. Biomaterials 31, 714–729. doi: 10.1016/j.biomaterials.2009.09.090
Sevick-Muraca, M. E. (2012). Translation of near-infrared fluorescence imaging technologies: emerging clinical applications. Annu. Rev. Med. 63, 217–231. doi: 10.1146/annurev-med-070910-083323
Sharma, M., Sharma, R., and Jain, D. K. (2016). Nanotechnology based approaches for enhancing oral bioavailability of poorly water soluble antihypertensive drugs. Scientifica (Cairo) 2016:8525679. doi: 10.1155/2016/8525679
Shi, C., He, Y., Ding, M., Wang, Y., and Zhong, J. (2019a). Nanoimaging of food proteins by atomic force microscopy. Part I: Components, imaging modes, observation ways, and research types. Trends Food Sci. Tech. 87, 3–13. doi: 10.1016/j.tifs.2018.11.028
Shi, C., He, Y., Ding, M., Wang, Y., and Zhong, J. (2019b). Nanoimaging of food proteins by atomic force microscopy. Part II: Application for food proteins from different sources. Trends Food Sci. Tech. 87, 14–25. doi: 10.1016/j.tifs.2018.11.027
Sioud, M. (2015). RNA interference: mechanisms, technical challenges, and therapeutic opportunities. Methods Mol. Biol. 1218, 1–15. doi: 10.1007/978-1-4939-1538-5_1
Somasuntharam, I., Boopathy, A. V., Khan, R. S., Martinez, M. D., Brown, M. E., Murthy, N., et al. (2013). Delivery of Nox2-NADPH oxidase siRNA with polyketal nanoparticles for improving cardiac function following myocardial infarction. Biomaterials 34, 7790–7798. doi: 10.1016/j.biomaterials.2013.06.051
Su, X., Chan, C., Shi, J., Tsang, M. K., Pan, Y., Cheng, C., et al. (2017). A graphene quantum dot@Fe3O4@SiO2 based nanoprobe for drug delivery sensing and dual-modal fluorescence and MRI imaging in cancer cells. Biosens. Bioelectron. 92, 489–495. doi: 10.1016/j.bios.2016.10.076
Suckau, L., Fechner, H., Chemaly, E., Krohn, S., Hadri, L., Kockskamper, J., et al. (2009). Long-term cardiac-targeted RNA interference for the treatment of heart failure restores cardiac function and reduces pathological hypertrophy. Circulation 119, 1241–1252. doi: 10.1161/CIRCULATIONAHA.108.783852
Suwa, T., Hogg, J. C., Quinlan, K. B., Ohgami, A., Vincent, R., and van Eeden, S. F. (2002). Particulate air pollution induces progression of atherosclerosis. J. Am. Coll. Cardiol. 39, 935–942. doi: 10.1016/s0735-1097(02)01715-1
Tadin-Strapps, M., Robinson, M., Le Voci, L., Andrews, L., Yendluri, S., Williams, S., et al. (2015). Development of lipoprotein(a) siRNAs for mechanism of action studies in non-human primate models of atherosclerosis. J. Cardiovasc. Transl. 8, 44–53. doi: 10.1007/s12265-014-9605-1
Tan, J., Thomas, A., and Liu, Y. (2011). Influence of red blood cells on nanoparticle targeted delivery in microcirculation. Soft Matter 8, 1934–1946. doi: 10.1039/C2SM06391C
Torchilin, V. (2011). Tumor delivery of macromolecular drugs based on the EPR effect. Adv. Drug Deliv. Rev. 63, 131–135. doi: 10.1016/j.addr.2010.03.011
Torchilin, V. P. (2002). PEG-based micelles as carriers of contrast agents for different imaging modalities. Adv. Drug Deliv. Rev. 54, 235–252. doi: 10.1016/S0169-409X(02)00019-4
Vimbela, G. V., Ngo, S. M., Fraze, C., Yang, L., and Stout, D. A. (2017). Antibacterial properties and toxicity from metallic nanomaterials. Int. J. Nanomedicine 12, 3941–3965. doi: 10.2147/IJN.S134526
Wall, E. E. V. D. (2013). Molecular imaging of coronary atherosclerosis; predictive of an acute myocardial infarction? Neth. Heart J. 22, 1–2. doi: 10.1007/s12471-013-0500-1
Wang, J., Jin, X., Huang, Y., Ran, X., Luo, D., Yang, D., et al. (2018). Endovascular stent-induced alterations in host artery mechanical environments and their roles in stent restenosis and late thrombosis. Regen. Biomater. 5, 177–187. doi: 10.1093/rb/rby006
Wang, W., Ding, J., Xiao, C., Tang, Z., Li, D., Chen, J., et al. (2011). Synthesis of amphiphilic alternating polyesters with oligo(ethylene glycol) side chains and potential use for sustained release drug delivery. Biomacromolecules 12, 2466–2474. doi: 10.1021/bm200668n
Wang, W., Sun, X., Zhang, H., Yang, C., Liu, Y., Yang, W., et al. (2016). Controlled release hydrogen sulfide delivery system based on mesoporous silica nanoparticles protects graft endothelium from ischemia-reperfusion injury. Int. J. Nanomedicine 11, 3255–3263. doi: 10.2147/IJN.S104604
Wang, Y., Chen, J., Yang, B., Qiao, H., Gao, L., Su, T., et al. (2016). In vivo MR and fluorescence dual-modality imaging of atherosclerosis characteristics in mice using profilin-1 targeted magnetic nanoparticles. Theranostics 6, 272–286. doi: 10.7150/thno.13350
Wang, Z., Cuddigan, J. L., Gupta, S. K., and Meenach, S. A. (2016). Nanocomposite microparticles (nCmP) for the delivery of tacrolimus in the treatment of pulmonary arterial hypertension. Int. J. Pharm. 512, 305–313. doi: 10.1016/j.ijpharm.2016.08.047
Wei, D., Qiao, R., Dao, J., Su, J., Jiang, C., Wang, X., et al. (2018). Soybean lecithin-mediated nanoporous PLGA microspheres with highly entrapped and controlled released BMP-2 as a stem cell platform. Small 14:1800063. doi: 10.1002/smll.201800063.
Wei, H., Bruns, O. T., Kaul, M. G., Hansen, E. C., Barch, M., Wisniowska, A., et al. (2017). Exceedingly small iron oxide nanoparticles as positive MRI contrast agents. Proc. Natl. Acad. Sci. U.S.A. 114, 2325–2330. doi: 10.1073/pnas.1620145114
Wei, L., Chen, J., Zhao, S., Ding, J., and Chen, X. (2017). Thermo-sensitive polypeptide hydrogel for locally sequential delivery of two-pronged antitumor drugs. Acta Biomater. 58, 44–53. doi: 10.1016/j.actbio.2017.05.053
Weissleder, R., and Ntziachristos, V. (2003). Shedding light onto live molecular targets. Nat. Med. 9, 123–128. doi: 10.1038/nm0103-123
Winter, P. M., Caruthers, S. D., Zhang, H., Williams, T. A., Wickline, S. A., and Lanza, G. M. (2008). Antiangiogenic synergism of integrin-targeted fumagillin nanoparticles and atorvastatin in atherosclerosis. JACC Cardiovasc. Imaging 1, 624–634. doi: 10.1016/j.jcmg.2008.06.003
Wu, T., Ding, M., Shi, C., Qiao, Y., Wang, P., Qiao, R., et al. (2019). Resorbable polymer electrospun nanofibers: history, shapes and application for tissue engineering. Chin. Chem. Lett. doi: 10.1016/j.cclet.2019.07.033. [Epub ahead of print].
Xi, X., Trost, D. W., and Sista, A. K. (2018). Successful management of drug-coated balloon (DCB) for recurrent iliofemoral venous in-stent restenosis (ISR). Clin. Imaging 49, 184–186. doi: 10.1016/j.clinimag.2018.03.018
Xia, H., Jun, J., Wen-ping, L., Yi-feng, P., and Xiao-ling, C. (2013). Chitosan nanoparticle carrying small interfering RNA to platelet-derived growth factor B mRNA inhibits proliferation of smooth muscle cells in rabbit injured arteries. Vascular 21, 301–306. doi: 10.1177/1708538113478737
Yang, C., Gao, S., Dagn S-Hansen, F., Jakobsen, M., and Kjems, J. R. (2017). Impact of PEG chain length on the physical properties and bioactivity of PEGylated chitosan/siRNA nanoparticles in vitro and in vivo. ACS Appl. Mater. Interfaces 9, 12203–12216. doi: 10.1021/acsami.6b16556
Yang, H., Zhao, F., Li, Y., Xu, M., Li, L., Wu, C., et al. (2013). VCAM-1-targeted core/shell nanoparticles for selective adhesion and delivery to endothelial cells with lipopolysaccharide-induced inflammation under shear flow and cellular magnetic resonance imaging in vitro. Int. J. Nanomedicine 8, 1897–1906. doi: 10.2147/IJN.S44997
Yang, X., Hong, H., Grailer, J. J., Rowland, I. J., Javadi, A., Hurley, S. A., et al. (2011). cRGD-functionalized, DOX-conjugated, and 64Cu-labeled superparamagnetic iron oxide nanoparticles for targeted anticancer drug delivery and PET/MR imaging. Biomaterials 32, 4151–4160. doi: 10.1016/j.biomaterials.2011.02.006
Yi, Z., Cai, L., Di, L., Lao, Y. H., Liu, D., Li, M., et al. (2018). Tumor microenvironment-responsive hyaluronate-calcium carbonate hybrid nanoparticle enables effective chemotherapy for primary and advanced osteosarcomas. Nano Res. 11, 1–17. doi: 10.1007/s12274-018-2066-0
Yingchoncharoen, P., Kalinowski, D. S., and Richardson, D. R. (2016). Lipid-based drug delivery systems in cancer therapy: what is available and what is yet to come. Pharmacol. Rev. 68, 701–787. doi: 10.1124/pr.115.012070
Yoo, S. P., Pineda, F., Barrett, J. C., Poon, C., Tirrell, M., and Chung, E. J. (2016). Gadolinium-functionalized peptide amphiphile micelles for multimodal imaging of atherosclerotic lesions. ACS Omega 1, 996–1003. doi: 10.1021/acsomega.6b00210
Yu, F., Wu, H., Tang, Y., Xu, Y., Qian, X., and Zhu, W. (2018). Temperature-sensitive copolymer-coated fluorescent mesoporous silica nanoparticles as a reactive oxygen species activated drug delivery system. Int. J. Pharm. 536, 11–20. doi: 10.1016/j.ijpharm.2017.11.025
Yu, J., Li, W., and Yu, D. (2018). Atrial natriuretic peptide modified oleate adenosine prodrug lipid nanocarriers for the treatment of myocardial infarction: in vitro and in vivo evaluation. Drug Des. Dev. Ther. 12, 1697–1706. doi: 10.2147/DDDT.S166749
Yue, X., and Dai, Z. (2018). Liposomal nanotechnology for cancer theranostics. Curr. Med. Chem. 25, 1397–1408. doi: 10.2174/0929867324666170306105350
Zhang, M., He, J., Jiang, C., Zhang, W., Yang, Y., Wang, Z., et al. (2017). Plaque-hyaluronidase-responsive high-density- lipoprotein-mimetic nanoparticles for multistage intimal-macrophage-targeted drug delivery and enhanced anti-atherosclerotic therapy. Int. J. Nanomedicine 12, 533–558. doi: 10.2147/IJN.S124252
Zhang, X., Beduhn, M., Zheng, X., Lian, D., Chen, D., Li, R., et al. (2012). Induction of alloimmune tolerance in heart transplantation through gene silencing of TLR adaptors. Am. J. Transplant. 12, 2675–2688. doi: 10.1111/j.1600-6143.2012.04196.x
Zhang, Z., Runa, A., Wu, J., Zhang, H., Li, X., and He, Z. (2019). Bioresponsive nanogated ensemble based on structure-switchable aptamer directed assembly and disassembly of gold nanoparticles from mesoporous silica supports. Chin. Chem. Lett. 30, 267–270. doi: 10.1016/j.cclet.2018.10.019.
Zhou, F., Jia, X., Yang, Q., Yang, Y., Zhao, Y., Fan, Y., et al. (2016). Targeted delivery of microRNA-126 to vascular endothelial cells via REDV peptide modified PEG-trimethyl chitosan. Biomater. Sci. 4, 849–856. doi: 10.1039/C5BM00629E
Zhou, X., Hao, Y., Yuan, L., Pradhan, S., Shrestha, K., Pradhan, O., et al. (2018). Nano-formulations for transdermal drug delivery: a review. Chin. Chem. Lett. 29, 1713–1724. doi: 10.1016/j.cclet.2018.10.037
Zhou, X., Luo, Y. C., Ji, W. J., Zhang, L., Dong, Y., Ge, L., et al. (2013). Modulation of mononuclear phagocyte inflammatory response by liposome-encapsulated voltage gated sodium channel inhibitor ameliorates myocardial ischemia/reperfusion injury in rats. PLoS ONE 8:e0074390. doi: 10.1371/journal.pone.0074390
Keywords: nano-drug delivery system, cardiovascular disease, targeting strategy, application progress, safety
Citation: Deng Y, Zhang X, Shen H, He Q, Wu Z, Liao W and Yuan M (2020) Application of the Nano-Drug Delivery System in Treatment of Cardiovascular Diseases. Front. Bioeng. Biotechnol. 7:489. doi: 10.3389/fbioe.2019.00489
Received: 21 November 2019; Accepted: 31 December 2019;
Published: 31 January 2020.
Edited by:
Wenguo Cui, School of Medicine, Shanghai Jiao Tong University, ChinaReviewed by:
Jian Zhong, Shanghai Ocean University, ChinaZhilu Yang, Southwest Jiaotong University, China
Copyright © 2020 Deng, Zhang, Shen, He, Wu, Liao and Yuan. This is an open-access article distributed under the terms of the Creative Commons Attribution License (CC BY). The use, distribution or reproduction in other forums is permitted, provided the original author(s) and the copyright owner(s) are credited and that the original publication in this journal is cited, in accordance with accepted academic practice. No use, distribution or reproduction is permitted which does not comply with these terms.
*Correspondence: Wenzhen Liao, wenzhenliao@163.com; Miaomiao Yuan, yuanmm2019@163.com
†These authors have contributed equally to this work