The Design of Poly(lactide-co-glycolide) Nanocarriers for Medical Applications
- Wits Advanced Drug Delivery Platform, Department of Pharmacy and Pharmacology, School of Therapeutic Sciences, Faculty of Health Sciences, University of the Witwatersrand, Johannesburg, South Africa
Polymeric biomaterials have found widespread applications in nanomedicine, and poly(lactide-co-glycolide), (PLGA) in particular has been successfully implemented in numerous drug delivery formulations due to its synthetic malleability and biocompatibility. However, the need for preconception in these formulations is increasing, and this can be achieved by selection and elimination of design variables in order for these systems to be tailored for their specific applications. The starting materials and preparation methods have been shown to influence various parameters of PLGA-based nanocarriers and their implementation in drug delivery systems, while the implementation of computational simulations as a component of formulation studies can provide valuable information on their characteristics. This review provides a critical summary of the synthesis and applications of PLGA-based systems in bio-medicine and outlines experimental and computational design considerations of these systems.
Introduction
The design of novel delivery systems using nanomaterials has experienced substantial growth since the application of nanotechnology to biomedical applications established the field of nanomedicine. As a result of the ongoing discovery of numerous new pharmaceutically active compounds which have shown excellent efficacy but inadequate clinical translation, there is a growing need to fill the gap between the formulations available and their successful inclusion into active treatment. This has urged scientists to investigate alternate forms of delivery to the biological target in order to overcome the hurdles associated with conventional drug delivery, such as poor drug entrapment, inadequate bioavailability and pharmacokinetics, as well as systemic toxicity and side effects. These novel delivery systems all strive for the “magic bullet” effect (Bosch and Rosich, 2008) which is a vehicle that can form favorable interactions with a lipophilic or hydrophilic drug to facilitate high drug loading (Aravind et al., 2013; Bauer et al., 2016), can shield the drug from physiological conditions, deliver it to the biological target with minimal loss, and then can release it at the site in a sustained manner and at therapeutic concentrations (Al-Jamal et al., 2016; Almoustafa et al., 2017). Moreover, the carrier is ideally biodegradable, biocompatible and non-immunogenic, with low systemic toxicity (Alshamsan, 2014; Ananta et al., 2016). Nanomaterials are a befitting source to meet these requirements because they can be tailored to a vast range of sizes and shapes and can suit various delivery mechanisms, while the interactions between the carrier and the physiological medium can be controlled by adapting the surface properties of the carrier (Kakkar et al., 2017). This has given rise to the widespread implementation of nanomaterials as pharmaceutical carriers for medical diagnostics and therapeutics (theranostics) (Berthet et al., 2017; Singh et al., 2019). Nanostructures can be fabricated from organic, inorganic, metallic or non-metallic sources. Examples include carbon nanotubes (Singh et al., 2013), dendrimers, liposomes, micelles, and solid lipid nanoparticles (Mishra et al., 2014; Lombardo et al., 2019).
Polymeric nanoparticles are commonly implemented as components of drug delivery systems and the use of synthetic polymers in particular can enable the design of carriers in a well-controlled and reproducible manner in order to suit the desired application (Lai et al., 2014). Polymer-based nanoparticles act as drug delivery vehicles by encapsulating the active agent inside its polymeric matrix, by conjugating to the agent or by adsorbing it onto the surface of the polymer (Mahapatro and Singh, 2011; Ansary et al., 2014). Polymers can be constructed to be linear, branched or globular and their size and their properties can be modulated by the choice of synthetic process (Panyam and Labhasetwar, 2012; Chen et al., 2013). Biodegradable polymers are suitable as nanocarriers as they often self-assemble and are easily sourced. Natural biodegradable polymers are chitosan (Ali and Ahmed, 2018), alginate (Jana et al., 2016) and inorganic ceramic hydroxyapatite composites (Turon et al., 2017). Synthetic polymers such as poly lactide-co-glycolide (PLGA) are an attractive alternative as they can be precisely engineered from monomers to suit the target and physiological environment they are intended for Middleton and Tipton (2000).
Poly lactide-co-glycolide has become ubiquitous in the bio-medical field for many reasons. Firstly, it is a synthetic, biodegradable polymer that is easily broken down in vivo by hydrolysis into lactic acid and glycolic acid. These monomers are biocompatible and are physiologically metabolized by the tricarboxylic acid cycle for final excretion in the lungs (Semete et al., 2010; Sequeira et al., 2018), as shown by Figure 1 (Sun et al., 2017). Hence, PLGA as a nanocarrier is considered to produce minimal systemic toxicity when used for biomedical applications (Kumari et al., 2010) and has been used in various formulations including membranes, sponges and gels (Sun et al., 2017).
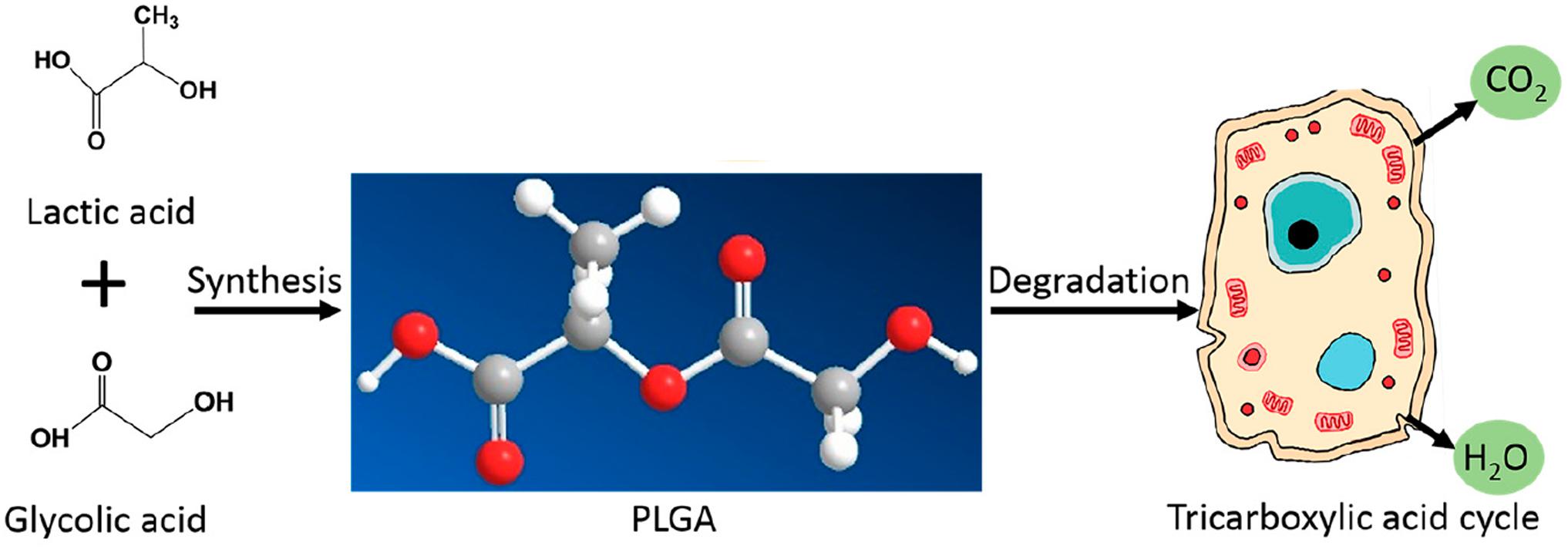
Figure 1. The synthesis and physiological breakdown of PLGA, adapted from Sun et al. (2017).
The appeal of PLGA also lies in the fact that its properties can be manipulated and adapted to modify the encapsulation profile and drug release kinetics of the nanostructure to suit the desired application (Mittal et al., 2007). PLGA is overall a hydrophobic polymer and is therefore detected by the RES and if unmodified, is bound by phagocytes for elimination by the liver or spleen and eliminated before delivering its payload to the target site (Danhier et al., 2012). To circumvent this, surface modification of PLGA is necessary. One such modification is the coating of hydrophilic poly ethylene glycol (PEG) groups on the surface of PLGA to shield the hydrophobic end groups from the reticulo endothelial system (RES), resulting in an amphiphilic di-block co-polymer (Salmaso and Caliceti, 2013). Other polymers used as surface modifiers include chitosan (Lu et al., 2019), polaxamer and poloxamines (Redhead et al., 2001) which work by altering the electrostatic and hydrophobic surface properties of the PLGA block co-polymer. To increase the therapeutic efficacy, the surface of the PLGA nanocarrier can be decorated with targeting ligands such as small molecules, antibodies, and aptamers. These molecules selectively bind to receptors on the target cell and guide the vehicle to the site of action (Jahan et al., 2017). Targeting moieties such as aptamers, have been shown to increase retention time at the site of action (Dinarvand et al., 2011).
The use of PLGA in biomedicine dates back to the 1970s when it was used as a component of biodegradable sutures and implants. With the advent of nanomedicine, it has found application as nanocarrier in various areas of medical research, including chemotherapeutics, immunology, and biomechanics (Swider et al., 2018). Numerous studies have also reported successful applications in antibiotics, antiseptics, imaging, wound healing, and as nano scaffolds (Sharma et al., 2016). The suitability and adaptability of PLGA as a nanocarrier is illustrated by Figure 2 (Mir et al., 2017).
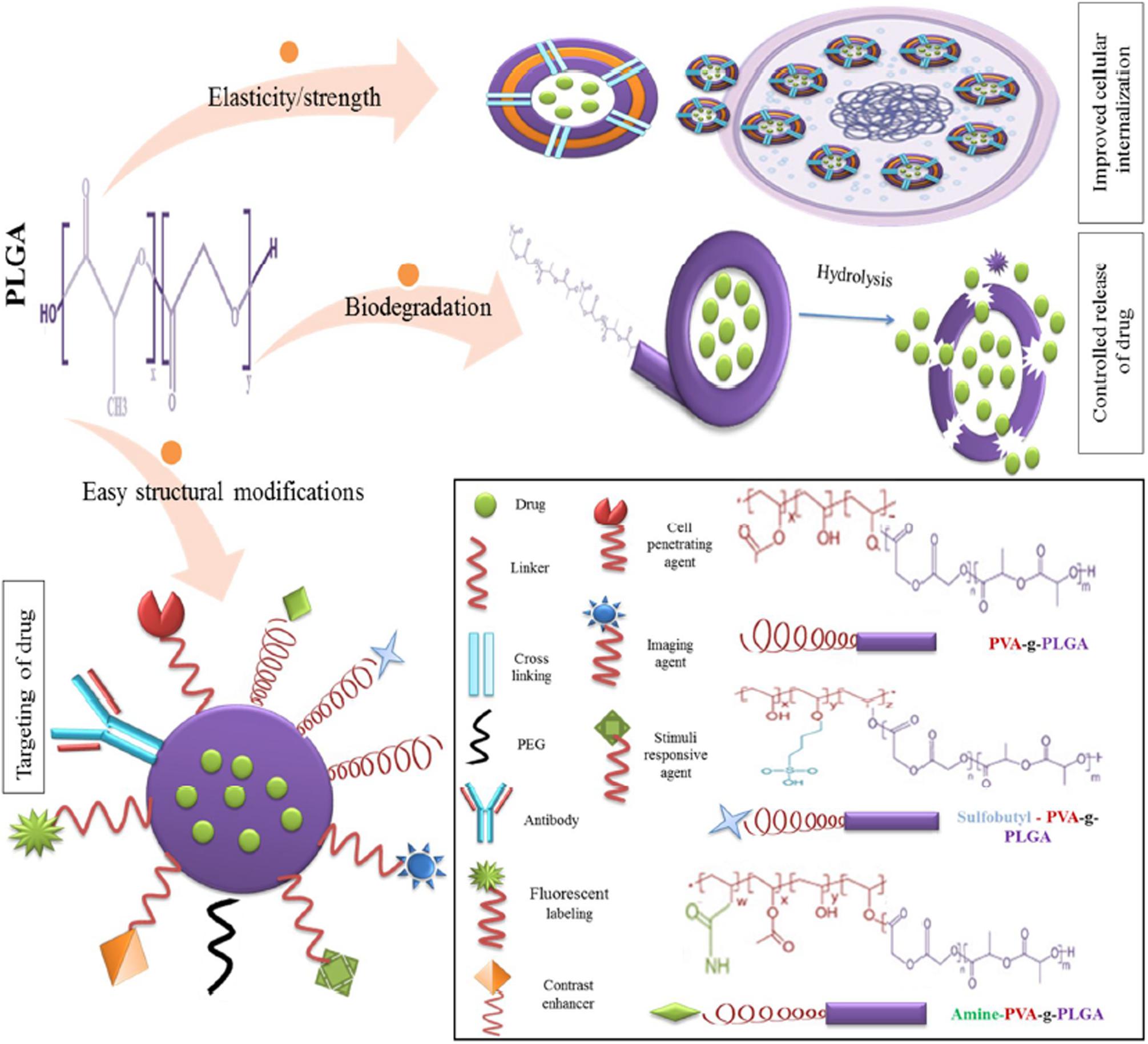
Figure 2. Favorable characteristics of PLGA as a nano delivery system, reproduced from Mir et al. (2017).
Optimizing the synthetic procedure by changing the parameters can affect other properties of nanocarriers and therefore a great deal of forethought should go into the design of the system for the particular application (Rezvantalab et al., 2018). During synthesis, parameters such as particle size, surface behavior, degree of crystallinity, degradation rate, and molecular weight can be modified to adapt the nanocarrier for desired dosage and site specific action (Mittal et al., 2007). Bio-nano interactions are important considerations in design as they determine the suitability of the nanostructure for the intended application as well as the undesired toxicity that may result from the engineering process. Previous research has indicated accumulation of PLGA in the liver when used as nanocarriers and therefore there could be toxicity challenges caused by dose dumping (Makadia and Siegel, 2011). While there are numerous reviews on PLGA based nanodelivery systems in general, this work considers the literature from a design perspective. Schrur’s nano-toxicology editorial states “few studies offer consistent results that are of value, and it is difficult to compare studies because they are often carried out using poorly characterized nanomaterials and arbitrary experimental conditions” (Schrurs and Lison, 2012). With these considerations in mind, in silico design, which is an expanding field in drug delivery, could be used to model numerous parameters, including polymer degradation, drug loading and toxicity and hereby provide insight into the structure-behavior relationships of PLGA-based nanocarriers (Ramezanpour et al., 2016). The aim of this review is to collate research on PLGA based delivery vehicles that have been studied for common medical applications, to compare the choices of starting materials and synthetic methods on the properties and functions of the final polymer-drug systems, and to explore how computational investigations can assist in the design of these systems.
PLGA as a Nanocarrier
Properties
Poly lactide-co-glycolide is synthesized from its constituent monomers (Sun et al., 2017) and can be obtained commercially in varying ratios of these monomers (Sadat Tabatabaei Mirakabad et al., 2014). Each constituent has its own physical characteristics that it brings to the co-polymer. PLGA retains properties of both copolymers and can be customized using these properties, which are stiff, hydrophobic and slowly degrading lactic acid vs. malleable, less hydrophobic and faster degrading glycolic acid (Engineer et al., 2011). For example, poly-DL -lactic acid has a methyl group, as shown in Figure 1, and is therefore more hydrophobic than poly glycolic acid. Hence, adjusting the concentration of poly-lactic acid in PLGA varies the solubility of the final polymer (Makadia and Siegel, 2011). A study investigating the rate of hydrolysis of PLGA demonstrated that increasing glycolic acid to lactic acid ratio increases the hydrophilicity of the PLGA co-polymer and hence leads to faster degradation (Keles et al., 2015), while a separate study quantified the degradation constant to be 1.3 times higher for glycolic units than for lactic units in the PLGA co-polymers investigated (Vey et al., 2011). It has been shown that PLGA co polymer ratios can be varied to adapt the degradation rate from months to years (Sun et al., 2017). In general, the higher the glycolic acid content of the PLGA polymer, the more amorphous it is and the faster it degrades due to it being more hydrophilic. An exception is PLGA 50:50 lactic: glycolic units, which has exhibited the fastest degradation rate (Lü et al., 2009). It has been shown that increasing the glycolic acid ratio increases the wettability of PLGA for thin film applications (Ayyoob and Kim, 2018) and that increasing the lactic acid content has application in designing PLGA carriers for sustained release (Li, 1999). PLGA co-polymers with lactic acid content less than 70% have been characterized as amorphous and suitable for drug delivery applications (Habraken et al., 2006). As expected, the higher the molecular weight of PLGA, the more structural integrity it exhibits and the longer it has shown to degrade in vivo (Anderson and Shive, 2012). The PLGA co-polymer can be end-capped with different functional groups which have shown to affect the degradation kinetics of the delivery system. For example ester end-capped polymers exhibit a slower degradation rate than acid end-capped polymers and are therefore suitable for slower release applications (Gentile et al., 2014). Apart from degradation rate, it is also possible to control solubility and glass transition temperature of the PLGA system by varying the molecular weight, lactic/glycolic ratios, and end-cap functional groups of the starting material (Gentile et al., 2014).
Poly lactide-co-glycolide is also soluble in a variety of organic solvents including acetone, dichloromethane, chloroform, ethyl acetate, and THF (Sharma et al., 2016) and therefore is relatively simple to work with as carriers for both hydrophobic and hydrophilic drugs (Zhang et al., 2014).
Surface Functionalization
Shielding
In order to avoid elimination by the RES, a stealth coating around the hydrophobic PLGA nanoparticle surface has been achieved by incorporation of co-polymers with desired properties. The most frequently used co-polymer is polyethylene glycol (PEG) as it is biocompatible and easily grafted or adsorbed onto the surface of PLGA. The hydrophilic PEG shields the PLGA carrier from being taken up by opsonins (Vllasaliu et al., 2014) and it has been shown that the PEG shield dramatically increases the blood circulation half-life of the nanocarrier (Owens and Peppas, 2006). Some studies have shown that the nanoparticle in vivo residence time is dependent on the surface density of the PEG chains (Bertrand et al., 2017). PEGylation has also been associated with enhanced drug loading and tunable carrier degradation (Khalil et al., 2013). Chitosan, a natural polymer that is formed by partial deacetylation of chitin, is also commonly grafted onto the surface of PLGA based systems to increase biocompatibility. It is biodegradable and has mucoadhesive properties as it carries a positive charge and can efficiently bind to negatively charged cell membranes (Bruinsmann et al., 2019). Hence, a coating of chitosan on the PLGA nanostructure shields it from opsonins and promotes stronger cellular interaction and retention (Lima et al., 2018). Collagen is a highly hydrophilic protein that also increases cellular interaction and when blended with PLGA, can form a delivery system with superior hybrid properties such as increased biological compatibility and mechanical strength (Sadeghi-Avalshahr et al., 2017). Heparin, a biocompatible material that can be obtained both naturally and synthetically, has been used to impart specific binding properties to delivery systems when combined with polymers (Rodriguez-Torres et al., 2018). It is a sulfated glycosaminoglycan with high binding affinity for various growth factors and has been used in sustained delivery applications by immobilization on the surface of PLGA delivery systems (Chung et al., 2006).
Surfactants
One of the strategies employed in the nanofabrication process to increase colloidal stability is the use of surfactants. These are agents which usually have amphiphilic properties and reduce the interfacial tension between the hydrophobic and hydrophilic components, hence increasing miscibility and dispersion (Heinz et al., 2017) and preventing particle aggregation (Shkodra-Pula et al., 2019). A commonly used agent is polyvinyl alcohol (PVA), which is a hydrophilic polymeric surfactant that has been shown to decrease the size and increase the uniformity of PLGA nanocarriers, but is also associated with hypertension and central nervous system depression in animal studies (Menon et al., 2012). The use of Polysorbate 80, 60, and 20 has also shown increased residence time and enhanced permeation of the blood brain barrier (Sharma et al., 2016). However, it has shown in some cases to cause anaphylactoid reactions (Coors et al., 2005) and long-term infertility (Gajdova et al., 1993). Polaxamer is a thermo-reversible, non-toxic coating that has been used when encapsulating hydrophobic drugs and has been shown to preferentially target cancer cells. However, it has shown rapid erosion times and it is associated with hyperlipidemia and hypercholesterolemia (Miller and Drabik, 1984). Poloxamine is an amphiphilic block co-polymer and therefore has been used to stabilize hydrophobic drugs while increasing circulatory residence time (Alvarez-Lorenzo et al., 2010). Vitamin E TGPS is a water-soluble form of vitamin E and is used as a solubilizing and emulsifying agent in nano drug delivery. It is commonly used to enhance drug loading (Zhang and Feng, 2006) and nanoparticle degradation rates (Jalali et al., 2011).
Active Targeting
In active targeting, the surface of the nanoparticle is further decorated with ligands that specifically bind to receptors on the cells of interest and enables the carrier to enter the cell by receptor mediated endocytosis (Muhamad et al., 2018). These targeted delivery systems are designed to localize drug release at the disease site (Danhier et al., 2010). There are various different kinds of targeting ligands such as small molecules, peptides, antibodies, aptamers and polysaccharides, as shown in Figure 3 (Yoo et al., 2019). These ligands can be either conjugated or adsorbed onto the surface of the nanocarrier after formation or can be linked to one of the components of the carrier before nanoparticle formation (Yoo et al., 2019). It has been shown that increasing the conjugation density of targeting ligands has an effect on the targeting ability of the nanocarrier.
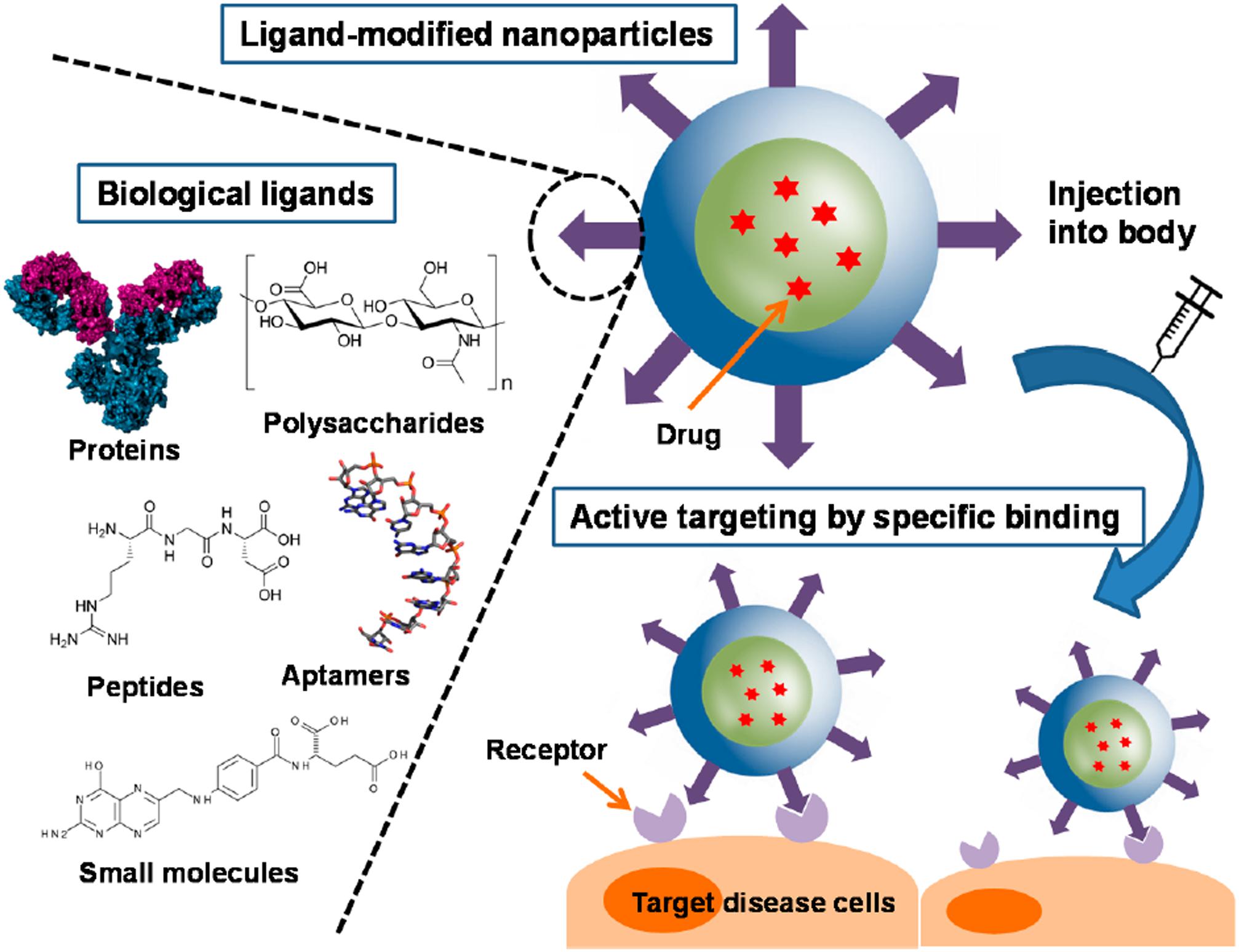
Figure 3. Surface functionalized PLGA nanoparticles for active targeting and cellular binding reproduced from Yoo et al. (2019).
Monoclonal antibodies have had a long history as targeting ligands (Friedman et al., 2013) since they have complementarity determining regions that enable them to bind to receptors on cell surfaces with high specificity and affinity (Carter et al., 2016). However, since they are large molecules, their conjugation density capacity on the nanocarrier is substantially decreased (Yoo et al., 2019) compared to other ligands, and furthermore, they raise immunogenicity concerns (Karra and Benita, 2012). Compared to antibodies, peptides have the advantage of smaller size and non-immunogenicity but they still are able to retain target specificity (Zhao et al., 2007). Aptamers are short strands of nucleic acids that can be synthetically designed to bind specific biological targets. They are non-immunogenic and non-toxic but their synthesis can be costly (Friedman et al., 2013). Despite their advantages for in vivo targeting, both peptides and aptamers are prone to enzymatic degradation (Yoo et al., 2019). Polysaccharides are advantageous because they are biocompatible and can be used as structural components of the nanocarrier (Choi et al., 2011) as well as to target carbohydrate binding receptors on cell surfaces. However, some polysaccharides could have solubility challenges and modification of the carbohydrate structure could result in unintended toxicity (Peng et al., 2018). Small molecules form a class of targeting ligands that comprise of synthetic compounds that are designed to target certain domains on cell surface receptors. They are usually chosen because of ease and control of synthesis but they often do not bind with high specificity and some target receptors can be expressed in healthy cells (Yoo et al., 2019), resulting in unintentional cell binding. Even though active targeting strategies provide an attractive avenue for site specific drug delivery, there are many challenges in this area, such as receptor accessibility and off-target binding. Particularly during different stages of tumor development, certain receptors can be up or down regulated, which provides an additional challenge for the use of targeting ligands in chemotherapeutic drug delivery (Vhora et al., 2014).
Toxicity
Despite biocompatibility and biodegradability of PLGA as a polymer, its toxicological profile in nanoformulations deserves to be investigated because of altered physicochemical properties, such as higher surface area to mass ratios. Furthermore, reports have suggested that particles of any material may acquire unique toxicological properties in the nanoscale (Makadia and Siegel, 2011). Different effects such as acute toxicity, repeated dose toxicity, inflammation, oxidative stress, genotoxicity, and reproductive system toxicity of PLGA nanocarriers have been examined in order to obtain information on the possible risks of these materials in pharmaceutical preparations. A study of danorubicin loaded PEG-PLL-PLGA nanoparticles has described some toxicity in Kunming mice (Guo et al., 2015) but since no results were reported for blank nanoparticles, it is unclear whether the toxicity was due to the drug or nanocarrier (Jesus et al., 2019). Regarding oxidative stress, studies have reported an overall increase in the production of reactive oxidative species corresponding to increasing concentrations of the PLGA nanoformulations tested (Singh and Ramarao, 2013; Grabowski et al., 2015) and another study demonstrated mild inflammatory properties of different PLGA formulations (Grabowski et al., 2016). Several studies have confirmed no genotoxicity (Tulinska et al., 2015; Platel et al., 2016), no toxicity on reproduction (Chen et al., 2017; Sharma et al., 2017) and no hemolysis (Chen et al., 2017). A study comparing the toxicity of PLGA nanoparticles to silica-, iron-, and zinc-based nanoparticles showed that the PLGA system had no appreciable adverse in vitro or in vivo toxicological outcomes, and did not produce the toxicity commonly associated with the inorganic nanomaterials (Semete et al., 2010).
Synthetic Methods of PLGA Nanocarriers
Poly lactide-co-glycolide nanocarriers may be fabricated by different methods and the choice of method has shown to affect properties such as particle size, colloidal stability, drug loading/encapsulation efficiency, and release behavior of the final product (Swider et al., 2018). Depending on the process of preparation, the structural organization may also be different. The drug is either encapsulated inside the carrier or adsorbed on the surface (Danhier et al., 2012). There are several methods that can be employed for the preparation of PLGA nanocarriers, and the following provides a brief overview on both the well-established and relatively recently developed techniques. The traditional methods based on emulsions are illustrated by Figure 4 (Ding and Zhu, 2018).
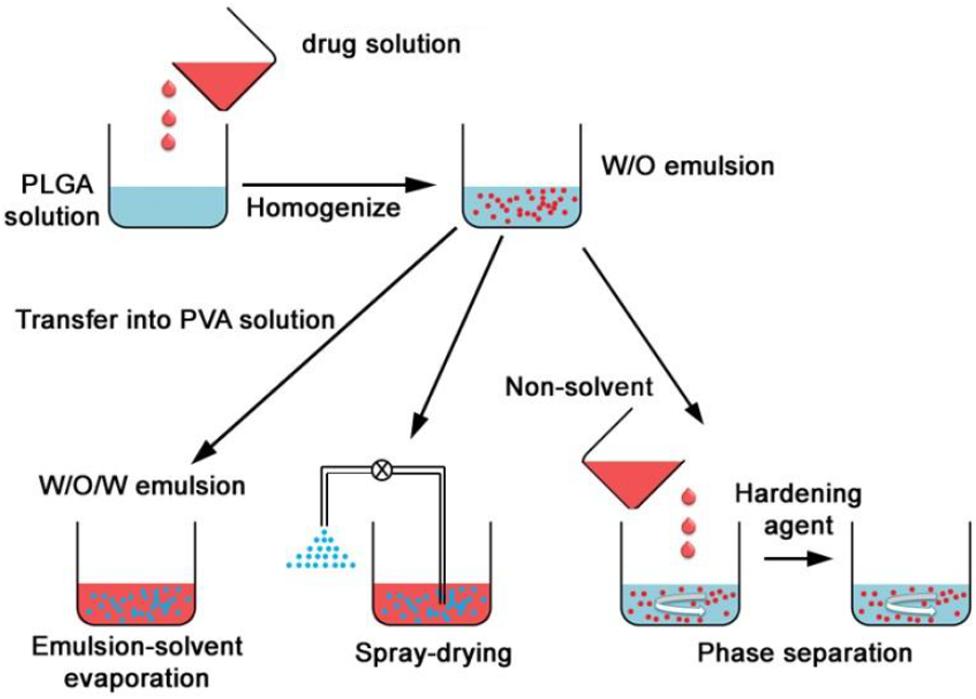
Figure 4. Formation of PLGA nanoparticles by double (W/O/W) emulsion, spray drying and phase separation (coacervation) methods, adapted from Ding and Zhu (2018).
Single and Double Emulsion
The emulsion methods have been the most frequently used methods of synthesis and they are suitable for a wide range of drugs with varying solubilities (Wang et al., 2016). The single emulsion (oil in water or O/W) method is suitable for hydrophobic drugs. PLGA and the drug is dissolved in a small volume of suitable volatile organic solvent and added dropwise to the aqueous phase containing a stabilizer, usually PVA. The mixture is sometimes sonicated and then stirred, often under sheer stress for a fixed amount of time to allow the organic solvent to evaporate. The double emulsion method is used when the active agent to be entrapped is hydrophilic, such as proteins and peptides. The active is dissolved in an aqueous phase and then added to PLGA which is dissolved in the organic phase, and this forms a primary water in oil (W/O) emulsion. This is then added to another aqueous phase containing a stabilizer and allowed to mix under stress, allowing the organic solvent to evaporate. The nanoparticles are therefore formed by a water in oil in water (W/O/W) emulsion (Makadia and Siegel, 2011). The product is isolated by centrifugation or ultrafiltration and washed to remove unreacted products. Thereafter it is freeze dried and can be stable for several months to years. Recently, a single emulsion method was used to successfully entrap proteins for vaccine application (Ospina-Villa et al., 2019) and a PLGA-PEG nanocarrier was formulated using the double emulsion method for intraperitoneal insulin delivery (Haggag et al., 2018). The emulsion methods can be adjusted by changing the drug to PLGA ratio, the organic solvent, the stabilizer concentration in the aqueous phase and the stirring speed and can hereby be adapted to control the size range of the nanocarriers to some extent. However, there are often batch to batch variation with these methods and the carriers prepared by this method for protein-based drugs have limited stability due to degradation of proteins at the aqueous interface and the sheer stress of homogenization leading to unfolding of the protein sheets (Ding and Zhu, 2018).
Spray Drying
This method involves the preparation of water in oil or solid in oil emulsions, which are sprayed in a thin stream of heated air. The type of drug (hydrophilic or hydrophobic) would determine the solvent used in the emulsion (Makadia and Siegel, 2011). Recently, spray drying was used in the preparation of a PLGA nanoformulation for sustained treatment of tuberculosis (TB) (Kalombo et al., 2019) and in the fabrication of a carrier for antibiotic coating of dental implants (Baghdan et al., 2019). This method is highly advantageous because it is suitable for hydrophobic and hydrophilic drugs and can be used for sensitive compounds since the conditions are mild. It is also a rapid method (Nie et al., 2008) which can be suitable for industrial scale-up due to the minimal processing parameters involved (Ding and Zhu, 2018). The main drawback of this technique is the wastage caused by inaccessible product that adheres to the inside of the nanosprayer (Wang et al., 2016). Parameters such as orientation of jets, temperature, and solvent choice can all affect the properties of the final nanoparticles (Berkland et al., 2004).
Coacervation
With coacervation or phase separation, the polymer and drug are prepared as O/W for hydrophobic drugs and W/O/W for hydrophilic drugs, and then a non-solvent, e.g., silicon oil is added dropwise under stirring (Verma et al., 2018). This reduces the solubility of PLGA in the organic solvent and results in the formation of a polymer-rich phase in which PLGA surrounds the drug molecules to form microdroplets (coacervates). These are rapidly quenched in a non-soluble medium to form the solid product (Wang et al., 2016). Parameters such as starting polymer, solvent choice, stirring rate and temperature can be varied to control the properties of the particles. Coacervation usually forms micrometer sized particles (Sharma et al., 2016) but has been used in protein nanoparticle preparation (Verma et al., 2018).
Salting Out
In the salting out method, drug and PLGA are dissolved in a miscible organic solvent and added to the aqueous phase containing stabilizer and a salt under sheer mechanical force. The salt usually used is magnesium chloride hexahydrate or magnesium acetate tetrahydrate (Wang et al., 2016) and is used at a ratio of 1:3 PLGA:salt (Eley et al., 2004). Upon addition of water, the organic solvent diffuses into the aqueous phase, causing the formation of PLGA-drug nanoparticles, illustrated by Figure 5 (Crucho and Barros, 2017). This method is not suitable for lipophilic drugs and can be time intensive since isolation of the product involves several washing steps to remove reagents. However, it would suit drugs which are very temperature sensitive since heat is not required (Nagavarma et al., 2012). This method is robust and is suitable for nanoparticles with high polymer concentrations since the size of the particle is not generally affected by the amount of polymer (Swider et al., 2018).
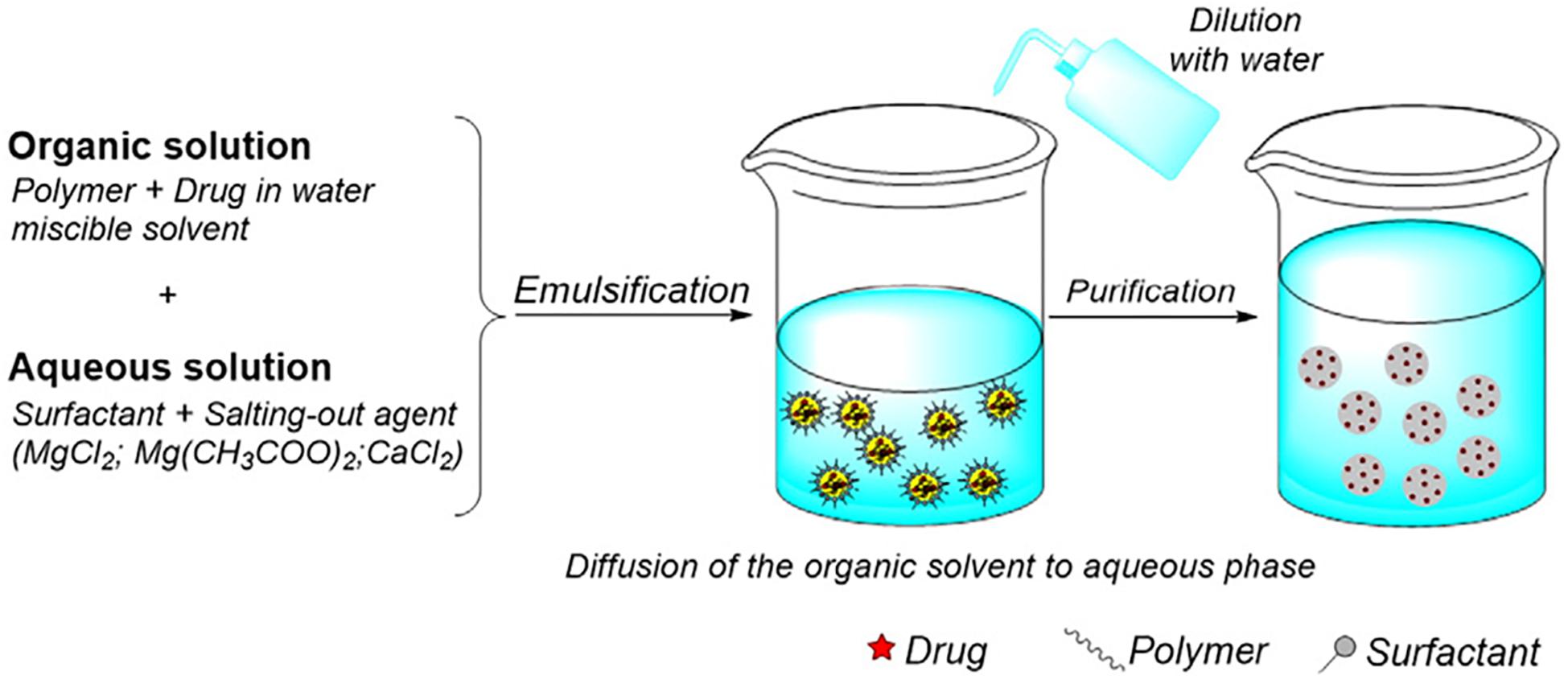
Figure 5. Illustration of the salting out method of preparation, reproduced from Crucho and Barros (2017).
Nanoprecipitation
In this method, PLGA and the drug is dissolved in a polar, water miscible solvent and added dropwise to the aqueous phase, which may contain a surfactant. The product is formed by rapid diffusion of the water miscible solvent into the aqueous phase, resulting in precipitation of the PLGA-drug nanoparticles, as shown in Figure 6 (Crucho and Barros, 2017). The properties of the nanocarrier are controlled by PLGA content and molecular weight, PLGA to drug ratio and choice of solvent (Wang et al., 2016). Recently, an optimized nanoprecipitation method was developed for the preparation of PLGA encapsulated alendronate sodium, a drug for osteoporosis (Oz et al., 2019) and a modified procedure was reported for a PLGA hybrid nanocarrier for simvastatin (Zhang et al., 2018). Nanoprecipitation can be used to prepare particles in the 100 nm size range and is advantageous because of the absence of shear stress (Fessi et al., 1989). However, the unmodified nanoprecipitation method does not usually work well for hydrophilic drugs as they do not form favorable interactions with PLGA in a water miscible solvent (Govender et al., 1999).
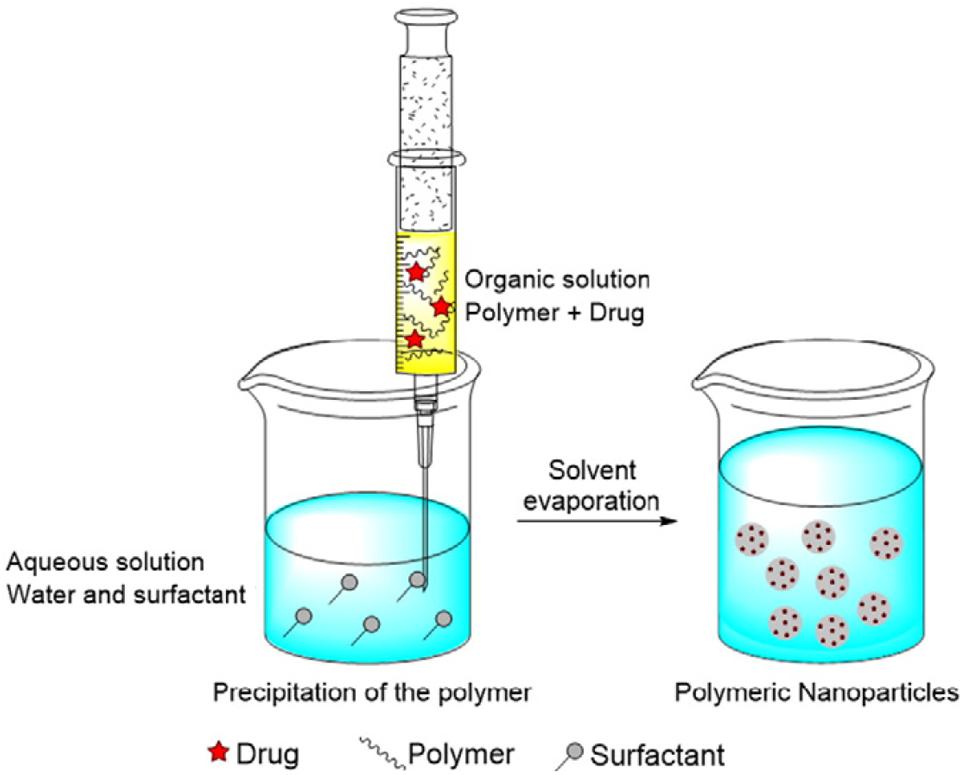
Figure 6. The nanoprecipitation method, adapted from Crucho and Barros (2017).
Supercritical Fluid Technology
Supercritical fluid technology, illustrated by Figure 7 (Jog and Burgess, 2017), can provide an environmentally friendly method of generating nanoparticles since it reduces, and in some cases, eliminates the use of organic solvents (Koushik and Kompella, 2004). In this method, the polymer and drug are dissolved in a supercritical fluid which is then rapidly expanded and depressurized. The resultant mixture is then passed through a fine nozzle or capillary, resulting in supersaturation and formation of nanoparticles, which are collected separately (Mishima, 2008). This is an attractive method as it is highly tunable but the kind of nanoparticle products are restricted since not all starting materials are compatible with the supercritical fluid (Soh and Lee, 2019).
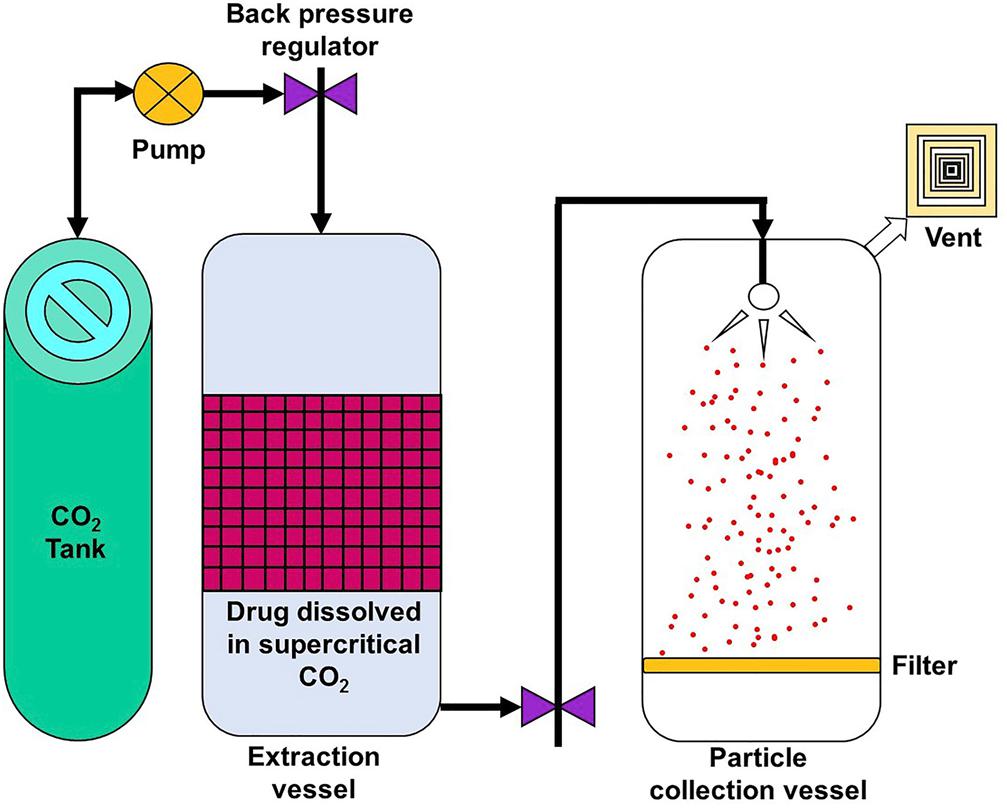
Figure 7. Simple setup of a supercritical fluid technology method, reproduced from Jog and Burgess (2017).
Microfluidics
The area of microfluidics deals with channels of the micrometer size range that are used to control and manipulate the movement of volumes of fluid from the nanolitre size range and below. When working at the nanoscale, the conditions of flow can be precisely controlled and constant laminar flow is maintained, which is impossible when conducting reactions at the macroscale level (Chiesa et al., 2018). Therefore this technique has lent itself to the synthesis of nanoparticles by the formation of emulsions using droplet microfluidics. In this method, the polymer and drug are combined and the emulsion is formed in the microfluidic mixer, which can have different channel architechtures (Collins et al., 2015). The most commonly used geometries for droplet based PLGA nanoparticle formation are the t-junction, flow focusing, and continuous flow microchannels, as shown in Figure 8I (Shembekar et al., 2016). In the t-junction geometry, channels are perpendicular to each other. The dispersed phase (aqueous) flows through one channel while the continuous phase (oil) flows through the other and droplets are formed at the junction. In the flow focusing system, the aqueous phase flows through a square capillary where shear force is provided on either side of it by the flow of the oil phase. The emulsion then flows through a narrow capillary and droplets are formed in a collection chamber. With continuous flow geometry channels, the aqueous phase flows through a capillary that resides in another capillary through which the oil flows in the same direction. Droplets begin to form once the two phases mix (Shembekar et al., 2016). SEM images of particles formed by this method are shown in Figures 8II-D,E (Xu et al., 2009).
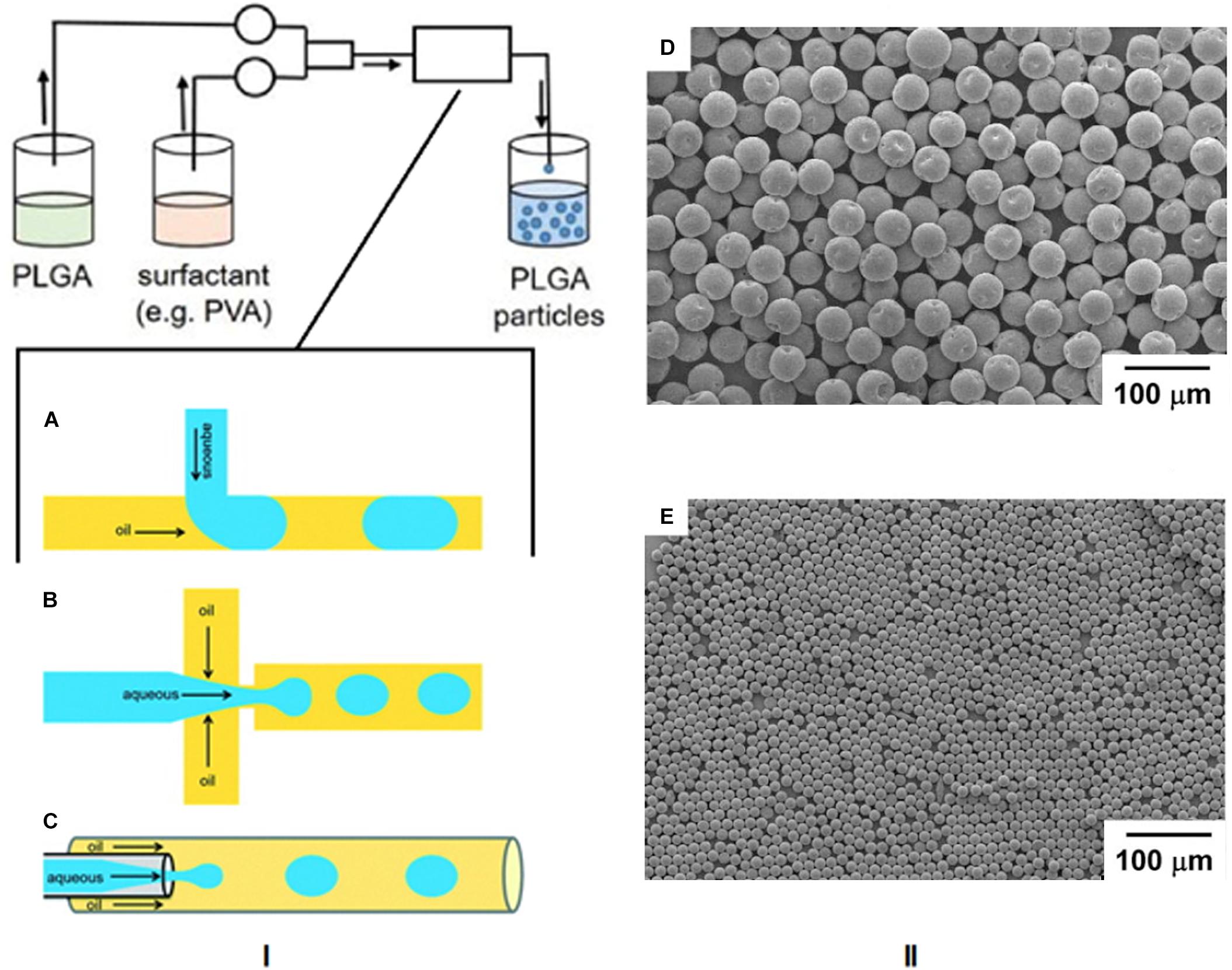
Figure 8. (I) Schematic of the microfluidics method and the three most common microchannel geometries. (A) T junction, (B) flow focusing, (C) continuous flow. Reproduced from Shembekar et al. (2016)-published by the Royal Society of Chemistry. (II-D,E) SEM images of PLGA particles formed by this technique, adapted from Xu et al. (2009).
These microchannels are used for O/W emulsions, but can be adapted for double (W/O/W) emulsions by using a combination of channels. Recently, a microfluidics method was developed for the encapsulation of cell penetrating peptides (Streck et al., 2019) and targeted delivery of taxanes (Martins and Sarmento, 2020). There are numerous advantages of microfluidics for nanoparticle synthesis. With this technique, the chemical composition of the final product can be preselected according to the desired application. The synthetic parameters can be controlled to the extent that there is a much larger particle size homogeneity compared to bulk methods, and there are also smaller volumes of solvent needed. However, the scale of nanoparticle production is limited and the microchannels are also susceptible to blockage and contamination. The time and temperature of mixing, flow rate, choice of solvents and payload type determine the properties of the final nanoparticles (Kim K.T. et al., 2019).
Membrane Extrusion Emulsification
With this technique, single or double emulsions of PLGA and drug are either initially prepared or formed when extruded through a membrane of predetermined pore size. There are two ways to do this – direct and pre-mix membrane extrusion (ME), as illustrated by Figure 9A (Guo et al., 2018), and a characteristic emulsion is shown in Figure 9B. In direct ME, the membrane emulsifies the dispersed phase into nanosized droplets, while in premix ME, the emulsion is formed via a conventional method and thereafter extruded through the membrane, which downsizes the coarse emulsion into uniform nanosized droplets. This method can be used for hydrophobic or hydrophilic drugs and is advantageous because the size of product can be controlled by varying the nanoporous membrane pore size to create particles of required dimensions, resulting in a large size homogeneity. Premix ME in particular has been shown to have a higher uniformity in dispersity of final nanoparticles as shown by Figure 9C, compared to direct ME. In general, it is a mild procedure with low energy requirements and can be easily scaled up. However, it is not suitable for emulsions with high viscosity (Guo et al., 2018).
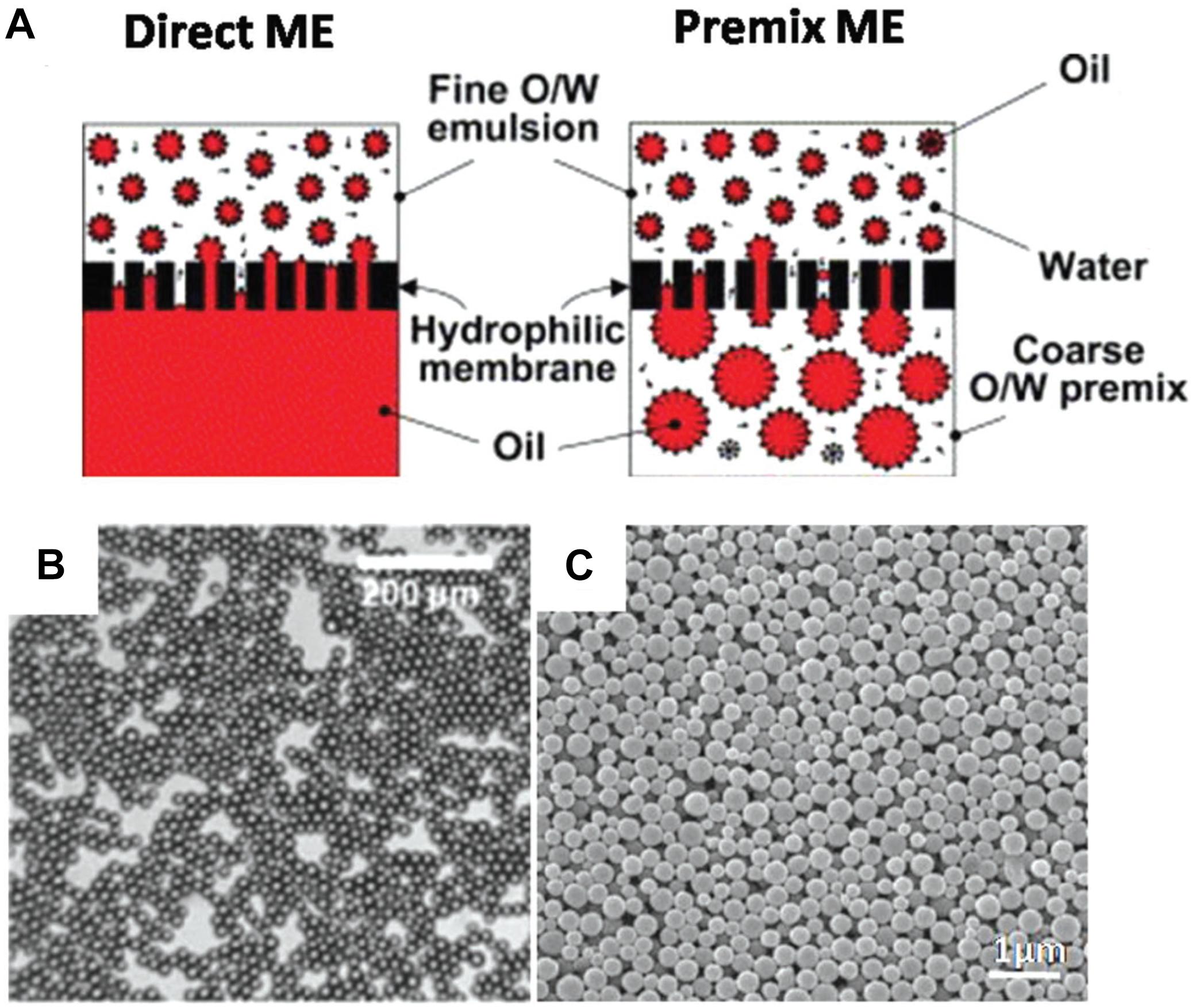
Figure 9. (A) Direct- and premix- two common processes using membrane extrusion (ME). (B) SEM image of an emulsion formed by this method (C) SEM images of nanoparticles formed by premix ME, reproduced from Guo et al. (2018).
Nanoimprint Lithography and the PRINT Technique
Nanoimprint lithography is used to form nanoparticles from a nanostructure template that is placed over a layer of precursor material which is heated to above the glass transition temperature of the polymer. Thereby, the malleable precursor material is molded into the desired size and shape, which is retained upon cooling. The template is then removed, leaving the product on the substrate base. Su et al. (2015) have successfully used this method for the nanofabrication of submicron PLGA grooves for the control of the length and direction of retraction fibers during cell division. The major drawback of this method is the residual interconnecting layer on the substrate base that prevents the formation of isolated nanostructures (Fu et al., 2018). The PRINT (particle replication in non-wetting template) technique involves the preparation of the PLGA-drug solution matrix and casting it on a delivering sheet. Thereafter a mold with nanosized cavities is placed over the delivering tray and it is passed through a nip and separated so that the polymeric material fills the mold cavities. The particles are then solidified and placed on a high energy adhesive layer and passed through the nip without separation. After the mold is removed, the nanoparticles are collected by washing with a solvent that dissolves the adhesive (Perry et al., 2011). The method is automated with a high degree of control over the individual parameters, and can be used for a wide variety of cargos including hydrophobic and hydrophilic drugs, vaccines, and proteins. However, it is a multistep process that can be labor intensive (Swider et al., 2018). The desired particle size, surface properties and composition can be preset and controlled in the initial step. The PRINT process is illustrated in Figure 10 (Perry et al., 2011), while Figure 11 shows SEM micrographs of the different shapes of PLGA nanoparticles that have been prepared by this method. Enlow and colleagues have reported the PRINT process whereby PLGA micro- and nanoparticles were prepared, with cylindrical, spherical, ridged, and fenestrated morphologies. These particles demonstrated > 40% drug loading and > 90% encapsulation efficiencies of docetaxel (Enlow et al., 2011).
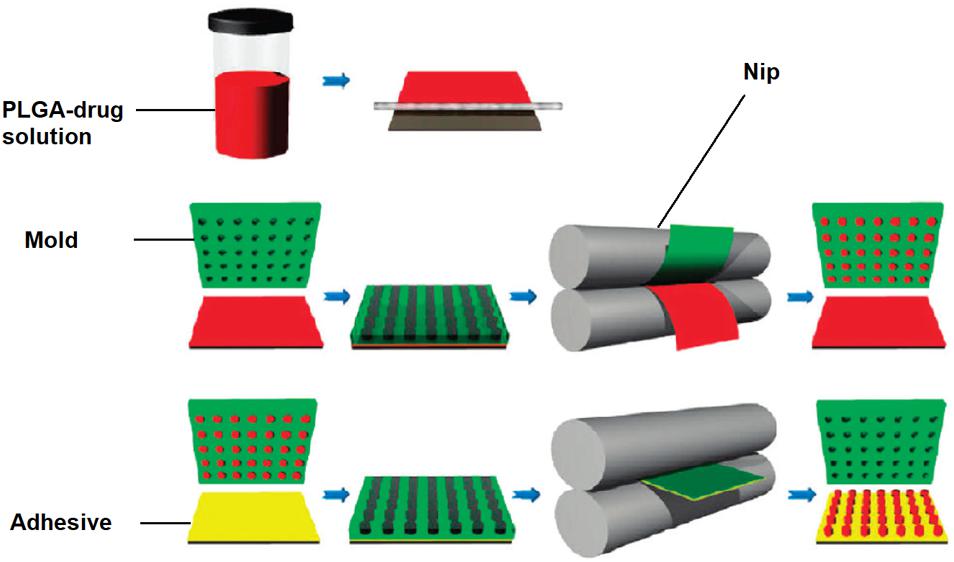
Figure 10. Schematic showing PRINT procedure, reprinted with permission from Perry et al. (2011). Copyright (2019). American Chemical Society.
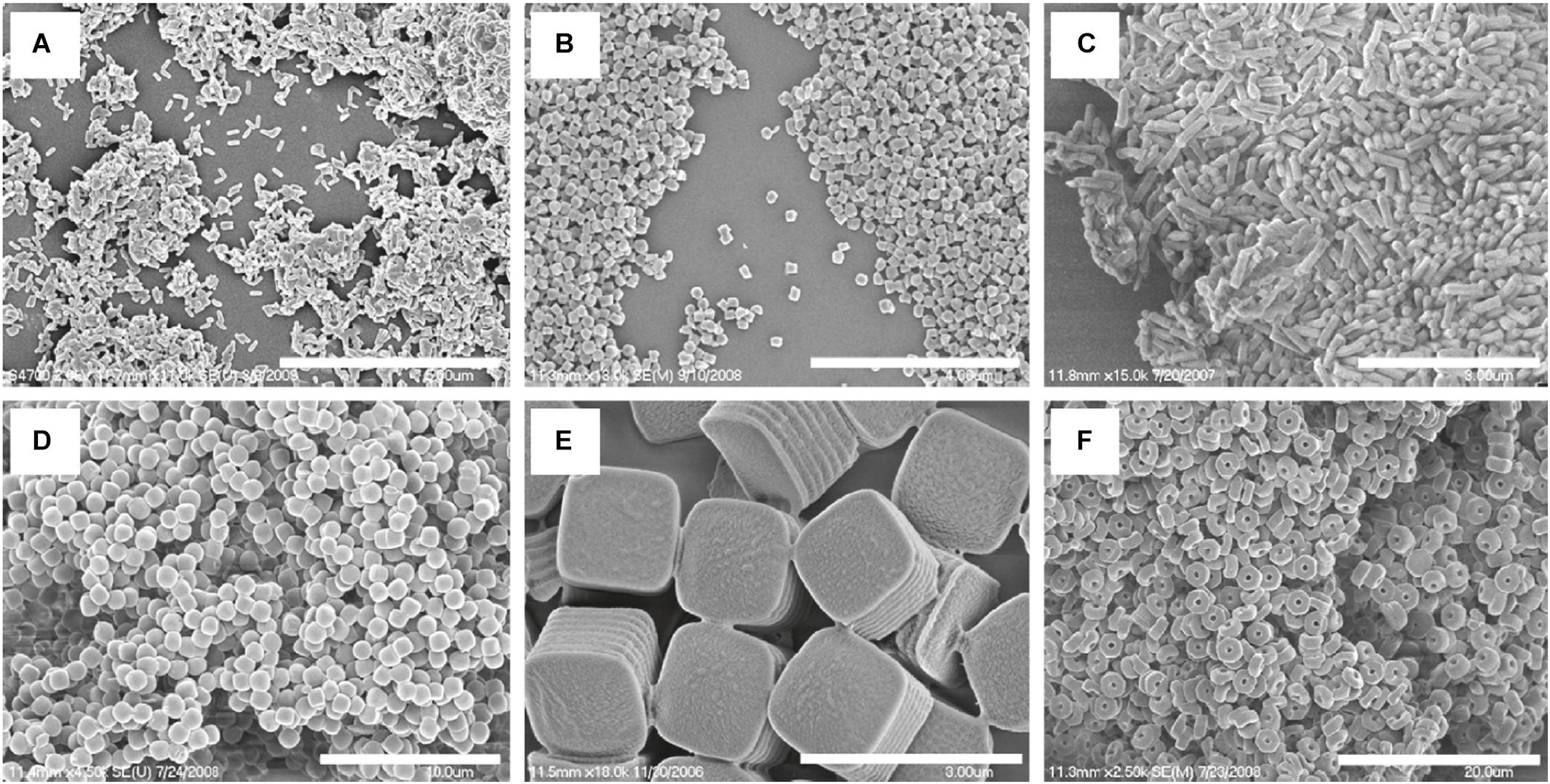
Figure 11. SEM Images of PLGA PRINT particles. (A–C) Cylinders of different dimensions. (D) Spheres; (E) ridged cubes; (F, bottom right) particles with center fenestrations, reprinted with permission from Enlow et al. (2011). Copyright (2019) American Chemical Society.
Medical Applications
Cancer Research
Actively Targeted Chemotherapeutics
In the United States of America alone, 1,762,450 new cancer diagnoses and 606,880 cancer related deaths are expected to occur in 2019 Siegel et al. (2019). Despite the ubiquity of this disease, treatment options are challenging due to the complex pathology of the different cancers. Current chemotherapy often leaves debilitating and life altering side effects since most drugs on the market that target the rapidly dividing cancer cells also inadvertently damage cells that are vital for normal life processes (Rizvi and Saleh, 2018). Actively targeting PLGA nanoparticles are able to circumvent this; Moku et al. (2019) have shown increased drug loading and efficacy against lung cancer by using transactivator of transcription (TAT) peptide ligands to target mesenchymal stem cells while Ganipineni et al. (2019) found that magnetically targeted paclitaxel- and SPIO-loaded PLGA-based nanoparticles effected increased cellular uptake in glioblastoma cells compared to the non-targeted carriers. Another type of nanoparticle targeting is the use of ‘smart’ carriers that are engineered to respond to a stimulus (Kapoor et al., 2015). Recently, a pH dependent aptamer functionalized PLGA nanocarrier system was reported to increase anti-cancer activity of doxorubicin to human lung cancer cells, with reduced toxicity to healthy cells (Saravanakumar et al., 2019) and a superparamagnetic iron oxide encapsulated nanocarrier for docetaxel demonstrated favorable pharmacokinetics and a greater degree of uptake in breast cancer cells (Panda et al., 2019).
Immunotherapy
Since Allison and Honjo were awarded the 2018 Nobel prize in Physiology and Medicine “for their discovery of cancer therapy by inhibition of negative immune regulation” (Guo, 2018), the area of nanomedical research into cancer immunotherapy has received substantial attention. This approach involves the use of pharmaceutical agents to activate a patient’s immune system to fight cancers as opposed to traditional chemotherapy which involves directly drugging the cancer cells (Khalil et al., 2016). Chen et al. (2016) have described PLGA nanocarriers equipped with an immunostimulant and photothermal agent, and this formulation showed increased activation of the immune system of BALB mice compared to the free agent. More recently a sustained controlled release PLGA nanosystem was developed to activate the anti-tumor immune response in mice bearing melanoma and colon cancer (Yin, 2019) and a PLGA system containing an immune adjuvant together with an enzyme that increased the efficacy of radiation therapy demonstrated the feasibility of combination immunotherapy and targeted radiotherapy in BALB mice (Chen et al., 2019).
Imaging and Diagnostics
Poly lactide-co-glycolide has applications for tumor diagnostics as it is able to deliver imaging agents to cancer cells with specificity and controlled biodistribution. Advances in nanotheranostics, which is the incorporation of imaging and therapeutic agents in one nanocarrier, have shown promise for real time imaging throughout a patient’s treatment course (Chapman et al., 2013). A novel theranostic PLGA nanocarrier with a near infrared imaging agent, further decorated with gold nanoparticles has been synthesized and shown to have increased activity and photodynamic properties in tumor grafted BALB mice (Xi et al., 2018) and a targeted PLGA-based nanobubble was designed with an ultrasound contrast agent, and demonstrated specificity and imaging capabilities to breast cancer in BALB mice (Du et al., 2018). More recently an image guided photothermal PLGA nanocarrier for doxorubicin showed promise for real time photoacoustic imaging in tumor bearing nude mice (Shen et al., 2019) and a near infra-red dye loaded PEGylated PLGA nanocarrier was also able to provide information on the circulation and distribution of the nanoparticles in nude mice (Kumar et al., 2019).
HIV Treatment
The delivery of anti-retro viral drugs faces many of the general limitations of conventional drug delivery and therefore biomaterials with low toxicity such as PLGA based nanocarriers are being implemented in formulations to treat HIV. Mannosylated PLGA nanoparticle carriers have shown promise for targeted delivery of anti-retro viral drugs to the brain (Patel et al., 2018) and the use of microfluidics technology enabled the novel synthesis of efiravine loaded PLGA nanoparticles (Martins et al., 2019). A recent study reported a PLGA based nanocarrier for the combination of the anti-retro virals griffithsin and dapirivine which showed a long acting treatment profile (Yang et al., 2019) and a separate proof of concept study showed promise for a long acting bictegravir encapsulated PLGA nanocarrier (Mandal et al., 2019).
Inflammatory Disorders
Many current treatments have proven to be inadequate at treating or alleviating symptoms of inflammation. The specific delivery of anti-inflammatory agents to the target site could potentially increase their therapeutic concentration in the inflamed tissue with reduced side effects (Gendelman et al., 2015), and the use of PLGA is particularly suitable to this application because of its favorable biodegradability and non-immunogenicity (Lamprecht et al., 2001). Davoudi et al. (2018) described a carrier within a carrier system using intestinal organoids to transport 5-ASA encapsulated PLGA nanoparticle to treat inflammatory bowel disease, and Perreira’s research involved the development of a metformin loaded nanoformulation that showed efficacy against periodontal inflammation in diabetic rats (Pereira et al., 2018). Gholizadeh et al. (2018) have formulated a dactolisib-PLGA nanoparticle that showed activity against inflamed endothelial cells and more recently, Yang (2019) group reported the synthesis of a crocetin-loaded nanoparticles that reduced the level of pro-inflammatory cytokines in renal tissue and therefore shows potential for the treatment of diabetes induced nephropathy.
Other Applications
Poly lactide-co-glycolide has been adapted to treat conditions in many fields of biomedicine, as shown by Figure 12 (Mir et al., 2017). A hyaluronic acid functionalized PLGA based nanocarrier for methatrexate has been developed for targeted treatment of rheumatoid arthritis (Trujillo-Nolasco et al., 2019), a PLGA nanoparticle with protease inhibitor has shown to overcome gastro-intestinal limitations of oral insulin delivery in rats (Faheem et al., 2019), a PLGA-chitosan based nanocarrier has been synthesized and shown to be selective for human antigen presenting cells (Durán et al., 2019), a potential DNA vaccine delivery system has been designed using a PLGA based nanocarrier (Besumbes et al., 2019), and a Vitamin D encapsulated PLGA based delivery system has recently shown activity against various markers for Alzheimer’s disease in mice (Jeon et al., 2019). Gonzalez-Pizarro et al. (2018) have prepared an optimized fluoromethalone-PLGA nanoparticle that demonstrated increased efficacy in treating ocular inflammation compared to the commercial formula. The use of some of the available methods in PLGA nanocarrier synthesis and their applications are summarized in Table 1.
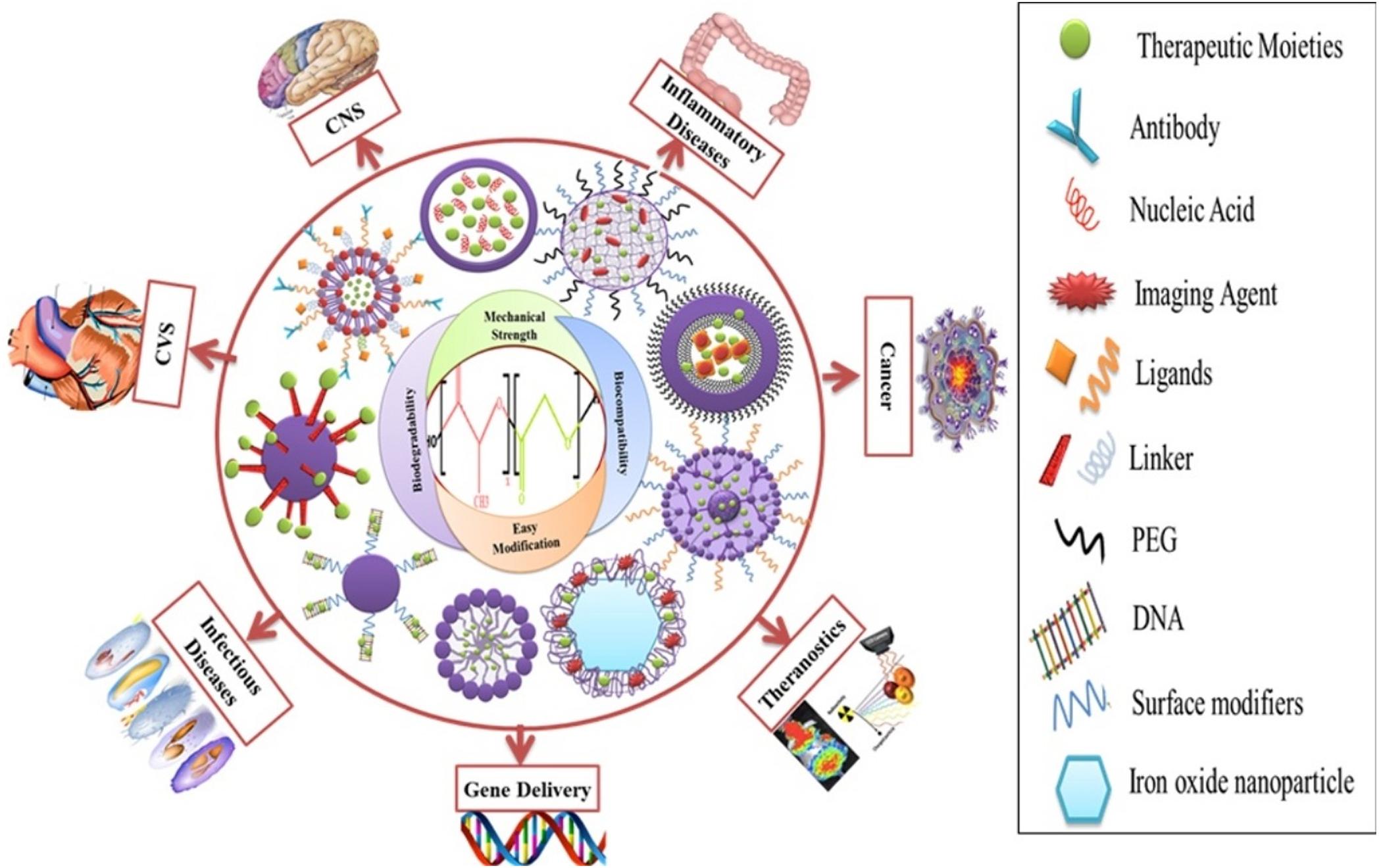
Figure 12. Functionalized PLGA nanocarriers and their medical applications, reproduced from Mir et al. (2017).
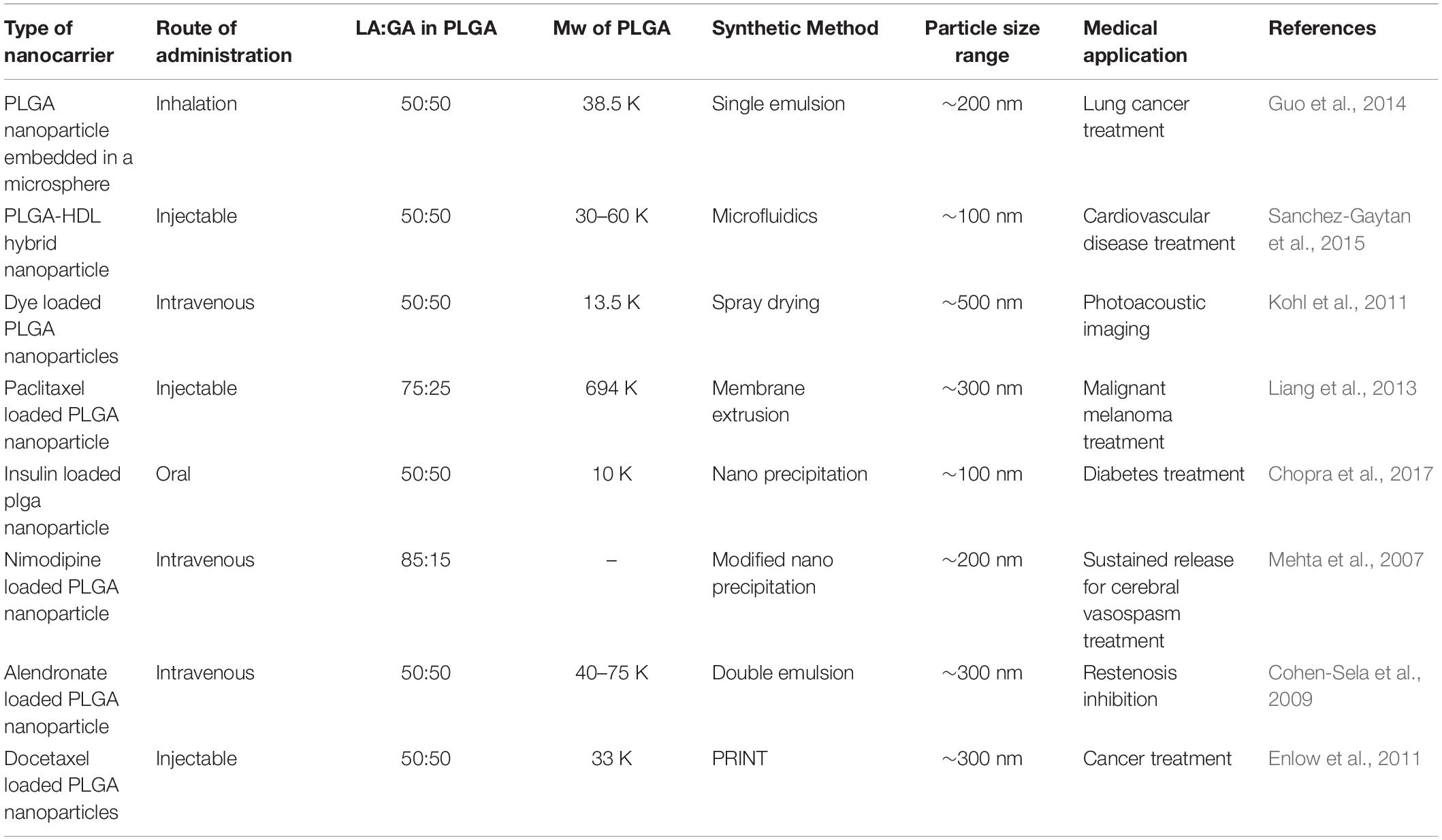
Table 1. Properties of some prepared PLGA nanocarriers, their methods of formation and biological targets.
Inclusion of PLGA Formulations in the Clinic
The biocompatibility, biodegradability and versatility of PLGA has made it suitable for a wide range of clinical applications. PLGA was commercialized in the 1970s as a suture material under the trade name Vicryl® (Kamaly et al., 2016). Other sutures include Dolphin Sutures®, and Polysorb® which are both currently approved. PLGA-containing chemotherapeutic formulations approved for clinical use include Lupron Depot®, for sustained release of leuprolide, which has application in the management of prostate cancer (Swider et al., 2018), Trelstar®, a triptorelin-containing suspension for the treatment of prostate cancer and Zoladex, a goserelin-containing implant used in the treatment of breast and prostate cancer and endometriosis. Formulations approved for other applications include Risperdal® Consta®(risperidone), Vivitrol®(naltrexone) and Arestin®(minocycline) for the treatment of schizophrenia, opioid dependence and periodontal disease respectively (Jain et al., 2016). A promising direction for clinical development is the engineering of PLGA based systems with imaging agents to monitor disease progress and/or relapse patterns using magnetic resonance imaging (MRI). Studies have shown these structures to be non-invasive and cost effective, with excellent safety profiles (Strohbehn et al., 2015). Furthermore, there are a number of PLGA based systems have been used in clinical trials that are ongoing or have been recently concluded (U.S. National Library of Medicine, 2019).
Computational Modeling
Despite the numerous formulations and methods available for PLGA synthesis, there is a large discrepancy between in vitro, in vivo and clinical results. One of the reasons for this could be the fact that it is difficult to obtain mechanistic insight into nano-formulation behavior in the various systems by evaluation of results based solely on experimental methods (Huynh et al., 2012). Because of the ubiquity of PLGA across so many biomedical fields of research, there is an abundance of data at our fingertips for computational modeling (in silico). There are various levels of detail that can be used in computational simulations. The approach used most widely for nanoparticle drug delivery systems is molecular dynamics (MDs). This technique uses the motion of the molecules in the system to predict its behavior. The parameters it uses are the bonds, bond angles and dihedrals, and here the atoms are treated as point charges. If the degree of detail of atomistic interaction is not required, a coarse grained (CG) model can be used. Here, atoms are grouped into molecular fragments and their behavior in the system is modeled (Frenkel and Smit, 2001). Density functional theory is a model based on electronic density around atoms in the system and measures these interactions within the system of interest (Geerlings et al., 2003). Computational simulations at these levels can give insight into polymer interactions, drug-carrier miscibility, drug loading, drug release, and complex stability (Ramezanpour et al., 2016). Mathematical modeling such as finite element analysis and computational flow dynamics are particular useful when studying polymeric nanoparticle formation (Lince et al., 2011), diffusion and degradation (Kojic et al., 2017). Hence, coupled with experimental methods, they can be powerful tools in the rational design of PLGA nanocarriers for biomedical applications. A study of PLGA binding to curcumin was conducted using MDs with the GROningen MAchine for Chemical Simulations (GROMACS) program, compared to laboratory findings and collated with the experimental results of 10 other PLGA-drug formulations. This study also predicted that PLGA could entrap curcumin with a higher encapsulation efficiency than tripalmitin, a lipid-based carrier, and this prediction was confirmed experimentally (Metwally and Hathout, 2015). A study on the drug release of the anti-cancer drug oxaliplatin in a PLGA matrix was conducted using the Large-scale Atomic/Molecular Massively Parallel Simulator (LAMMPS) program (Lange et al., 2016). A detailed insight into PLGA “patchy particles,” which are particles made up of PLGA and lipid-polymer groups, was obtained from computational fluid dynamics, MDs and coarse grain simulations (Salvador-Morales et al., 2016). A study involving the simulation of PLGA-PEG co-polymer with the hydrophobic drug itraconazole, as shown in Figure 13, provided information about the drug loading limitations of this system (Wilkosz et al., 2018). A MDs simulation of the peptide Melittin showed that it constituted a more stable formulation with PLGA than PLA (Asadzadeh and Moosavi, 2019). The level of these studies as well as the information they provide is summarized in Table 2.
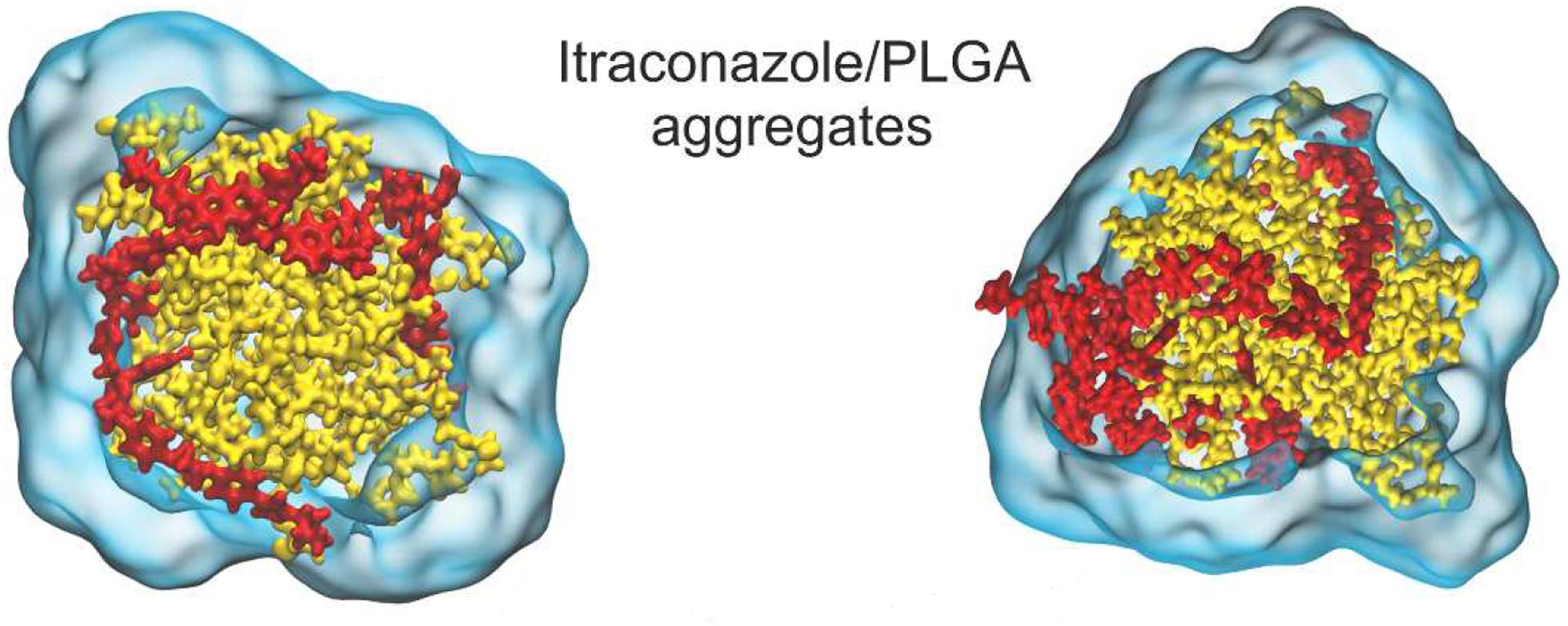
Figure 13. Molecular dynamics (MDs) simulation showing (A, left) 12% w/w and (B, right) 24% itraconazole loading in a PLGA nanoparticle, adapted with permission from Wilkosz et al. (2018). Copyright (2019) American Chemical Society.
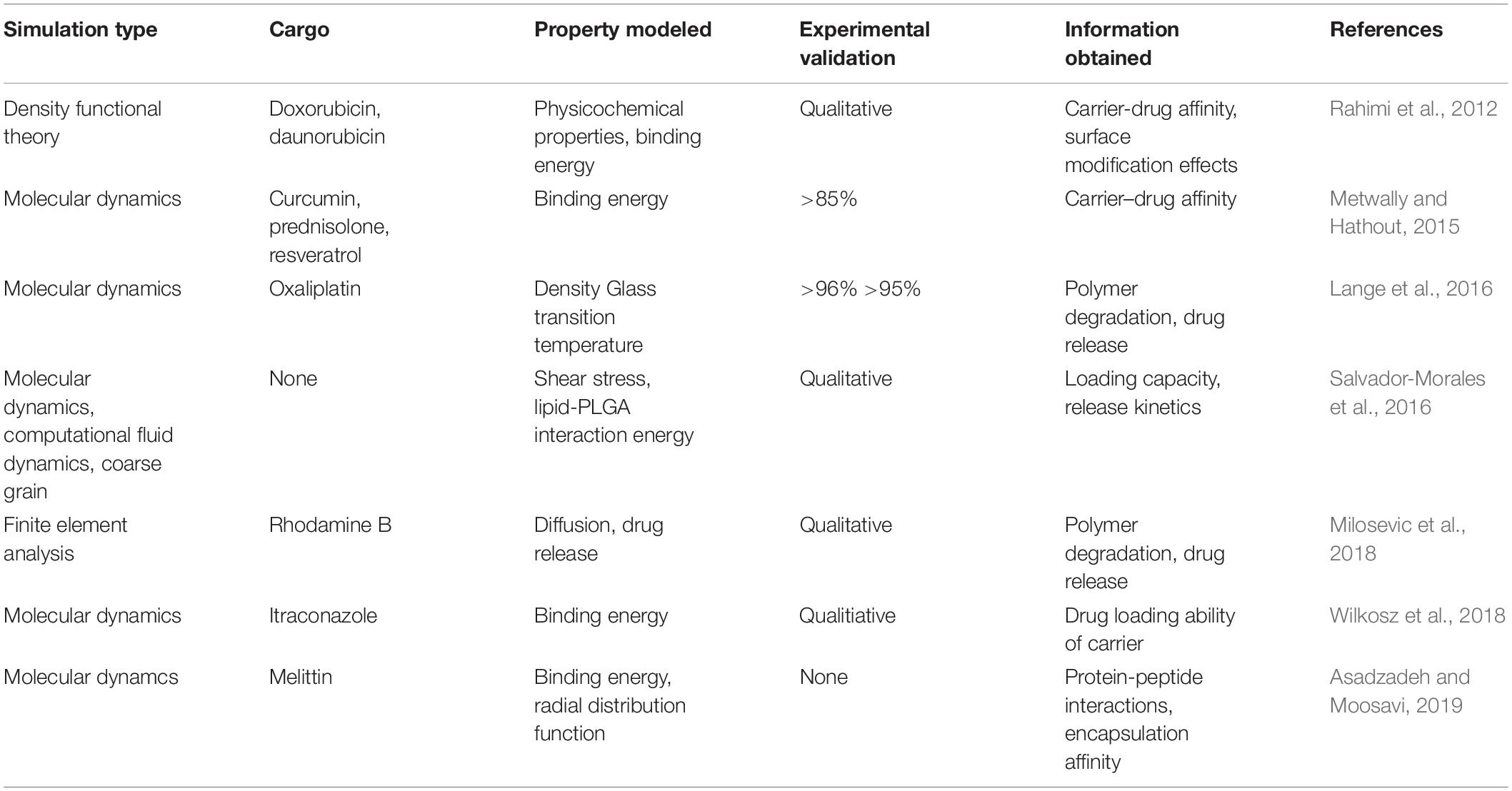
Table 2. Molecular simulations of PLGA and their significance to nanocarrier drug delivery properties.
Another area of expansion of computational modeling on PLGA nanosystems is be the study of the transport of these nanocarriers in the circulatory system. One of the limitations of the clinical translation of nanosystems in general is the poor correlation between in vitro and in vivo results. The use of mathematical and computational methods to model the interactions between the drug, carrier, biological transport system and tumor vasculature can be used to gain insight into these complexities (Curtis et al., 2015). Finite element analysis can be employed to model the dynamics of a nanoparticles within a channel, hence simulating transport in a blood vessel while continuum models can also be used to simulate nanoparticles in a vascular network generated by physical input parameters (Liu et al., 2012).
While computational simulations can provide valuable information on the molecular interactions in various PLGA nanocarrier systems, they can be limited by computational cost and time intensive calculations (Ramezanpour et al., 2016). It has been proposed that a minimum reporting standard be instituted where researchers are required to present their results with enough information to make it useful for in silico modeling and future work (Faria et al., 2018).
Computational modeling could be immensely useful once it reaches a level where it can be used to select or eliminate certain experimental variables before laboratory research is conducted. Currently, even though simulation time scales are appropriate for the modeling of several nano-systems, the detailed investigation of the formation of nanoparticles by new methods, for example microfluidics, is beyond the abilities of current computational technology. The majority of studies conducted thus far involve the modeling of individual systems; however, more data is needed so that we can move away from specific systems to create profiles to generalize these delivery systems for rational design (Ramezanpour et al., 2016).
Optimizing nanoformulations especially with PLGA polymers which have numerous possible combinations of lactic acid to glycolic acid ratio, molecular weight, endcaps and surface functionalization, could be very time consuming, expensive and in some cases not experimentally feasible. Since computational simulations give a molecular insight to macroscopic properties (Huynh et al., 2012), it could provide a platform to model these initial parameters in order to narrow down the possibilities in a specific study.
Design Considerations
Several studies have demonstrated the increase in particle size and decrease in drug release rate with increase in molecular weight and lactide:glycolide ratios (Song et al., 2008; Dinarvand et al., 2011). Recently, Lu et al. (2019) designed a 75:25 lactide:glycolide PLGA nanocarrier for the sustained release of paclitaxel. Surface functionalization is a component that needs to factor in when designing nanocarriers. Gu et al. (2008) conducted a study that optimized the in vitro release rate of docetaxel in PLGA, and additionally found that they could reduce the size of the nanoparticles from ∼291 to ∼160 nm by shortening the length of the PEG chains that were used for surface functionalization, while Bertrand et al. (2017) found that up to a point, increasing the density of PEG surface functionalization increased the blood circulation time of their nanoformulations. Gu’s group also investigated an optimum targeting ligand density in order to provide maximum targeting ability without inhibiting the shielding effect of the PEG corona (Gu et al., 2008), while Lu’s group found that increasing the density of the chitosan coating in their formulation increased the particle size from ∼133 to ∼173 nm (Lu et al., 2019).
Since the choice of fabrication methods and processes can determine the physicochemical characteristics of the resulting system, it is important to select an approach that is associated with the desired nanoparticle properties for the system of interest. For example Kim S.R. et al. (2019) reported that even though the preparation of their entacavir-loaded system by spray drying produced larger particle size diameters compared to emulsion techniques, the spray dried system showed a much more favorable drug loading and release profiles and hence was the better performing delivery system. Krishnamoorthy described a multi-criteria decision making approach to the synthesis of polymeric nanoparticles which concluded that nanoprecipitation would be the best suited preparation method for a campthothecin-loaded system (Krishnamoorthy and Mahalingam, 2015), and an adapted approach could be implemented in the selection of synthetic methods for specific PLGA-based systems.
Disadvantages of PLGA as a Nanocarrier
Even though the versatility of PLGA makes it an attractive option as a nanocarrier, it does present several challenges in nanomedicine. PLGA co-polymers are usually readily commercially available, but to obtain it in a high purity, and the specificity required for different molecular weights, lactic/glycolic acid ratios and end capped options can make it very costly (Danhier et al., 2012). Many formulations show poor drug loading and therefore would require large doses in order to achieve therapeutic concentrations of cargo at the target site. Furthermore, these systems often exhibit burst release kinetics, which would result in off target in vivo delivery. The degradation rate of PLGA is often unpredictable and the acidic degradation products have shown to affect the activity of the encapsulated drug (Sharma et al., 2016), and despite its biodegradability, reports have shown that the use of PLGA in medical devices may produce localized reaction at the site of delivery (Makadia and Siegel, 2011). Even though targeted PLGA based carriers theoretically have more efficient site specific delivery properties, the targeting moieties in these nanosystems can induce additional immunogenocity (Danhier et al., 2010). There are various in vivo physiological barriers and up- and down-regulation of cell surface receptors and other targets can also decrease the efficacy of the targeting agents in these systems. The adaptability of PLGA as a polymer for its specific application has resulted in it being used in many delivery systems and therefore it is difficult to make comprehensive predictions at this stage about its general behavior and toxicity (Sharma et al., 2016).
Conclusion and Future Work
Even though PLGA is a polymer with many desirable features, there are various areas in which research can be conducted to improve the viability of PLGA based nanocarriers for clinical translation. Since PLGA has been developed in drug delivery systems for such a wide berth of applications, it should be precisely designed in terms of cargo suitability, particle size, drug entrapment and degradation kinetics, for its specific target. This would determine the choice of starting materials and in some cases, the method of preparation and would therefore remove some of the uncertainty present in several trial-and-error attempts in previous drug delivery systems. The innovative fabrication techniques mentioned above could also be attempted to increase control over homogeneity of the products. The use of in silico modeling for PLGA nanoparticles as an element of experimental design and could have tremendous implications for the future of nanoparticle design.
Author Contributions
DE, PK, YC, and VP from designing the framework and main content of the manuscript, revisions to optimize the manuscript, approved the final submission, and the manuscript was accomplished with contributions.
Funding
This work was supported by a National Research Foundation (NRF) SARChI grant.
Conflict of Interest
The authors declare that the research was conducted in the absence of any commercial or financial relationships that could be construed as a potential conflict of interest.
References
Ali, A., and Ahmed, S. (2018). A review on chitosan and its nanocomposites in drug delivery. Int. J. Biol. Macromol. 109, 273–286. doi: 10.1016/j.ijbiomac.2017.12.078
Al-Jamal, K. T., Bai, J., Wang, J. T.-W., Protti, A., Southern, P., Bogart, L., et al. (2016). Magnetic drug targeting: preclinical in vivo studies, mathematical modeling, and extrapolation to humans. Nano Lett. 16, 5652–5660. doi: 10.1021/acs.nanolett.6b02261
Almoustafa, H. A., Alshawsh, M. A., and Chik, Z. (2017). Technical aspects of preparing PEG-PLGA nanoparticles as carrier for chemotherapeuticı agents by nanoprecipitation method. Int. J. Pharm. 533, 275–284. doi: 10.1016/j.ijpharm.2017.09.054
Alshamsan, A. (2014). Nanoprecipitation is more efficient than emulsion solvent evaporation method to encapsulate cucurbitacin I in PLGA nanoparticles. Saudi Pharm. J. 22, 219–222. doi: 10.1016/j.jsps.2013.12.002
Alvarez-Lorenzo, C., Rey-Rico, A., Sosnik, A., Taboada, P., and Concheiro, A. (2010). Poloxamine-based nanomaterials for drug delivery. Front. Biosci. 2, 424–440. doi: 10.2741/e102
Ananta, J. S., Paulmurugan, R., and Massoud, T. (2016). Temozolomide-loaded PLGA nanoparticles to treat glioblastoma cells: a biophysical and cell culture evaluation. Neurol. Res. 38, 51–59. doi: 10.1080/01616412.2015.1133025
Anderson, J. M., and Shive, M. S. (2012). Biodegradation and biocompatibility of PLA and PLGA microspheres. Adv. Drug Deliv. Rev. 64, 72–82. doi: 10.1016/j.addr.2012.09.004
Ansary, R. H., Awang, M. B., and Rahman, M. M. (2014). Biodegradable poly (D, L-lactic-co-glycolic acid)-based micro/nanoparticles for sustained release of protein drugs-A review. Trop. J. Pharm. Res. 13, 1179–1190.
Aravind, A., Nair, R., Raveendran, S., Veeranarayanan, S., Nagaoka, Y., Fukuda, T., et al. (2013). Aptamer conjugated paclitaxel and magnetic fluid loaded fluorescently tagged PLGA nanoparticles for targeted cancer therapy. J. Magn. Magn. Mater. 344, 116–123. doi: 10.1016/j.jmmm.2013.05.036
Asadzadeh, H., and Moosavi, A. (2019). Investigation of the interactions between Melittin and the PLGA and PLA polymers: molecular dynamic simulation and binding free energy calculation. Mater. Res. Exp. 6:055318. doi: 10.1088/2053-1591/ab06d3
Ayyoob, M., and Kim, Y. (2018). Effect of chemical composition variant and oxygen plasma treatments on the wettability of PLGA thin films, synthesized by direct copolycondensation. Polymers 10:E1132. doi: 10.3390/polym10101132
Baghdan, E., Raschpichler, M., Lutfi, W., Pinnapireddy, S. R., Pourasghar, M., Schäfer, J., et al. (2019). Nano spray dried antibacterial coatings for dental implants. Eur. J. Pharm. Biopharm. 139, 59–67. doi: 10.1016/j.ejpb.2019.03.003
Bauer, L. M., Situ, S. F., Griswold, M. A., and Samia, A. C. S. (2016). High-performance iron oxide nanoparticles for magnetic particle imaging–guided hyperthermia (hMPI). Nanoscale 8, 12162–12169. doi: 10.1039/c6nr01877g
Berkland, C., Pack, D. W., and Kim, K. K. (2004). Controlling surface nano-structure using flow-limited field-injection electrostatic spraying (FFESS) of poly(D,L-lactide-co-glycolide). Biomaterials 25, 5649–5658. doi: 10.1016/j.biomaterials.2004.01.018
Berthet, M., Gauthier, Y., Lacroix, C., Verrier, B., and Monge, C. (2017). Nanoparticle-based dressing: the future of wound treatment? Trends Biotechnol. 35, 770–784. doi: 10.1016/j.tibtech.2017.05.005
Bertrand, N., Grenier, P., Mahmoudi, M., Lima, E. M., Appel, E. A., Dormont, F., et al. (2017). Mechanistic understanding of in vivo protein corona formation on polymeric nanoparticles and impact on pharmacokinetics. Nat. Commun. 8:777. doi: 10.1038/s41467-017-00600-w
Besumbes, E. S., Fornaguera, C., Monge, M., García-Celma, M. J., Carrión, J., Solans, C., et al. (2019). PLGA cationic nanoparticles, obtained from nano-emulsion templating, as potential DNA vaccines. Eur. Polym. J. 120:109229. doi: 10.1016/j.eurpolymj.2019.109229
Bosch, F., and Rosich, L. J. P. (2008). The contributions of Paul Ehrlich to pharmacology: a tribute on the occasion of the centenary of his Nobel Prize. Pharmacology 82, 171–179. doi: 10.1159/000149583
Bruinsmann, F. A., Pigana, S., Aguirre, T., Dadalt Souto, G., Garrastazu Pereira, G., Bianchera, A., et al. (2019). Chitosan-coated nanoparticles: effect of chitosan molecular weight on nasal transmucosal delivery. Pharmaceutics 11:86. doi: 10.3390/pharmaceutics11020086
Carter, T., Mulholland, P., and Chester, K. (2016). Antibody-targeted nanoparticles for cancer treatment. Immunotherapy 8, 941–958. doi: 10.2217/imt.16.11
Chapman, S., Dobrovolskaia, M., Farahani, K., Goodwin, A., Joshi, A., Lee, H., et al. (2013). Nanoparticles for cancer imaging: the good, the bad, and the promise. Nano Today 8, 454–460. doi: 10.1016/j.nantod.2013.06.001
Chen, J., Wu, Q., Luo, L., Wang, Y., Zhong, Y., Dai, H. B., et al. (2017). Dual tumor-targeted poly(lactic-co-glycolic acid)-polyethylene glycol-folic acid nanoparticles: a novel biodegradable nanocarrier for secure and efficient antitumor drug delivery. Int. J. Nanomedicine 12, 5745–5760. doi: 10.2147/ijn.S136488
Chen, Q., Chen, J., Yang, Z., Xu, J., Xu, L., Liang, C., et al. (2019). Nanoparticle-enhanced radiotherapy to trigger robust cancer immunotherapy. Adv. Mater. 31:1802228. doi: 10.1002/adma.201802228
Chen, Q., Xu, L., Liang, C., Wang, C., Peng, R., and Liu, Z. (2016). Photothermal therapy with immune-adjuvant nanoparticles together with checkpoint blockade for effective cancer immunotherapy. Nat. Commun. 7:13193. doi: 10.1038/ncomms13193
Chen, Y., Yang, Z., Liu, C., Wang, C., Zhao, S., Yang, J., et al. (2013). Synthesis, characterization, and evaluation of paclitaxel loaded in six-arm star-shaped poly (lactic-co-glycolic acid). Int. J. Nanomedicine 8, 4315–4326. doi: 10.2147/IJN.S51629
Chiesa, E., Dorati, R., Pisani, S., Conti, B., Bergamini, G., Modena, T., et al. (2018). The microfluidic technique and the manufacturing of polysaccharide nanoparticles. Pharmaceutics 10:267. doi: 10.3390/pharmaceutics10040267
Choi, K. Y., Yoon, H. Y., Kim, J. H., Bae, S. M., Park, R. W., Kang, Y. M., et al. (2011). Smart nanocarrier based on PEGylated hyaluronic acid for cancer therapy. ACS Nano 5, 8591–8599. doi: 10.1021/nn202070n
Chopra, S., Bertrand, N., Lim, J. M., Wang, A., Farokhzad, O. C., and Karnik, R. (2017). Design of insulin-loaded nanoparticles enabled by multistep control of nanoprecipitation and zinc chelation. ACS Appl. Mater. Interfaces 9, 11440–11450. doi: 10.1021/acsami.6b16854
Chung, H. J., Kim, H. K., Yoon, J. J., and Park, T. G. (2006). Heparin immobilized porous PLGA microspheres for angiogenic growth factor delivery. Pharm. Res. 23, 1835–1841. doi: 10.1007/s11095-006-9039-9
Cohen-Sela, E., Chorny, M., Koroukhov, N., Danenberg, H. D., and Golomb, G. (2009). A new double emulsion solvent diffusion technique for encapsulating hydrophilic molecules in PLGA nanoparticles. J. Control. Release 133, 90–95. doi: 10.1016/j.jconrel.2008.09.073
Collins, D. J., Neild, A., deMello, A., Liu, A.-Q., and Ai, Y. (2015). The poisson distribution and beyond: methods for microfluidic droplet production and single cell encapsulation. Lab Chip 15, 3439–3459. doi: 10.1039/C5LC00614G
Coors, E. A., Seybold, H., Merk, H. F., and Mahler, V. (2005). Polysorbate 80 in medical products and nonimmunologic anaphylactoid reactions. Ann. Allergy Asthma Immunol. 95, 593–599. doi: 10.1016/s1081-1206(10)61024-1
Crucho, C. I. C., and Barros, M. T. (2017). Polymeric nanoparticles: a study on the preparation variables and characterization methods. Mater. Sci. Eng. C Mater. Biol. Appl. 80, 771–784. doi: 10.1016/j.msec.2017.06.004
Curtis, L. T., Wu, M., Lowengrub, J., Decuzzi, P., and Frieboes, H. B. (2015). Computational modeling of tumor response to drug release from vasculature-bound nanoparticles. PLoS One 10:e0144888. doi: 10.1371/journal.pone.0144888
Danhier, F., Ansorena, E., Silva, J. M., Coco, R., Le Breton, A., and Préat, V. (2012). PLGA-based nanoparticles: an overview of biomedical applications. J. Control. Release 161, 505–522. doi: 10.1016/j.jconrel.2012.01.043
Danhier, F., Feron, O., and Préat, V. (2010). To exploit the tumor microenvironment: passive and active tumor targeting of nanocarriers for anti-cancer drug delivery. J. Control. Release 148, 135–146. doi: 10.1016/j.jconrel.2010.08.027
Davoudi, Z., Peroutka-Bigus, N., Bellaire, B., Wannemuehler, M., Barrett, T. A., Narasimhan, B., et al. (2018). Intestinal organoids containing poly(lactic-co-glycolic acid) nanoparticles for the treatment of inflammatory bowel diseases. J. Biomed. Mater. Res. Part A 106, 876–886. doi: 10.1002/jbm.a.36305
Dinarvand, R., Sepehri, N., Manoochehri, S., Rouhani, H., and Atyabi, F. (2011). Polylactide-co-glycolide nanoparticles for controlled delivery of anticancer agents. Int. J. Nanomedicine 6, 877–895. doi: 10.2147/IJN.S18905
Ding, D., and Zhu, Q. (2018). Recent advances of PLGA micro/nanoparticles for the delivery of biomacromolecular therapeutics. Mater. Sci. Eng. C Mater. Biol. Appl. 92, 1041–1060. doi: 10.1016/j.msec.2017.12.036
Du, J., Li, X.-Y., Hu, H., Xu, L., Yang, S.-P., and Li, F.-H. (2018). Preparation and imaging investigation of dual-targeted C 3 F 8-filled PLGA nanobubbles as a novel ultrasound contrast agent for breast cancer. Sci. Rep. 8:3887.
Durán, V., Yasar, H., Becker, J., Thiyagarajan, D., Loretz, B., Kalinke, U., et al. (2019). Preferential uptake of chitosan-coated PLGA nanoparticles by primary human antigen presenting cells. Nanomedicine 21:102073. doi: 10.1016/j.nano.2019.102073
Eley, J. G., Pujari, V. D., and McLane, J. (2004). Poly (lactide-co-glycolide) nanoparticles containing coumarin-6 for suppository delivery: in vitro release profile and in vivo tissue distribution. Drug Deliv. 11, 255–261. doi: 10.1080/10717540490467384
Engineer, C., Parikh, J., Raval, A., and Organs, A. (2011). Review on hydrolytic degradation behavior of biodegradable polymers from controlled drug delivery system. Trends Biomater. Artif. Organs 25, 79–85. doi: 10.1002/wnan.10
Enlow, E. M., Luft, J. C., Napier, M. E., and DeSimone, J. M. (2011). Potent engineered PLGA nanoparticles by virtue of exceptionally high chemotherapeutic loadings. Nano Lett. 11, 808–813. doi: 10.1021/nl104117p
Faheem, A. M., Abdeåkader, D., Osman, M. A., McCarron, P. A., and El-Gizawy, S. A. (2019). Oral insulin delivery in diabetic rats by PLGA nanoparticles combined with a protease inhibitor (N-ethylmaleimide). Br. J. Pharm. 4, S2–3.
Faria, M., Björnmalm, M., Thurecht, K. J., Kent, S. J., Parton, R. G., Kavallaris, M., et al. (2018). Minimum information reporting in bio–nano experimental literature. Nat. Nanotechnol. 13, 777–785. doi: 10.1038/s41565-018-0246-4
Fessi, H., Puisieux, F., Devissaguet, J. P., Ammoury, N., and Benita, S. (1989). Nanocapsule formation by interfacial polymer deposition following solvent displacement. Int. J. Pharm. 55, R1–R4. doi: 10.1016/0378-5173(89)90281-0
Frenkel, D., and Smit, B. (2001). Understanding Molecular Simulation: From Algorithms to Applications, Vol. 1. San Diego, CA: Elsevier, 363.
Friedman, A. D., Claypool, S. E., and Liu, R. (2013). The smart targeting of nanoparticles. Curr. Pharm. Des. 19, 6315–6329. doi: 10.2174/13816128113199990375
Fu, X., Cai, J., Zhang, X., Li, W.-D., Ge, H., and Hu, Y. (2018). Top-down fabrication of shape-controlled, monodisperse nanoparticles for biomedical applications. Adv. Drug Deliv. Rev. 132, 169–187. doi: 10.1016/j.addr.2018.07.006
Gajdova, M., Jakubovsky, J., and Valky, J. (1993). Delayed effects of neonatal exposure to tween 80 on female reproductive organs in rats. Food Chem. Toxicol. 31, 183–190. doi: 10.1016/0278-6915(93)90092-d
Ganipineni, L. P., Ucakar, B., Joudiou, N., Riva, R., Jérôme, C., Gallez, B., et al. (2019). Paclitaxel-loaded multifunctional nanoparticles for the targeted treatment of glioblastoma. J. Drug Target. 27, 614–623. doi: 10.1080/1061186X.2019.1567738
Geerlings, P., De Proft, F., and Langenaeker, W. (2003). Conceptual density functional theory. Chem. Rev. 103, 1793–1873. doi: 10.1021/cr990029p
Gendelman, H. E., Anantharam, V., Bronich, T., Ghaisas, S., Jin, H., Kanthasamy, A. G., et al. (2015). Nanoneuromedicines for degenerative, inflammatory, and infectious nervous system diseases. Nanomedicine 11, 751–767. doi: 10.1016/j.nano.2014.12.014
Gentile, P., Chiono, V., Carmagnola, I., and Hatton, P. (2014). An overview of poly (lactic-co-glycolic) acid (PLGA)-based biomaterials for bone tissue engineering. Int. J. Mol. Sci. 15, 3640–3659. doi: 10.3390/ijms15033640
Gholizadeh, S., Kamps, J. A. A. M., Hennink, W. E., and Kok, R. J. (2018). PLGA-PEG nanoparticles for targeted delivery of the mTOR/PI3kinase inhibitor dactolisib to inflamed endothelium. Int. J. Pharm. 548, 747–758. doi: 10.1016/j.ijpharm.2017.10.032
Gonzalez-Pizarro, R., Silva-Abreu, M., Calpena, A. C., Egea, M. A., Espina, M., and Garcia, M. L. (2018). Development of fluorometholone-loaded PLGA nanoparticles for treatment of inflammatory disorders of anterior and posterior segments of the eye. Int. J. Pharm. 547, 338–346. doi: 10.1016/j.ijpharm.2018.05.050
Govender, T., Stolnik, S., Garnett, M. C., Illum, L., and Davis, S. S. (1999). PLGA nanoparticles prepared by nanoprecipitation: drug loading and release studies of a water soluble drug. J. Control. Release 57, 171–185. doi: 10.1016/s0168-3659(98)00116-3
Grabowski, N., Hillaireau, H., Vergnaud, J., Tsapis, N., Pallardy, M., Kerdine-Romer, S., et al. (2015). Surface coating mediates the toxicity of polymeric nanoparticles towards human-like macrophages. Int. J. Pharm. 482, 75–83. doi: 10.1016/j.ijpharm.2014.11.042
Grabowski, N., Hillaireau, H., Vergnaud-Gauduchon, J., Nicolas, V., Tsapis, N., Kerdine-Romer, S., et al. (2016). Surface-modified biodegradable nanoparticles’ impact on cytotoxicity and inflammation response on a co-culture of lung epithelial cells and human-like macrophages. J. Biomed. Nanotechnol. 12, 135–146. doi: 10.1166/jbn.2016.2126
Gu, F., Zhang, L., Teply, B. A., Mann, N., Wang, A., Radovic-Moreno, A. F., et al. (2008). Precise engineering of targeted nanoparticles by using self-assembled biointegrated block copolymers. Proc. Natl. Acad. Sci. U.S.A. 105, 2586–2591. doi: 10.1073/pnas.0711714105
Guo, L., Chen, B., Liu, R., Xia, G., Wang, Y., Li, X., et al. (2015). Biocompatibility assessment of polyethylene glycol-poly L-lysine-poly lactic-co-glycolic acid nanoparticles in vitro and in vivo. J. Nanosci. Nanotechnol. 15, 3710–3719. doi: 10.1166/jnn.2015.9509
Guo, P., Huang, J., Zhao, Y., Martin, C. R., Zare, R. N., and Moses, M. A. (2018). Nanomaterial preparation by extrusion through nanoporous membranes. Small 14:e1703493. doi: 10.1002/smll.201703493
Guo, X., Zhang, X., Ye, L., Zhang, Y., Ding, R., Hao, Y., et al. (2014). Inhalable microspheres embedding chitosan-coated PLGA nanoparticles for 2-methoxyestradiol. J. Drug Target. 22, 421–427. doi: 10.3109/1061186x.2013.878944
Guo, Z. S. (2018). The 2018 Nobel Prize in medicine goes to cancer immunotherapy (editorial for BMC cancer). BMC Cancer 18:1086. doi: 10.1186/s12885-018-5020-3
Habraken, W. J., Wolke, J. G., Mikos, A. G., and Jansen, J. A. (2006). Injectable PLGA microsphere/calcium phosphate cements: physical properties and degradation characteristics. J. Biomater. Sci. Polym. Ed. 17, 1057–1074. doi: 10.1163/156856206778366004
Haggag, Y. A., Faheem, A. M., Tambuwala, M. M., Osman, M. A., El-Gizawy, S. A., O’Hagan, B., et al. (2018). Effect of poly(ethylene glycol) content and formulation parameters on particulate properties and intraperitoneal delivery of insulin from PLGA nanoparticles prepared using the double-emulsion evaporation procedure. Pharm. Dev. Technol. 23, 370–381. doi: 10.1080/10837450.2017.1295066
Heinz, H., Pramanik, C., Heinz, O., Ding, Y., Mishra, R. K., Marchon, D., et al. (2017). Nanoparticle decoration with surfactants: molecular interactions, assembly, and applications. Surf. Sci. Rep. 72, 1–58. doi: 10.1016/j.surfrep.2017.02.001
Huynh, L., Neale, C., Pomès, R., and Allen, C. (2012). Computational approaches to the rational design of nanoemulsions, polymeric micelles, and dendrimers for drug delivery. Nanomedicine 8, 20–36. doi: 10.1016/j.nano.2011.05.006
Jahan, S. T., Sadat, S. M. A., Walliser, M., and Haddadi, A. (2017). Targeted therapeutic nanoparticles: an immense promise to fight against cancer. J. Drug Deliv. 2017:9090325. doi: 10.1155/2017/9090325
Jain, A., Kunduru, K. R., Basu, A., Mizrahi, B., Domb, A. J., and Khan, W. (2016). Injectable formulations of poly(lactic acid) and its copolymers in clinical use. Adv. Drug Deliv. Rev. 107, 213–227. doi: 10.1016/j.addr.2016.07.002
Jalali, N., Moztarzadeh, F., Mozafari, M., Asgari, S., Motevalian, M., Alhosseini, S. N. J. C., et al. (2011). Surface modification of poly (lactide-co-glycolide) nanoparticles by d-α-tocopheryl polyethylene glycol 1000 succinate as potential carrier for the delivery of drugs to the brain. Colloids Surf. A Physicochem. Eng. Asp. 392, 335–342. doi: 10.1016/j.colsurfa.2011.10.012
Jana, S., Kumar Sen, K., and Gandhi, A. (2016). Alginate based nanocarriers for drug delivery applications. Curr. Pharm. Des. 22, 3399–3410. doi: 10.2174/1381612822666160510125718
Jeon, S. G., Cha, M.-Y., Kim, J.-I., Hwang, T. W., Kim, K. A., Kim, T. H., et al. (2019). Vitamin D-binding protein-loaded PLGA nanoparticles suppress Alzheimer’s disease-related pathology in 5XFAD mice. Nanomedicine 17, 297–307. doi: 10.1016/j.nano.2019.02.004
Jesus, S., Schmutz, M., Som, C., Borchard, G., Wick, P., and Borges, O. (2019). Hazard assessment of polymeric nanobiomaterials for drug delivery: what can we learn from literature so far. Front. Bioeng. Biotechnol. 7:261. doi: 10.3389/fbioe.2019.00261
Jog, R., and Burgess, D. J. (2017). Pharmaceutical amorphous nanoparticles. J. Pharm. Sci. 106, 39–65. doi: 10.1016/j.xphs.2016.09.014
Kakkar, A., Traverso, G., Farokhzad, O. C., Weissleder, R., and Langer, R. (2017). Evolution of macromolecular complexity in drug delivery systems. Nat. Rev. Chem. 1:0063. doi: 10.1038/s41570-017-0063
Kalombo, L., Lemmer, Y., Semete-Makokotlela, B., Ramalapa, B., Nkuna, P., Booysen, L. L., et al. (2019). Spray-dried, nanoencapsulated, multi-drug anti-tuberculosis therapy aimed at once weekly administration for the duration of treatment. Nanomaterials 9:1167. doi: 10.3390/nano9081167
Kamaly, N., Yameen, B., Wu, J., and Farokhzad, O. C. (2016). Degradable controlled-release polymers and polymeric nanoparticles: mechanisms of controlling drug release. Chem. Rev. 116, 2602–2663. doi: 10.1021/acs.chemrev.5b00346
Kapoor, D. N., Bhatia, A., Kaur, R., Sharma, R., Kaur, G., and Dhawan, S. (2015). PLGA: a unique polymer for drug delivery. Ther. Deliv. 6, 41–58. doi: 10.4155/tde.14.91
Karra, N., and Benita, S. (2012). The ligand nanoparticle conjugation approach for targeted cancer therapy. Curr. Drug Metab. 13, 22–41. doi: 10.2174/138920012798356899
Keles, H., Naylor, A., Clegg, F., and Sammon, C. (2015). Investigation of factors influencing the hydrolytic degradation of single PLGA microparticles. Polym. Degrad. Stab. 119, 228–241. doi: 10.1016/j.polymdegradstab.2015.04.025
Khalil, D. N., Smith, E. L., Brentjens, R. J., and Wolchok, J. D. (2016). The future of cancer treatment: immunomodulation, CARs and combination immunotherapy. Nat. Rev. Clin. Oncol. 13, 273–290. doi: 10.1038/nrclinonc.2016.25
Khalil, N. M., do Nascimento, T. C., Casa, D. M., Dalmolin, L. F., de Mattos, A. C., Hoss, I., et al. (2013). Pharmacokinetics of curcumin-loaded PLGA and PLGA-PEG blend nanoparticles after oral administration in rats. Colloids Surf. B Biointerfaces 101, 353–360. doi: 10.1016/j.colsurfb.2012.06.024
Kim, K. T., Lee, J. Y., Kim, D. D., Yoon, I. S., and Cho, H. J. (2019). Recent progress in the development of poly(lactic-co-glycolic acid)-based nanostructures for cancer imaging and therapy. Pharmaceutics 11:E280. doi: 10.3390/pharmaceutics11060280
Kim, S. R., Ho, M. J., Choi, Y. W., and Kang, M. J. (2019). Improved drug loading and sustained release of entecavir-loaded PLGA microsphere prepared by spray drying technique. Bull. Korean Chem. Soc. 40, 306–312. doi: 10.1002/bkcs.11682
Kohl, Y., Kaiser, C., Bost, W., Stracke, F., Fournelle, M., Wischke, C., et al. (2011). Preparation and biological evaluation of multifunctional PLGA-nanoparticles designed for photoacoustic imaging. Nanomedicine 7, 228–237. doi: 10.1016/j.nano.2010.07.006
Kojic, M., Milosevic, M., Simic, V., Stojanovic, D., and Uskokovic, P. (2017). A radial 1D finite element for drug release from drug loaded nanofibers. J. Serbian Soc. Comput. Mech. 11, 82–93. doi: 10.24874/jsscm.2017.11.01.08
Koushik, K., and Kompella, U. B. (2004). Preparation of large porous deslorelin-PLGA microparticles with reduced residual solvent and cellular uptake using a supercritical carbon dioxide process. Pharm. Res. 21, 524–535. doi: 10.1023/B:PHAM.0000019308.25479.a4
Krishnamoorthy, K., and Mahalingam, M. (2015). Selection of a suitable method for the preparation of polymeric nanoparticles: multi-criteria decision making approach. Adv. Pharm. Bull. 5, 57–67. doi: 10.5681/apb.2015.008
Kumar, P., Van Treuren, T., Ranjan, A. P., Chaudhary, P., and Vishwanatha, J. K. J. N. (2019). In vivo imaging and biodistribution of near infrared dye loaded brain-metastatic-breast-cancer-cell-membrane coated polymeric nanoparticles. Nanotechnology 30:265101. doi: 10.1088/1361-6528/ab0f46
Kumari, A., Yadav, S. K., and Yadav, S. C. (2010). Biodegradable polymeric nanoparticles based drug delivery systems. Colloids Surf. B Biointerfaces 75, 1–18. doi: 10.1016/j.colsurfb.2009.09.001
Lai, P., Daear, W., Löbenberg, R., and Prenner, E. J. (2014). Overview of the preparation of organic polymeric nanoparticles for drug delivery based on gelatine, chitosan, poly (d, l-lactide-co-glycolic acid) and polyalkylcyanoacrylate. Colloids Surf. B Biointerfaces 118, 154–163. doi: 10.1016/j.colsurfb.2014.03.017
Lamprecht, A., Ubrich, N., Yamamoto, H., Schafer, U., Takeuchi, H., Maincent, P., et al. (2001). Biodegradable nanoparticles for targeted drug delivery in treatment of inflammatory bowel disease. J. Pharmacol. Exp. Ther. 299, 775–781.
Lange, J., de Souza, F. G., Nele, M., Tavares, F. W., Segtovich, I. S. V., and da Silva, G. C. Q. (2016). Molecular dynamic simulation of oxaliplatin diffusion in poly (lactic acid-co-glycolic acid). part a: parameterization and validation of the force-field CVFF. Macromol. Theory Simul. 25, 45–62. doi: 10.1002/mats.201500049
Li, S. (1999). Hydrolytic degradation characteristics of aliphatic polyesters derived from lactic and glycolic acids. J. Biomed. Mater. Res. 48, 342–353. doi: 10.1002/(sici)1097-4636(1999)48:3<342::aid-jbm20>3.0.co;2-7
Liang, R., Wang, J., Wu, X., Dong, L., Deng, R., Wang, K., et al. (2013). Multifunctional biodegradable polymer nanoparticles with uniform sizes: generation and in vitro anti-melanoma activity. Nanotechnology 24:455302. doi: 10.1088/0957-4484/24/45/455302
Lima, I. A. D., Khalil, N. M., Tominaga, T. T., Lechanteur, A., Sarmento, B., and Mainardes, R. M. (2018). Mucoadhesive chitosan-coated PLGA nanoparticles for oral delivery of ferulic acid. Artif. Cells Nanomed. Biotechnol. 46(Suppl. 2), 993–1002. doi: 10.1080/21691401.2018.1477788
Lince, F., Marchisio, D. L., and Barresi, A. A. (2011). A comparative study for nanoparticle production with passive mixers via solvent-displacement: use of CFD models for optimization and design. Chem. Eng. Process. 50, 356–368. doi: 10.1016/j.cep.2011.02.015
Liu, Y., Shah, S., and Tan, J. (2012). Computational modeling of nanoparticle targeted drug delivery. Rev. Nanosci. Nanotechnol. 1, 66–83. doi: 10.1166/rnn.2012.1014
Lombardo, D., Kiselev, M. A., and Caccamo, M. T. (2019). Smart nanoparticles for drug delivery application: development of versatile nanocarrier platforms in biotechnology and nanomedicine. J. Nanomater. 2019:3702518.
Lu, B., Lv, X., and Le, Y. (2019). Chitosan-modified PLGA nanoparticles for control-released drug delivery. Polymers 11:304. doi: 10.3390/polym11020304
Lü, J.-M., Wang, X., Marin-Muller, C., Wang, H., Lin, P. H., Yao, Q., et al. (2009). Current advances in research and clinical applications of PLGA-based nanotechnology. Expert Rev. Mol. Diagn. 9, 325–341. doi: 10.1586/erm.09.15
Mahapatro, A., and Singh, D. K. (2011). Biodegradable nanoparticles are excellent vehicle for site directed in-vivo delivery of drugs and vaccines. J. Nanobiotechnol. 9:55. doi: 10.1186/1477-3155-9-55
Makadia, H. K., and Siegel, S. (2011). Poly lactic-co-glycolic acid (PLGA) as biodegradable controlled drug delivery carrier. Polymers 3, 1377–1397. doi: 10.3390/polym3031377
Mandal, S., Prathipati, P. K., Belshan, M., and Destache, C. (2019). A potential long-acting bictegravir loaded nano-drug delivery system for HIV-1 infection: a proof-of-concept study. Antiviral Res. 167, 83–88. doi: 10.1016/j.antiviral.2019.04.007
Martins, C., Araújo, F., Gomes, M. J., Fernandes, C., Nunes, R., Li, W., et al. (2019). Using microfluidic platforms to develop CNS-targeted polymeric nanoparticles for HIV therapy. Eur. J. Pharm. Biopharm. 138, 111–124. doi: 10.1016/j.ejpb.2018.01.014
Martins, C., and Sarmento, B. (2020). Microfluidic manufacturing of multitargeted PLGA/PEG nanoparticles for delivery of taxane chemotherapeutics. Methods Mol. Biol. 2059, 213–224. doi: 10.1007/978-1-4939-9798-5_11
Mehta, A. K., Yadav, K. S., and Sawant, K. K. (2007). Nimodipine loaded PLGA nanoparticles: formulation optimization using factorial design, characterization and in vitro evaluation. Curr. Drug Deliv. 4, 185–193. doi: 10.2174/156720107781023929
Menon, J. U., Kona, S., Wadajkar, A. S., Desai, F., Vadla, A., and Nguyen, K. T. (2012). Effects of surfactants on the properties of PLGA nanoparticles. J. Biomed. Mater. Rese. Part A 100, 1998–2005. doi: 10.1002/jbm.a.34040
Metwally, A. A., and Hathout, R. M. (2015). Computer-assisted drug formulation design: novel approach in drug delivery. Mol. Pharm. 12, 2800–2810. doi: 10.1021/mp500740d
Middleton, J. C., and Tipton, A. (2000). Synthetic biodegradable polymers as orthopedic devices. Biomaterials 21, 2335–2346. doi: 10.1016/s0142-9612(00)00101-0
Miller, S. C., and Drabik, B. R. (1984). Rheological properties of poloxamer vehicles. Int. J. Pharm. 18, 269–276. doi: 10.1016/0378-5173(84)90142-x
Milosevic, M., Stojanovic, D., Simic, V., Milicevic, B., Radisavljevic, A., Uskokovic, P., et al. (2018). A computational model for drug release from PLGA implant. Materials 11:E2416. doi: 10.3390/ma11122416
Mir, M., Ahmed, N., and Ur Rehman, A. (2017). Recent applications of PLGA based nanostructures in drug delivery. Colloids Surf. B Biointerfaces 159, 217–231. doi: 10.1016/j.colsurfb.2017.07.038
Mishima, K. (2008). Biodegradable particle formation for drug and gene delivery using supercritical fluid and dense gas. Adv. Drug Deliv. Rev. 60, 411–432. doi: 10.1016/j.addr.2007.02.003
Mishra, A., Singh, S. K., Dash, D., Aswal, V. K., Maiti, B., Misra, M., et al. (2014). Self-assembled aliphatic chain extended polyurethane nanobiohybrids: emerging hemocompatible biomaterials for sustained drug delivery. Acta Biomater. 10, 2133–2146. doi: 10.1016/j.actbio.2013.12.035
Mittal, G., Sahana, D. K., Bhardwaj, V., and Ravi Kumar, M. N. (2007). Estradiol loaded PLGA nanoparticles for oral administration: effect of polymer molecular weight and copolymer composition on release behavior in vitro and in vivo. J. Control. Release 119, 77–85. doi: 10.1016/j.jconrel.2007.01.016
Moku, G., Layek, B., Trautman, L., Putnam, S., Panyam, J., and Prabha, S. J. C. (2019). Improving payload capacity and anti-tumor efficacy of mesenchymal stem cells using TAT peptide functionalized polymeric nanoparticles. Cancers 11:E491. doi: 10.3390/cancers11040491
Muhamad, N., Plengsuriyakarn, T., and Na-Bangchang, K. (2018). Application of active targeting nanoparticle delivery system for chemotherapeutic drugs and traditional/herbal medicines in cancer therapy: a systematic review. Int. J. Nanomedicine 13, 3921–3935. doi: 10.2147/ijn.S165210
Nagavarma, B., Yadav, H. K., Ayaz, A., Vasudha, L., and Shivakumar, H. J. A. (2012). Different techniques for preparation of polymeric nanoparticles-a review. Asian J. Pharm. Clin. Res. 5, 16–23.
Nie, H., Lee, L. Y., Tong, H., and Wang, C.-H. (2008). PLGA/chitosan composites from a combination of spray drying and supercritical fluid foaming techniques: new carriers for DNA delivery. J. Control. Release 129, 207–214. doi: 10.1016/j.jconrel.2008.04.018
Ospina-Villa, J. D., Gómez-Hoyos, C., Zuluaga-Gallego, R., and Triana-Chávez, O. (2019). Encapsulation of proteins from Leishmania panamensis into PLGA particles by a single emulsion-solvent evaporation method. J. Microbiol. Methods 162, 1–7. doi: 10.1016/j.mimet.2019.05.004
Owens, D. E. III, and Peppas, N. A. (2006). Opsonization, biodistribution, and pharmacokinetics of polymeric nanoparticles. Int. J. Pharm. 307, 93–102. doi: 10.1016/j.ijpharm.2005.10.010
Oz, U. C., Küçüktürkmen, B., Devrim, B., Saka, O. M., and Bozkir, A. (2019). Development and optimization of alendronate sodium loaded PLGA nanoparticles by central composite design. Macromol. Res. 27, 857–866. doi: 10.1007/s13233-019-7119-z
Panda, J., Satapathy, B. S., Majumder, S., Sarkar, R., Mukherjee, B., Tudu, B., et al. (2019). Engineered polymeric iron oxide nanoparticles as potential drug carrier for targeted delivery of docetaxel to breast cancer cells. J. Magn. Magn. Mater. 485, 165–173. doi: 10.1016/j.jmmm.2019.04.058
Panyam, J., and Labhasetwar, V. (2012). Biodegradable nanoparticles for drug and gene delivery to cells and tissue. Adv. Drug Deliv. Rev. 64, 61–71. doi: 10.1016/j.addr.2012.09.023
Patel, B. K., Parikh, R. H., and Patel, N. (2018). Targeted delivery of mannosylated-PLGA nanoparticles of antiretroviral drug to brain. Int. J. Nanomedicine 13, 97–100. doi: 10.2147/IJN.S124692
Peng, P., Yang, K., Tong, G., and Ma, L. (2018). Polysaccharide nanoparticles for targeted cancer therapies. Curr. Drug Metab. 19, 781–792. doi: 10.2174/1389200219666180511153403
Pereira, A. D. S. B. F., Brito, G. A. D. C., Lima, M. L. D. S., Silva Júnior, A. A. D., Silva, E. D. S., de Rezende, A. A., et al. (2018). Metformin hydrochloride-loaded PLGA nanoparticle in periodontal disease experimental model using diabetic rats. Int. J. Mol. Sci. 19:3488. doi: 10.3390/ijms19113488
Perry, J. L., Herlihy, K. P., Napier, M. E., and Desimone, J. M. (2011). PRINT: a novel platform toward shape and size specific nanoparticle theranostics. ACC Chem. Res. 44, 990–998. doi: 10.1021/ar2000315
Platel, A., Carpentier, R., Becart, E., Mordacq, G., Betbeder, D., and Nesslany, F. (2016). Influence of the surface charge of PLGA nanoparticles on their in vitro genotoxicity, cytotoxicity, ROS production and endocytosis. J. Appl. Toxicol. 36, 434–444. doi: 10.1002/jat.3247
Rahimi, F., Bahlake, A., Chamani, Z., and Bagheri, S. (2012). Theoretical study on the conjugation of PLGA and PLGA-PEG carriers to doxorubicin and daunorubicin. Indian J. Exp. Biol. 2, 2055–2060.
Ramezanpour, M., Leung, S., Delgado-Magnero, K., Bashe, B., Thewalt, J., and Tieleman, D. (2016). Computational and experimental approaches for investigating nanoparticle-based drug delivery systems. Biochim. Biophys. Acta 1858(7 Pt B), 1688–1709. doi: 10.1016/j.bbamem.2016.02.028
Redhead, H. M., Davis, S. S., and Illum, L. (2001). Drug delivery in poly(lactide-co-glycolide) nanoparticles surface modified with poloxamer 407 and poloxamine 908: in vitro characterisation and in vivo evaluation. J. Control. Release 70, 353–363. doi: 10.1016/s0168-3659(00)00367-9
Rezvantalab, S., Drude, N. I., Moraveji, M. K., Güvener, N., Koons, E. K., Shi, Y., et al. (2018). PLGA-based nanoparticles in cancer treatment. Front. Pharmacol. 9:1260. doi: 10.3389/fphar.2018.01260
Rizvi, S. A. A., and Saleh, A. M. (2018). Applications of nanoparticle systems in drug delivery technology. Saudi Pharm. J. 26, 64–70. doi: 10.1016/j.jsps.2017.10.012
Rodriguez-Torres, M. D. P., Acosta-Torres, L. S., and Diaz-Torres, L. A. (2018). Heparin-based nanoparticles: an overview of their applications. J. Nanomater. 2018:9780489. doi: 10.1155/2018/9780489
Sadat Tabatabaei Mirakabad, F., Nejati-Koshki, K., Akbarzadeh, A., Yamchi, M. R., Milani, M., Zarghami, N., et al. (2014). PLGA-based nanoparticles as cancer drug delivery systems. Asian Pac. J. Cancer Prev. 15, 517–535. doi: 10.7314/apjcp.2014.15.2.517
Sadeghi-Avalshahr, A., Nokhasteh, S., Molavi, A. M., Khorsand-Ghayeni, M., and Mahdavi-Shahri, M. (2017). Synthesis and characterization of collagen/PLGA biodegradable skin scaffold fibers. Regen. Biomater. 4, 309–314. doi: 10.1093/rb/rbx026
Salmaso, S., and Caliceti, P. (2013). Stealth properties to improve therapeutic efficacy of drug nanocarriers. Nanotechnol. Cancer 2013:374252.
Salvador-Morales, C., Brahmbhatt, B., Márquez-Miranda, V., Araya-Duran, I., Canan, J., Gonzalez-Nilo, F., et al. (2016). Mechanistic studies on the self-assembly of PLGA patchy particles and their potential applications in biomedical imaging. Langmuir 32, 7929–7942. doi: 10.1021/acs.langmuir.6b02177
Sanchez-Gaytan, B. L., Fay, F., Lobatto, M. E., Tang, J., Ouimet, M., Kim, Y., et al. (2015). HDL-mimetic PLGA nanoparticle to target atherosclerosis plaque macrophages. Bioconjug. Chem. 26, 443–451. doi: 10.1021/bc500517k
Saravanakumar, K., Hu, X., Shanmugam, S., Chelliah, R., Sekar, P., Oh, D.-H., et al. (2019). Enhanced cancer therapy with pH-dependent and aptamer functionalized doxorubicin loaded polymeric (poly D, L-lactic-co-glycolic acid) nanoparticles. Arch. Biochem. Biophys. 671, 143–151. doi: 10.1016/j.abb.2019.07.004
Schrurs, F., and Lison, D. (2012). Join the dialogue. Nat. Nanotechnol. 7, 545–545. doi: 10.1038/nnano.2012.150
Semete, B., Booysen, L., Lemmer, Y., Kalombo, L., Katata, L., Verschoor, J., et al. (2010). In vivo evaluation of the biodistribution and safety of PLGA nanoparticles as drug delivery systems. Nanomedicine 6, 662–671. doi: 10.1016/j.nano.2010.02.002
Sequeira, J. A. D., Santos, A. C., Serra, J., Veiga, F., and Ribeiro, A. J. (2018). “Chapter 10 - Poly(lactic-co-glycolic acid) (PLGA) matrix implants,” in Nanostructures for the Engineering of Cells, Tissues and Organs, ed. A. M. Grumezescu, (Norwich, NY: William Andrew Publishing), 375–402. doi: 10.1016/b978-0-12-813665-2.00010-7
Sharma, M., Sharma, S., Sharma, V., Sharma, K., Yadav, S. K., Dwivedi, P., et al. (2017). Oleanolic-bioenhancer coloaded chitosan modified nanocarriers attenuate breast cancer cells by multimode mechanism and preserve female fertility. Int. J. Biol. Macromol. 104(Pt A), 1345–1358. doi: 10.1016/j.ijbiomac.2017.06.005
Sharma, S., Parmar, A., Kori, S., and Sandhir, R. (2016). PLGA-based nanoparticles: a new paradigm in biomedical applications. TrAC Trends Anal. Chem. 80, 30–40. doi: 10.1016/j.trac.2015.06.014
Shembekar, N., Chaipan, C., Utharala, R., and Merten, C. A. (2016). Droplet-based microfluidics in drug discovery, transcriptomics and high-throughput molecular genetics. Lab Chip 16, 1314–1331. doi: 10.1039/c6lc00249h
Shen, X., Li, T., Chen, Z., Xie, X., Zhang, H., Feng, Y., et al. (2019). NIR-light-triggered anticancer strategy for dual-modality imaging-guided combination therapy via a bioinspired hybrid PLGA nanoplatform. Mol. Pharm. 16, 1367–1384. doi: 10.1021/acs.molpharmaceut.8b01321
Shkodra-Pula, B., Grune, C., Traeger, A., Vollrath, A., Schubert, S., Fischer, D., et al. (2019). Effect of surfactant on the size and stability of PLGA nanoparticles encapsulating a protein kinase C inhibitor. Int. J. Pharm. 566, 756–764. doi: 10.1016/j.ijpharm.2019.05.072
Siegel, R. L., Miller, K. D., and Jemal, A. (2019). Cancer statistics, 2019. CA Cancer J. Clin. 69, 7–34. doi: 10.3322/caac.21551
Singh, A. P., Biswas, A., Shukla, A., and Maiti, P. (2019). Targeted therapy in chronic diseases using nanomaterial-based drug delivery vehicles. Signal Transduct. Target. Ther. 4:33. doi: 10.1038/s41392-019-0068-3
Singh, N. K., Singh, S. K., Dash, D., Gonugunta, P., Misra, M., and Maiti, P. (2013). CNT induced β-phase in polylactide: unique crystallization, biodegradation, and biocompatibility. J. Phys. Chem. C 117, 10163–10174. doi: 10.1021/jp4009042
Singh, R. P., and Ramarao, P. (2013). Accumulated polymer degradation products as effector molecules in cytotoxicity of polymeric nanoparticles. Toxicol. Sci. 136, 131–143. doi: 10.1093/toxsci/kft179
Soh, S. H., and Lee, L. Y. (2019). Microencapsulation and nanoencapsulation using supercritical fluid (SCF) techniques. Pharmaceutics 11:21. doi: 10.3390/pharmaceutics11010021
Song, X., Zhao, Y., Wu, W., Bi, Y., Cai, Z., Chen, Q., et al. (2008). PLGA nanoparticles simultaneously loaded with vincristine sulfate and verapamil hydrochloride: systematic study of particle size and drug entrapment efficiency. Int. J. Pharm. 350, 320–329. doi: 10.1016/j.ijpharm.2007.08.034
Streck, S., Clulow, A. J., Nielsen, H. M., Rades, T., Boyd, B. J., McDowell, A., et al. (2019). The distribution of cell-penetrating peptides on polymeric nanoparticles prepared using microfluidics and elucidated with small angle X-ray scattering. J. Colloid Interface Sci. 555, 438–448. doi: 10.1016/j.jcis.2019.08.007
Strohbehn, G., Coman, D., Han, L., Ragheb, R. R. T., Fahmy, T. M., Huttner, A. J., et al. (2015). Imaging the delivery of brain-penetrating PLGA nanoparticles in the brain using magnetic resonance. J. Neurooncol. 121, 441–449. doi: 10.1007/s11060-014-1658-0
Su, Y. H., Chiang, P. C., Cheng, L. J., Lee, C. H., Swami, N. S., and Chou, C. F. (2015). High aspect ratio nanoimprinted grooves of poly(lactic-co-glycolic acid) control the length and direction of retraction fibers during fibroblast cell division. Biointerphases 10:041008. doi: 10.1116/1.4936589
Sun, X., Xu, C., Wu, G., Ye, Q., and Wang, C. J. P. (2017). Poly (lactic-co-glycolic acid): applications and future prospects for periodontal tissue regeneration. Polymers 9:E189. doi: 10.3390/polym9060189
Swider, E., Koshkina, O., Tel, J., Cruz, L. J., de Vries, I. J. M., and Srinivas, M. (2018). Customizing poly(lactic-co-glycolic acid) particles for biomedical applications. Acta Biomater. 73, 38–51. doi: 10.1016/j.actbio.2018.04.006
Trujillo-Nolasco, R. M., Morales-Avila, E., Ocampo-García, B. E., Ferro-Flores, G., Gibbens-Bandala, B. V., Escudero-Castellanos, A., et al. (2019). Preparation and in vitro evaluation of radiolabeled HA-PLGA nanoparticles as novel MTX delivery system for local treatment of rheumatoid arthritis. Mater. Sci. Eng. C Mater. Biol. Appl. 103:109766. doi: 10.1016/j.msec.2019.109766
Tulinska, J., Kazimirova, A., Kuricova, M., Barancokova, M., Liskova, A., Neubauerova, E., et al. (2015). Immunotoxicity and genotoxicity testing of PLGA-PEO nanoparticles in human blood cell model. Nanotoxicology 9(Suppl. 1), 33–43. doi: 10.3109/17435390.2013.816798
Turon, P., del Valle, L., Alemán, C., and Puiggalí, J. (2017). Biodegradable and biocompatible systems based on hydroxyapatite nanoparticles. Appl. Sci. 7:60. doi: 10.3390/app7010060
U.S. National Library of Medicine (2019). Available at: https://clinicaltrials.gov/ct2/results?cond=&term=plga&cntry=&state=&city=&dist= (accessed December 10, 2019). doi: 10.1007/s13233-019-7119-z
Verma, D., Gulati, N., Kaul, S., Mukherjee, S., and Nagaich, U. (2018). Protein based nanostructures for drug delivery. J. Pharm. 2018:9285854. doi: 10.1155/2018/9285854
Vey, E., Rodger, C., Booth, J., Claybourn, M., Miller, A. F., and Saiani, A. (2011). Degradation kinetics of poly (lactic-co-glycolic) acid block copolymer cast films in phosphate buffer solution as revealed by infrared and Raman spectroscopies. Polym. Degrad. Stab. 96, 1882–1889. doi: 10.1016/j.polymdegradstab.2011.07.011
Vhora, I., Patil, S., Bhatt, P., Gandhi, R., Baradia, D., and Misra, A. (2014). Receptor-targeted drug delivery: current perspective and challenges. Ther. Deliv. 5, 1007–1024. doi: 10.4155/tde.14.63
Vllasaliu, D., Fowler, R., and Stolnik, S. (2014). PEGylated nanomedicines: recent progress and remaining concerns. Expert Opin. Drug Deliv. 11, 139–154. doi: 10.1517/17425247.2014.866651
Wang, Y., Li, P., Truong-Dinh Tran, T., Zhang, J., and Kong, L. (2016). Manufacturing techniques and surface engineering of polymer based nanoparticles for targeted drug delivery to cancer. Nanomaterials 6:E26. doi: 10.3390/nano6020026
Wilkosz, N., Łazarski, G., Kovacik, L., Gargas, P., Nowakowska, M., Jamroìz, D., et al. (2018). Molecular insight into drug-loading capacity of PEG–PLGA nanoparticles for itraconazole. J. Phys. Chem. B 122, 7080–7090. doi: 10.1021/acs.jpcb.8b03742
Xi, J., Wang, W., Da, L., Zhang, J., Fan, L., Gao, L., et al. (2018). Au-PLGA hybrid nanoparticles with catalase-mimicking and near-infrared photothermal activities for photoacoustic imaging-guided cancer therapy. ACS Biomater. Sci. Eng. 4, 1083–1091. doi: 10.1021/acsbiomaterials.7b00901
Xu, Q., Hashimoto, M., Dang, T. T., Hoare, T., Kohane, D. S., Whitesides, G. M., et al. (2009). Preparation of monodisperse biodegradable polymer microparticles using a microfluidic flow-focusing device for controlled drug delivery. Small 5, 1575–1581. doi: 10.1002/smll.200801855
Yang, H., Li, J., Patel, S. K., Palmer, K. E., Devlin, B., and Rohan, L. C. (2019). Design of poly (lactic-co-glycolic acid)(PLGA) nanoparticles for vaginal co-delivery of griffithsin and dapivirine and their synergistic effect for HIV prophylaxis. Pharmaceutics 11:E184.
Yang, X. (2019). Design and optimization of crocetin loaded PLGA nanoparticles against diabetic nephropathy via suppression of inflammatory biomarkers: a formulation approach to preclinical study. Drug Deliv. 26, 849–859. doi: 10.1080/10717544.2019.1642417
Yin, Q. (2019). Abstract B142: Activation of Endogenous Anergic Self-Specific CD8+ T-Cells by Polymeric Nanoparticles for Enhanced Cancer Immunotherapy. Philadelphia, PA: AACR.
Yoo, J., Park, C., Yi, G., Lee, D., and Koo, H. (2019). Active targeting strategies using biological ligands for nanoparticle drug delivery systems. Cancers 11:E640. doi: 10.3390/cancers11050640
Zhang, K., Tang, X., Zhang, J., Lu, W., Lin, X., Zhang, Y., et al. (2014). PEG–PLGA copolymers: their structure and structure-influenced drug delivery applications. J. Control. Release 183, 77–86. doi: 10.1016/j.jconrel.2014.03.026
Zhang, M., He, J., Zhang, W., and Liu, J. (2018). Fabrication of TPGS-stabilized liposome-PLGA hybrid nanoparticle via a new modified nanoprecipitation approach: in vitro and in vivo evaluation. Pharm. Res. 35:199. doi: 10.1007/s11095-018-2485-3
Zhang, Z., and Feng, S.-S. (2006). The drug encapsulation efficiency, in vitro drug release, cellular uptake and cytotoxicity of paclitaxel-loaded poly(lactide)–tocopheryl polyethylene glycol succinate nanoparticles. Biomaterials 27, 4025–4033. doi: 10.1016/j.biomaterials.2006.03.006
Keywords: poly(lactide-co-glycolide), drug delivery, biodegradable polymer, nanoparticle preparation, nanomedicine, computational simulation
Citation: Essa D, Kondiah PPD, Choonara YE and Pillay V (2020) The Design of Poly(lactide-co-glycolide) Nanocarriers for Medical Applications. Front. Bioeng. Biotechnol. 8:48. doi: 10.3389/fbioe.2020.00048
Received: 08 October 2019; Accepted: 22 January 2020;
Published: 11 February 2020.
Edited by:
Gianni Ciofani, Italian Institute of Technology (IIT), ItalyReviewed by:
Daniele Di Mascolo, Italian Institute of Technology (IIT), ItalyJyothi U. Menon, The University of Rhode Island, United States
Copyright © 2020 Essa, Kondiah, Choonara and Pillay. This is an open-access article distributed under the terms of the Creative Commons Attribution License (CC BY). The use, distribution or reproduction in other forums is permitted, provided the original author(s) and the copyright owner(s) are credited and that the original publication in this journal is cited, in accordance with accepted academic practice. No use, distribution or reproduction is permitted which does not comply with these terms.
*Correspondence: Viness Pillay, viness.pillay@wits.ac.za