Potential of Chitosan and Its Derivatives for Biomedical Applications in the Central Nervous System
- 1Biotecnología Industrial, CONACYT Centro de Investigación y Asistencia en Tecnología y Diseño del Estado de Jalisco (CIATEJ), Zapopan, Mexico
- 2Unidad de Evaluación Preclínica, Biotecnología Médica y Farmacéutica, CONACYT Centro de Investigación y Asistencia en Tecnología y Diseño del Estado de Jalisco (CIATEJ), Guadalajara, Mexico
- 3Servicio de Neurología, Instituto de Neurociencias, Instituto de Investigación Sanitaria San Carlos (IdISSC), Hospital Clínico San Carlos, Madrid, Spain
It is well known that the central nervous system (CNS) has a limited regenerative capacity and that many therapeutic molecules cannot cross the blood brain barrier (BBB). The use of biomaterials has emerged as an alternative to overcome these limitations. For many years, biomedical applications of chitosan have been studied due to its remarkable biological properties, biocompatibility, and high versatility. Moreover, the interest in this biomaterial for CNS biomedical implementation has increased because of its ability to cross the BBB, mucoadhesiveness, and hydrogel formation capacity. Several chitosan-based biomaterials have been applied with promising results as drug, cell and gene delivery vehicles. Moreover, their capacity to form porous scaffolds and to bear cells and biomolecules has offered a way to achieve neural regeneration. Therefore, this review aims to bring together recent works that highlight the potential of chitosan and its derivatives as adequate biomaterials for applications directed toward the CNS. First, an overview of chitosan and its derivatives is provided with an emphasis on the properties that favor different applications. Second, a compilation of works that employ chitosan-based biomaterials for drug delivery, gene therapy, tissue engineering, and regenerative medicine in the CNS is presented. Finally, the most interesting trends and future perspectives of chitosan and its derivatives applications in the CNS are shown.
Introduction
The central nervous system (CNS) consists of the brain, spinal cord, and retina, which are composed of more than 100 billion individual nerve cells surrounded by bone structures (Payne et al., 2019). The CNS has long been recognized as immune-privileged, attributed to the blood brain barrier (BBB) and the lack of lymphatic vessels within the parenchyma (Engelhardt et al., 2016). Nevertheless, the CNS is unable to generate robust adaptive immune responses (Ransohoff and Brown, 2012). In the absence of immediate or long-term medical care, this situation could lead to permanent damage or death following a severe nervous system injury (Weil et al., 2008). The treatment of CNS diseases gets further complicated by the BBB, which acts as a shield for foreign substances including therapeutic molecules (Huang et al., 2017). Potential treatments against neurodegenerative disorders are considered difficult to implement because of the limited access to the CNS and the aggressiveness of surgical interventions (Tysseling and Kessler, 2017).
It is now known that central axons are capable of regenerating after injury, but their success is highly dependent on their local environment (He and Jin, 2016; Tedeschi and Bradke, 2017). The composition of the microenvironment is defined by the presence of reactive neural cells. Astrocytes and microglia secrete biomolecules as cytokines, chemokines and growth factors in response to insults. These cells display a big heterogeneity (in morphology, function, and gene expression) and have been associated with both beneficial and detrimental regenerative outcomes on CNS injury (Anderson et al., 2014; Karve et al., 2015). It is also known that the adult CNS possesses neural stem cells with the ability to differentiate into neurons and glia. However, these stem cells need a neurogenic microenvironment to achieve migration and differentiation (Gáge and Temple, 2013). Recent advances in biomaterials have encouraged the search to overcome these challenges, either on their own or as vehicles for stem cell, genetic material, or bioactive molecule delivery (Führmann and Shoichet, 2018). These biomaterials can have a natural or synthetic origin. Natural biomaterials often present good biocompatibility, biodegradability, and cell adhesion but can exhibit some disadvantages as poor mechanical properties or trigger an immune response. Their synthetic counterparts are often easier to chemically modify and have low immune responses but may contain toxic substances (Lim and Spector, 2017; Wang Y. et al., 2018). Thus, natural and synthetic biomaterials are frequently used together to exploit the advantages of both, resulting in products with the desired characteristics for each application.
Currently, chitosan is one of the leading natural biomaterials for CNS applications, both in its natural form or as a modified derivative. In biomolecules delivery, it stands out for its penetration enhancement ability and mucoadhesive capacity, which make it a great material for nose-to-brain approaches (Rassu et al., 2016; Yu et al., 2019). In tissue engineering and regenerative medicine, chitosan and its derivatives have shown to promote axonal regeneration, anti-inflammation, and to successfully deliver neurotrophic factors and cells with a consequently functional recovery (Wang Y. et al., 2018). In this way, chitosan-based biomaterials have become increasingly popular to use, alone or in combination with other molecules. This review offers an overview of the physicochemical and biological properties of chitosan and its derivatives. These are useful for different applications, focusing on the delivery of therapeutic molecules and regenerative approaches in the CNS. A literature review was performed through online platforms as PubMed, ScienceDirect, and the National Library of Medicine (clinicaltrials.gov), considering only the works published in the last 5 years. This review aims to show the reader the current trends and limitations of this biopolymer in biomedical applications directed toward the CNS.
Chitosan
Chitosan is a polysaccharide mainly composed of D-glucosamine and, in a lower proportion, N-acetyl-D-glucosamine units randomly β-(1-4)-linked. It can be obtained by deacetylation processes of chitin, which has been recognized as the second most abundant polysaccharide in nature, after cellulose. Even though the main source of chitin is crustacean shell, recent technologies have made possible the obtention of chitin and chitosan from other sources like insects and microorganisms (Peniche et al., 2008; Zargar et al., 2015). Particularly, fungal sources have gained increased attention due to some potential advantages like a homogeneous polymer length, a high degree of deacetylation, and high solubility (Ghormade et al., 2017). In general, there are two types of processes to obtain chitosan: chemical and biological. The chemical method is the most commonly performed at an industrial scale, using strong acid and alkaline treatments (El-Knidri et al., 2018). Biological methods involve microorganisms and enzymes (Arbia et al., 2013), but despite the efforts to achieve scalable enzymatic deacetylation, the high crystallinity of chitin remains the main obstacle (Jaworska and Roberts, 2016).
The source and obtention process of chitosan are important factors to consider according to the desired application. These factors define the final product characteristics. For biomedical applications, its purity, molecular weight (Mw), crystallinity, and deacetylation degree (DD) are of great importance (Nwe et al., 2009). These factors deeply correlate with chitosan’s mechanical and biological properties. Aranaz et al. (2009) reported the relationship between the physicochemical properties of chitosan and its behavior in biomedical applications. This will be further detailed for each biomedical application described in this work.
The increasing interest in chitosan as a biomaterial is due to its natural origin and several biological properties: biocompatibility, non-toxicity, non-allergenicity, and biodegradability, as well as its antifungal, antibacterial, antioxidant, anti-tumor and anti-inflammatory activities. Besides, it has been recognized as an immunoadjuvant, anti-thrombogenic and anti-cholesteremic agent (Younes and Rinaudo, 2015; Kim, 2018). It also possesses high versatility, so it can be used in many physical forms as fibers (and nano-fibers), gels, sponges, beads, films, particles (and nanoparticles), membranes and scaffolds (El-hefian et al., 2011; Rebelo et al., 2017). All these properties make chitosan adequate for many biomedical applications as drug delivery, gene delivery, tissue engineering, and regenerative therapies, among others. However, when it is used on its own, it has poor mechanical properties in wet conditions and low solubility at pH > 7.0. This situation has led to the search of different strategies to overcome chitosan deficiencies by its combination with other materials or through changes in its superficial structure.
It is important to highlight that chitosan is a polycationic polymer, this attribute is conferred by the protonation of D-glucosamine which forms a positively charged moiety (NH3+) at neutral/physiological pH (Muanprasat and Chatsudthipong, 2017). Despite the versatility that this characteristic gives to chitosan, cationic polymers have been reported as neurotoxic and CNS damage inducers (Li and Ju, 2017). The neurotoxicity has been associated with chitosan’s particle size through inflammasome activation (Bueter et al., 2011). However, the evaluation of chitosan’s neurotoxicity is still limited and not detailed. On the other hand, chitosan and its derivatives have also been reported as neuroprotective over different neuronal disorders including Alzheimer’s and Parkinson’s disease, sclerosis, stroke, and injury, among others (Pangestuti and Kim, 2010; Hao C. et al., 2017; Ouyang et al., 2017).
Chitosan Derivatives
The molecular structure of chitosan’s units contains an amino/acetamido group at C-2, a secondary hydroxyl group at C-3 and a primary hydroxyl group at C-6 (Figure 1A). So, the improving modifications that have been developed for this polymer make use of these groups by grafting other molecules. Some of these modifications consist of carboxyalkylation, thiolation, sulfation, phosphorylation, esterification, graft copolymerization, and cross-linking strategies (Mourya and Inamdar, 2008; Muñoz and Zuluaga, 2017). These modifications confer new and unique properties to the obtained products. For example, chitosan has been grafted with heparin to increase its anticoagulant and angiogenic properties, and to increase its affinity for growth factors (Skop et al., 2019). It has also been grafted with laminin-derived peptides to facilitate the attachment of neurons and neurite outgrowth (Kuo and Chiu, 2011). Many molecules can be grafted to improve the application of chitosan to the CNS but have not been evaluated yet. Dicarboxylic acids contain two binding sites that can lead to the crosslinking of chitosan polymeric chains and offer an antioxidant environment. In the same way, hydroxycinnamic acids possesses an important antioxidant activity. For example, the release of ferulic acid into the lesion site of traumatic brain injury (TBI) has shown to effectively protect further secondary injury through the inhibition of neurological oxidative stress (Dong et al., 2015). Nevertheless, these acids have mainly been grafted to chitosan to modify its physicochemical characteristics as solubility, thermal stability, or rheological properties, and have not been widely studied into the CNS (Liu et al., 2017).
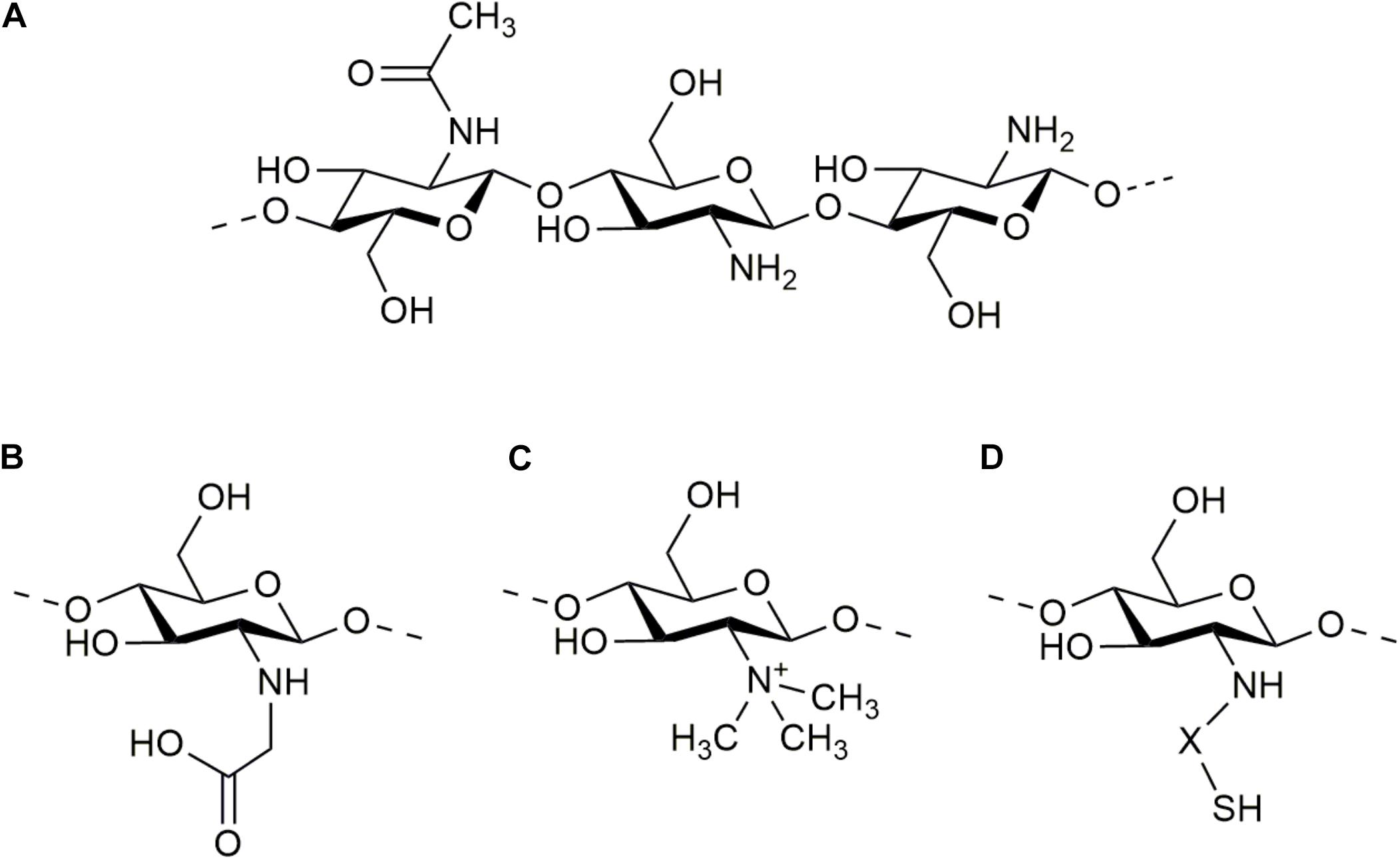
Figure 1. Molecular structure of chitosan (A) and some of its derivatives: N-carboxymethyl chitosan (B), N-trimethyl chitosan (C), and thiolated chitosan (D).
Among the most commonly used modifications of chitosan for biomedical applications directed to CNS are carboxymethylation, N-trimethylation, and thiolation (Figures 1B–D, respectively). These modifications confer new properties to chitosan, as solubility and mucoadhesiveness, converting these biomaterials into proper substrates for biomolecule delivery. Chitosan graft copolymerization is also widely used for CNS application, because it allows to obtain polymers with controlled structures and activities. These are defined by the graft characteristics, including the structure of the molecule, its length, and number (or binding degree). Copolymerization is widely used for the elaboration of tailor-made scaffolds (Mourya and Inamdar, 2008). In addition to the graft attributes, the binding site also plays an important role in the final properties of chitosan-based biomaterials. Ding et al. (2014) produced 6-O-sulfated chitosan and observed a strong effect of the sulfate site in promoting the neural differentiation of mouse embryonic stem cells.
Carboxymethyl Chitosan (CMC)
The introduction of carboxyalkyl groups into the structure of chitosan, as carboxymethyl, has been developed mainly as a strategy for increasing chitosan’s solubility. The reaction occurs either at the C6 hydroxyl group or at the NH2 moiety, giving N-CMC, O-CMC or N,O-CMC as products. These derivatives are amphoteric polymers that produce water-soluble compounds with pH-dependent solubility, water retention properties, biodegradability, biocompatibility, and antioxidant activity (Muñoz and Zuluaga, 2017; Shariatinia, 2018; Xu et al., 2018). Therefore, these amphoteric polymers can be loaded with hydrophobic drugs and display strong bioactivity (Upadhyaya et al., 2014). Moreover, the presence of the functional –OH, –NH2, and –COOH groups in its structure gives the possibility of being easily modified. For example, CMC has been crosslinked with alginate and agarose to be used as a scaffold for stem cell in situ differentiation into functional neurons and supporting neuroglia (Gu et al., 2016). CMC has also been employed to enhance the efficacy of active constituents with poor solubility and bioavailability, and increase brain drug concentration (Ding et al., 2016; Liu et al., 2018). However, Wahba and collaborators developed a galantamine delivery system, against Alzheimer’s disease, attaching galantamine to ceria-containing hydroxyapatite as well as ceria-containing CMC-coated hydroxyapatite nanocomposites. They found that the CMC coating delayed the in vitro release for galantamine and nanoceria (Wahba et al., 2016).
N-Trimethyl Chitosan (TMC)
Methylation consists in the introduction of various alkyl groups at the amino groups of chitosan. The most common product of these reactions is TMC, which is considered one of the strongest mucoadhesive polymers due to its cationic nature (M Ways et al., 2018). That is why it has been used for brain-targeting drug delivery, showing great potential in nose-to-brain applications (Kumar et al., 2013; Meng et al., 2018; Pardeshi and Belgamwar, 2018). Another promising application of TMC is its use to treat brain tumors. For example, Turabee and his team found that the addition of TMC to a pluronic F127 hydrogel increased the biological activity of docetaxel against U87-MG cells. The pluronic F127-TMC/docetaxel hydrogel was evaluated in vivo employing BALB/c nude mice and showed sustained release of docetaxel with tumor suppression (Turabee et al., 2019). Similarly, Sedeky et al. (2018) observed a significant improvement in cytotoxicity of Piperine-loaded TMC nanoparticles on human brain cancer cell line Hs683.
Thiolated Chitosan
Thiolation is the reaction of primary amino groups of chitosan with coupling reagents that contain thiol groups (thioglycolic acid, 2-iminothiolane, cysteine, and thiobutylamidine). This product has high permeation, mucoadhesion, higher solubility at physiological pH and displays in situ gelling properties (Sreenivas and Pai, 2008). These properties present thiolated chitosan as a good substrate for drug delivery to the brain, mainly used as nanoparticles (Patel et al., 2012, 2013; Singh et al., 2016; Sunena et al., 2019). In this way, Patel et al. (2013) studied brain uptake of cyclobenzaprine HCl-loaded thiolated chitosan nanoparticles on Swiss albino mice after intranasal administration and observed that thiolation of chitosan reduced trans-mucosal toxicity and enhanced the bioavailability. The in situ gelling ability makes thiolated chitosan suitable not only for nose-to-brain applications but also for the elaboration of scaffolds. However, it has not been widely used for neural tissue engineering. For this purpose, methacrylamide chitosan has been thiolated, giving as products porous and biodegradable scaffolds that are suitable for cell growth and neural stem cell differentiation in 3D (Yu et al., 2007; Leipzig et al., 2011).
Grafting Copolymerization of Chitosan
Frequently, chitosan is grafted with other polymers to reach copolymerization. The graft polymer is selected by its chemical, mechanical or biological properties and the copolymerization results in a chitosan-based product with added characteristics. For example, polyethylene glycol (PEG)-grafted chitosan derivatives have increased solubility over a wide range of pH and have shown enhanced mucoadhesion (Bhavsar et al., 2017). In this way, 2-O-PEGylated chitosan has been used for the elaboration of siRNA-carrying nanoparticles that target the brain to treat neurodegenerative diseases (Malhotra et al., 2013a). Other polymers that have been grafted to chitosan for CNS application are gelatin (Gao S. et al., 2014), poly lactic-co-glycolic acid (PLGA) (Tong et al., 2017), poly (3,4 ethylenedioxythiophene) (PEDOT) (Wang S. et al., 2018), alginate, and agarose (Gu et al., 2016), among others.
Chitosan-Based Delivery Systems to CNS
For many years, the increasing incidence of neurodegenerative disorders and the lack of functional treatments have encouraged the search for new therapeutic approaches to counteract CNS diseases. The administration routes directed to the CNS mainly consist of systemic administration, nose-to-brain, and direct injection into the brain parenchyma or cerebrospinal fluid. However, it remains challenging to find effective treatments. One of the main reasons for this is the BBB, which separates the brain from the blood supply and distinguishes between the molecules that can and cannot cross through itself. The BBB allows the entry of nutrients and hormones but restricts other external materials. Therefore, most of the therapeutic molecules are unable to cross and access to the CNS from the bloodstream, following systemic administration (Chatterjee et al., 2019). This situation has led to the development of different strategies for aiding therapeutic molecules to permeate the BBB and to get access to the brain. Dong elaborated a review article providing an overview of the current strategies to enhance drug delivery to the brain (Dong, 2018). According to it, permeability enhancers, active transporters, viral vectors, nanoparticles, and exosomes have been proposed for aiding therapeutic molecules to cross the BBB after systemic administration (Choi et al., 2008; Dong, 2018; He et al., 2018). For direct administration into the brain parenchyma or cerebrospinal fluid, implantable devices have emerged as effective delivery systems that avoid systemic concerns (Stewart et al., 2018). However, most of these strategies have the disadvantage of bearing low drug concentrations or being invasive. Therefore, the use of carriers/vehicles and non-conventional administration routes have emerged as a new approach for facilitating the delivery of therapeutic molecules to the brain (Upadhyay, 2014; Bonferoni et al., 2019). In this way, nose-to-brain administration has also made use of viral vectors, exosomes, and nanoparticles to achieve less invasive and more effective treatments (Jain, 2012; Belur et al., 2017; Dong, 2018; Li et al., 2019). This information is summarized in Figure 2. Nevertheless, the use of biocompatible carriers is encouraged to prevent unwanted effects and achieve high and sustained local drug delivery (Chen et al., 2019).
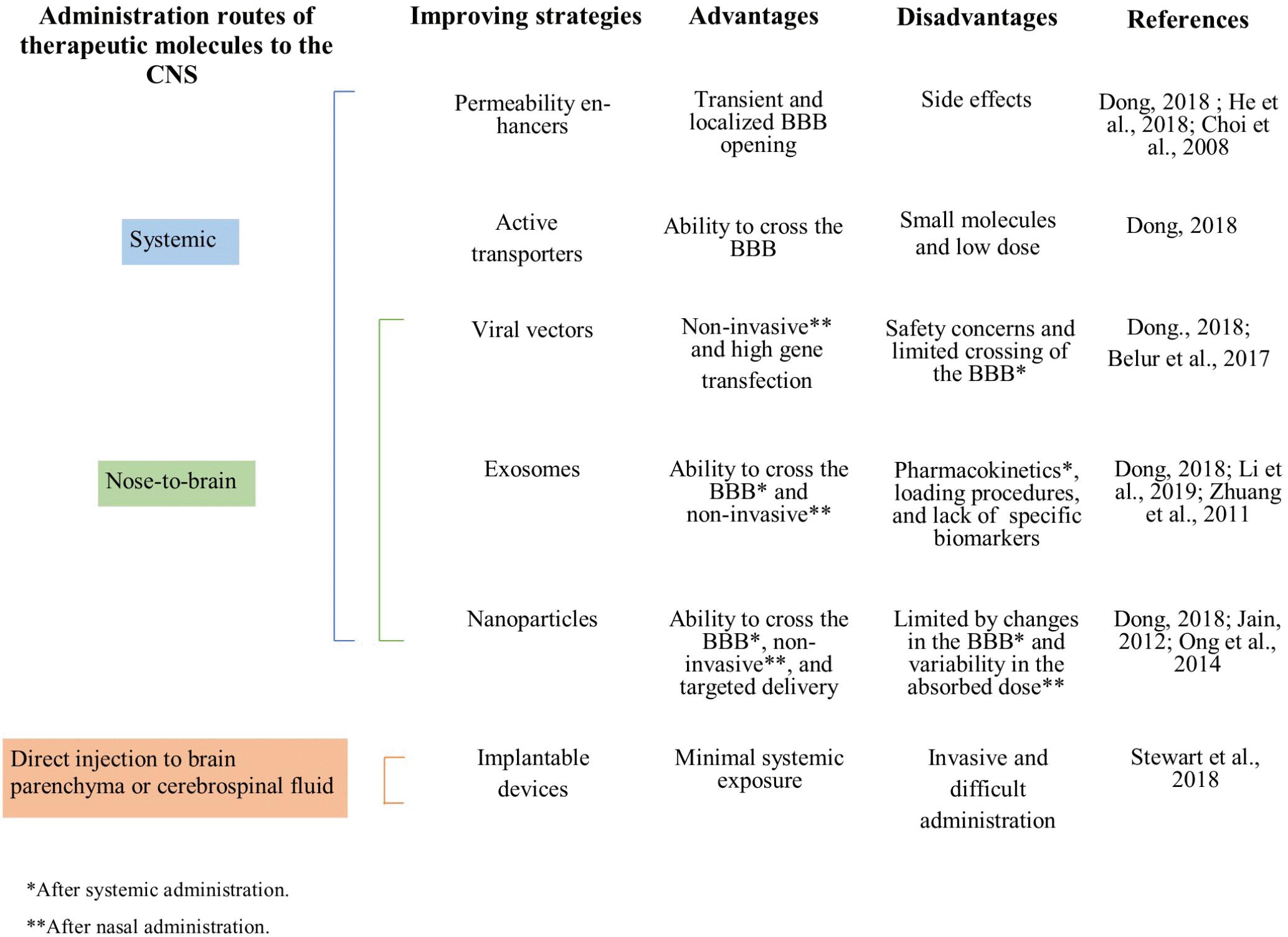
Figure 2. Advantages and disadvantages of the current strategies to enhance therapeutic molecules delivery to the CNS.
Drug Delivery to CNS
Chitosan possesses a lot of advantages as a brain-targeted drug carrier. Coupled with its capability to penetrate the BBB, it also can control release, adhere to mucus, and open tight junctions of the nasal membrane. These abilities favor its application in nose-to-brain drug delivery strategies (Mohammed et al., 2017; Yu et al., 2019).
Another advantage of chitosan is its versatility, it can be used for drug delivery purposes as microspheres, capsules, hydrogels, conjugates, nanoparticles, films, beads, or tablets (Ali and Ahmed, 2018). However, nanoparticles have gained special attention in this field due to their capability to protect drugs from degradation during administration (Tzeyung et al., 2019). Chitosan nanoparticles have shown to enhance the brain targeting efficiency and, therefore, to improve the therapeutic potential of drugs (Md et al., 2013; Nagpal et al., 2013). Chitosan has also been used as a nanoparticle coating, to grant drug-loaded nanoparticles with a net positive charge and facilitate cellular internalization (Varan and Bilensoy, 2017).
For drug delivery, it has been reported that the use of low Mw chitosan increases the encapsulation efficiency (Yang and Hon, 2009), reduces cytotoxicity and increases the degradation rate of nanoparticles, properties that have been also associated with higher DDs (Sarvaiya and Agrawal, 2015). On the other hand, the penetration in the mucin layer and the mucoadhesion strength of chitosan increase when the Mw is higher (Rassu et al., 2016). It is worth mentioning that these properties are influenced when chitosan is functionalized, and they depend on the added molecules. For example, Kuo and collaborators, recently developed chitosan-PLGA nanoparticles grafted with anti-aldehyde dehydrogenase and sialic acid for brain tumor-targeted delivery of curcumin (Kuo et al., 2019). They promoted the BBB permeation through N-acetylglucosamine. However, the targeting of the delivery system was improved with the addition of sialic acid and the anti-aldehyde dehydrogenase by directing it to the membrane of glioblastoma cells and brain cancer stem cells.
The use of chitosan and its derivatives for drug delivery to the brain has been employed for developing treatments against many neurological disorders, mainly for Parkinson’s and Alzheimer’s diseases. Other studies have been guided to treat conditions like depression, schizophrenia, migraine, brain tumor, general anxiety disorder, epilepsy, pain, viral and bacterial infections, and so on (Table 1). However, at the time of writing this article, only one of these studies has been taken to clinical trials (Ruppen, 2015). In that clinical research, a nasal ketamine spray with chitosan was evaluated in comparison with oral morphine to treat pain in cancer outpatients but no results have been reported yet.
Gene Therapy
Gene therapy has been set as a form of drug delivery, where cellular machinery is modulated to produce a therapeutic effect (Blau and Springer, 1995). As in drug delivery systems, some of the most remarkable difficulties to direct this technology toward the CNS consist of low BBB permeability, brain heterogeneity, invasive or inefficient routes of administration, and dosing (Joshi et al., 2017). Different types of vectors have been used to overcome these limitations, being the viral ones the most employed (Choudhury et al., 2017). Nevertheless, human infections and immune response caused by viral vectors have led to the search for safer vectors. The aforementioned polycationic property of chitosan confers the polymer the capacity to establish strong electrostatic interactions with negatively charged molecules, like DNA and RNA.
To this day many chitosan-based systems for gene delivery have been employed. Mao et al. (2010) reviewed the formulation factors that affect siRNA and DNA delivery and transfection efficiency. They highlighted that the transfection efficiency depends on many parameters and concluded that intermediate values of Mw and DD of chitosan form complexes of intermediate stability and efficient transfection. Chitosan derivatives have also been employed for this purpose. Specifically, for therapeutic gene delivery to the brain, PEGylation has shown to enhance biocompatibility and stability of siRNA loaded complexes (Gao Y. et al., 2014). Moreover, PEG plays the role of a linker between chitosan and targeting peptides, which form complexes with nucleic acid and enhance the cellular uptake of chitosan nanoparticles (Malhotra et al., 2013b; Jiang et al., 2014).
Despite the versatility of chitosan, nanoparticles have been the preferred candidates to counter different neurological disorders. Among these disorders are glioblastoma (Malmo et al., 2013; Danhier et al., 2015; Xu et al., 2015; Van Woensel et al., 2016, 2017), medulloblastoma (Kievit et al., 2015), Parkinson’s disease (Peng et al., 2014), Alzheimer’s disease (Gao Y. et al., 2014; Rassu et al., 2017), and multiple sclerosis (Youssef et al., 2019). Even viral infections, like HIV-infected brain, have been a target for this therapeutic strategy (Gu et al., 2017). Recently, the search for less invasive strategies has guided the development of novel formulations for nose-to-brain gene delivery. For example, Rassu et al. (2017), made chitosan-coated solid lipid nanoparticles carrying BACE1 siRNA for intranasal application against Alzheimer’s disease. Similarly, Van Woensel et al. (2017) formulated siRNA targeting Gal-1 loaded chitosan nanoparticles for intranasal delivery in mice, obtaining remarkable changes in the tumor micro-environment of glioblastoma multiforme. Moreover, Sánchez-Ramos and his collaborators designed a chitosan-Mangafodipir intranasal nanocarrier system for the delivery of siRNA or dsDNA. They employed anti-eGFP siRNA and reported the effectiveness of the nanoparticles for reducing GFP mRNA expression in Tg GFP+ mice along different brain zones (Sanchez-Ramos et al., 2018). These advances suggest an imminent overcoming of the difficulties that limit the CNS-directed gene therapy.
Chitosan-Based Materials for Tissue Engineering and Regenerative Medicine in CNS
The design of different chitosan-based biomaterials for tissue engineering and regenerative medicine in CNS aims to facilitate neural cell adhesion, proliferation, and differentiation. These biomaterials can be used as scaffolds to mimic the natural extracellular matrix and microenvironment for better in vitro approaches or tissue replacement. Thus, these polymeric materials can be useful to overcome the limitations of cell therapy. For in vitro applications, the conformation of the biomaterials must present good biocompatibility and porous structures that favor 3D cell growth. Regenerative medicine requires biomaterials that offer mechanical support for growing neurites. Biological support is also required to lead the processes to tissue restoration through stem cell differentiation and integration into the surrounding healthy tissue (Boni et al., 2018). Moreover, it is important to cause a minimal inflammatory response when implanted. In this way, properties as biocompatibility, biodegradability, mechanical strength, architecture, and cell-adhesion capacity become crucial for biomaterial success.
Gnavi et al. (2013) made a review article detailing the characteristics of chitosan-based scaffolds for nervous system regeneration. They highlighted that the physicochemical properties of chitosan (and modified chitosan) can be easily manipulated to design specific structural features for the scaffolds. According to the required structure and properties for tissue restoration, the biomaterial scaffolds for CNS regeneration can be classified into two types: hydrogels and biodegradable scaffolds (Wang Y. et al., 2018). Chitosan hydrogels can be obtained by physical or chemical crosslinking. The physical associations, like ionic bonding and hydrogen bonds, provide unstable structures while the chemical associations formed by covalent bonds give place to uniform properties. For faster hydrogel biodegradation, it is recommended the use of labile bonds that can be broken under physiological conditions (Pellá et al., 2018). On the other hand, in situ gelling can be achieved by physical interactions, providing the advantages of cell delivery without previous geometrical shape preparation of hydrogels and with a less invasive implantation process (Shariatinia and Jalali, 2018). For application in the CNS, hydrogels have been obtained from chitosan (Chedly et al., 2017), its derivatives as CMC (Xu et al., 2018) and chitosan lactate (Nawrotek et al., 2017), and mixtures with other polymers like gelatin (Gao S. et al., 2014). Biodegradable scaffolds are mainly structured by freeze-drying but can be also obtained by electrospinning, solvent evaporation, supercritical carbon dioxide, and 3D printing (Croisier and Jérôme, 2013; Wang Y. et al., 2018; Sun et al., 2019). For porous scaffolds, many chitosan-blends have been made by combining different biodegradable materials, like gelatin (Wang et al., 2017), collagen (Yan et al., 2019), and PEDOT (Wang S. et al., 2018), among others. One of the principal advantages of these scaffolds resides in having stabilized porous structures that can be designed with different size ranges and mechanical properties (Xu et al., 2017).
For non-CNS tissue engineering applications, Mw and DD have been associated with biodegradability and viscosity. Higher Mw gives delayed biodegradation when implanted, and more viscous biomaterials. DD values between 65 and 82% give faster biodegradation (Rodriguez-Vazquez et al., 2015). It is worth mentioning that there is huge variability in the main chemical properties of the starting chitosans used in the reviewed studies, including Mw from 1 (Yao et al., 2018) to 550 kDa (Chedly et al., 2017) and values of 75–95% of DD (Feng et al., 2014; Tseng et al., 2015). Moreover, none of the reviewed CNS application studies in tissue engineering and regenerative medicine evaluates different Mw or DD in their starting materials. Many of the studies do not detail these two important chemical characteristics of their starting chitosan. Otherwise, the main variation in these works consists of using different polymeric blends and ratios as starting materials.
Implanting chitosan-based biomaterials in the CNS provides a way to its poor regenerative capacity through the reconstruction of lost tissue and reconnection of neuronal processes. Although, the incorporation of stem cells and biomolecules into these scaffolds has emerged as an additional strategy to enhance regenerative therapies (Ricks et al., 2014). In this way, biomaterials assist cell therapy as delivery vehicles that promote cell survival and engraftment. Another advantage of the combination of both research areas is that the implanted cells can be separated from the host damaged tissue. Thereby, biomaterials provide an independent microenvironment for cell differentiation and proliferation, which does not occur in the natural response to damage (Wang Y. et al., 2018).
Beyond the aforementioned physicochemical properties of chitosan that make it a suitable biopolymer to make biodegradable scaffolds and hydrogels, chitosan has neuroprotective properties. Anti-neuroinflammatory activity, suppression of β-amyloid and acetylcholinesterase formation, and anti-apoptosis effects have been reported (Pangestuti and Kim, 2010; Hao C. et al., 2017). These neuroprotective effects promote an adequate microenvironment for cell proliferation in some CNS damage processes.
Chitosan-Based Scaffolding
Over the past few years, many strategies for increasing cell adhesion, differentiation and viability on chitosan-based scaffolds have been implemented (Table 2). Different mixtures of biopolymers with chitosan have been employed for modulating the micro-structure of the scaffolds and their properties. For example, collagen copolymerization has proven to promote cell affinity through its arginine-glycine-aspartic acid sequence which is recognized by transmembrane integrins (Kuo and Yeh, 2011). In the same way, polylactic acid copolymerization gives rise to materials with better mechanical properties and it has been cataloged as a perfect synthetic polymer to elaborate composite materials with chitosan (Ebrahimi-Barough et al., 2015; Hoveizi et al., 2015). On the other hand, Abasi et al. (2019) recently developed bionanocomposites of polyaniline-chloride/chitosan and observed that physical factors of the scaffolds (as electrical conductivity and morphology) have a bigger influence in cell-substrate interactions than molecular affinity. Also, Sung et al. (2015) studied the behavior of Neuro-2a cells over flat, micro-, and nano-textured chitosan substrates, and found that cellular adhesion increases over flat chitosan surfaces. Given that the design of the internal structure and surface of the scaffolds is determinant for cell adhesion and proliferation, Sun and collaborators printed a collagen-chitosan 3D scaffold with a specific structure. They observed nerve fibers regeneration and functional recovery after its implantation in rats with spinal cord injury (SCI), showing enhanced therapeutic effects compared with the non-3D-printed material (Sun et al., 2019).
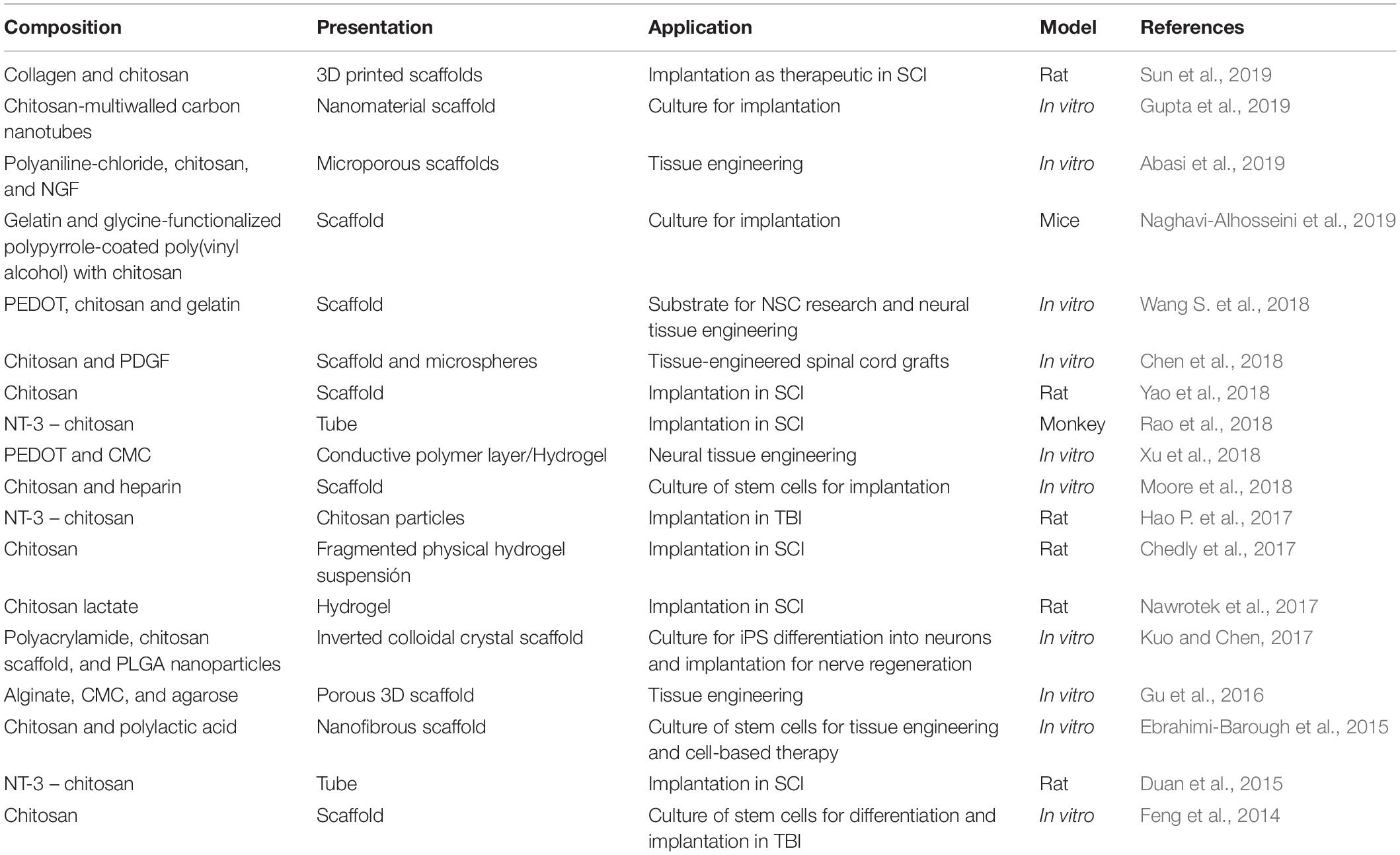
Table 2. Chitosan-based biomaterials for implantation in CNS or neural cell culture reported in the last 5 years.
The addition of neurotrophic factors into chitosan scaffolds or microspheres, like nerve growth factor (NGF), neurotrophin-3 (NT-3), or fibroblast growth factor-2 (FGF-2), has shown to enhance neurogenesis, neural differentiation, and cell survival (Yi et al., 2011; Skop et al., 2013; Duan et al., 2015; Hao P. et al., 2017). Rao et al. (2018) elaborated NT-3 – chitosan tubes that promoted neuroprotection, neurogenesis, revascularization, and antiinflammation on SCI conditions. After implantation, they observed robust neural regeneration with motor and sensory functional recovery in rhesus monkeys (Rao et al., 2018).
The implantation of chitosan hydrogels constitutes an interesting possibility for CNS restoration. Chitosan hydrogels have proved to provide a suitable micro-environment for axons regrowth and increase the survival rate of damaged neurons in different animal models. These hydrogels have shown remarkable potential in CNS repair, even in the absence of added trophic factors or without a detailed design of its structure (Tseng et al., 2015; Nawrotek et al., 2017). Chedly et al. (2017) elaborated a fragmented physical hydrogel suspension employing unmodified chitosan for its implantation in rat SCI (immediately after the injury). They observed axonal regrowth, modulated inflammatory response, and long-lasting locomotor function recovery (Chedly et al., 2017). Even though more studies employing chitosan hydrogels are required to define their therapeutic potential in different damage models or degenerative diseases, these results provide a tool for future evaluations in combined repair strategies.
Chitosan-Based Materials and Cell Therapy to the CNS
Spinal cord and brain injury, as well as neurodegenerative diseases, are conducted by different biological processes and cause diverse symptoms, though all of them result in neuronal degeneration and cell death. Cell therapies for CNS have attained clinical research in different pathological conditions like stroke, TBI, amyotrophic lateral sclerosis, and Parkinson’s disease, showing their contribution to mitigating damage (Watanabe, 2018). However, within damage processes occur extracellular matrix, neuronal, and glial cell loss. This tissue loss results in a hostile environment for transplanted cells and causes deficient engraftment with poor cell viability (Boisserand et al., 2016). In recent years, the incorporation of different biomaterials to cell therapy in CNS has shown to promote cell survival, integration, and differentiation (Führmann and Shoichet, 2018). In this way, chitosan-based biomaterials have been employed in combination with stem/precursor cells to build a way to neuro-regeneration (Table 3). The function of these biopolymeric structures is not only to serve as delivery vehicles and cell physical supports, besides they must regulate the biological microenvironment to guide axonal growth and favor the integration of the healthy tissue to the lesion zone (Boni et al., 2018). Some of the most studied cells for CNS repair are the mesenchymal stem cells (MSC), bone marrow mesenchymal stem cells (BM-MSC), neural stem cells (NSC), and neural precursor cells (NPC).
A study reported by Sugai et al. (2015) showed that modified-chitosan microfibers promote neural stem/progenitor cell proliferation in vitro but not cell survival after transplantation, contrary to collagen-based microfibers. The authors proposed that the stiffness of chitosan precluded the colonization of other cells, like vascular epithelial cells (Sugai et al., 2015). The stiffness of the scaffolds should be in the range of 0.1–1 kPa for mimicking soft tissue like the brain. It is well known that stiffness has a notorious influence on stem cell response and function (Liang et al., 2019). Moreover, it has been proved that cell size affects the cellular response to matrix stiffness in 3D cultures, especially large cells as many of the human stem cells (Bao et al., 2019). So, in these cell-scaffold strategies, it is very important to consider the starting material and cell population. The main advantage of using chitosan as the starting material for this purpose is its high versatility. Thus, stiffness and other important characteristics that affect cell behavior, as viscoelasticity, porosity, and topography can be easily modulated.
Besides its versatility, chitosan and its derivatives have shown to be a superior substrate for cell therapy in comparison with other polymers. Scanga et al. (2010) showed the capability of adult murine NPC to proliferate and differentiate into the three neural cell types when they were cultured over chitosan hydrogel films. On the contrary, NPC differentiation was not observed over poly(oligoethylene oxide dimethacrylate-co-2-amino ethyl methacrylate) or its blend with poly(vinyl alcohol), neither over poly(glycerol dimethacrylate-co-2-amino ethyl methacrylate) (Scanga et al., 2010). Kim et al. (2016) observed a better functional improvement in rats with SCI after MSC transplantation over chitosan scaffolds in contrast to PLGA scaffolds. Moreover, they studied intralesional injection of the same cells and compared it with scaffold-based transplantation in rats. They found a higher MSC engraftment when the scaffolds were employed (Kim et al., 2016). The culture of rat PC12 line and human neural stem cells over chitosan has also shown better results in comparison with cellulose acetate or polyethersulfone derived electrospun nanofibers (Du et al., 2014).
The incorporation of trophic factors as FGF-2, NGF, PDGF, and bGFG has also shown to enhance stemness of neural stem cells and favor its differentiation and proliferation when implanted with chitosan scaffolds or microspheres (Hoveizi et al., 2015; Skop et al., 2016; Moore et al., 2018). Recently, Skop and his collaborators designed a cell-scaffold strategy employing a radial glial neural precursor cell line that conditionally secreted insulin-like growth factor I. This cell line was attached to a chitosan-based microsphere scaffold and injected into the lesion cavity of adult rats with TBI. They observed differentiation toward the three neural cell types (neurons, astrocytes, and oligodendrocytes) and improved capacity for neuronal differentiation. These obtained effects led to the recovery of the somatosensory function. However, the presence of insulin-like growth factor I was not associated with a higher cell retention rate or improved cell replacement. So, the way it improves functional recovery must be elucidated in future studies (Skop et al., 2019).
Conclusion
One of the main factors that preclude the application of chitosan is its poor solubility and poor mechanical properties. However, this review summarizes the different strategies that have been used to overcome these conditions. The obtention of carboxymethylated, trimethylated, thiolated and other chitosan-grafted derivatives has increased the potential of this biopolymer, allowing for the elaboration of biomaterials that help to counteract neurological disorders. Nevertheless, there is still a lack of knowledge about the relation of the molecular changes and the acquired biological properties of these derivatives, especially within a heterogeneous landscape as the CNS. In this way, the authors suggest continuing with the exploration of grafting molecules that improve the biological properties of modified chitosan. For example, hydroxycinnamic acids have been studied by some of the authors and resulted in interesting bioconjugates for CNS applications.
Chitosan-based biomaterials have shown favorable projection. For drug and gene delivery purposes, chitosan nanoparticles have shown to be the most promising strategy due to its mucoadhesion and increased permeability that suits nose-to-brain applications. In this way, the degradation of the therapeutic molecules is reduced, and it also opens the door to less invasive and more effective administration routes. In order to achieve neuro-regeneration, the transplant of stem cells into chitosan-based vehicles gives an optimistic outlook. Strategies like the addition of neurotrophic factors or even the genetic modification of stem cells have successfully increased differentiation and viability. The observed functional recovery in different chitosan-based regenerative therapies encourages the exploration of new cell-scaffold-biomolecule configurations. Despite the wide variety of designed compositions and functions, many factors are implied in cell behavior and, until now, there is not a recipe to elaborate adequate chitosan-based biomaterials that fulfill all the requirements for neuro-repair and to transfer these strategies to clinical trials. Even so, the in vitro and in vivo studies carried out around the world are helping to understand the biological processes involved in neuro-repair and the effect of chitosan biomaterials on them. The authors suggest that future works with chitosan targeting the CNS must intermix the already suggested strategies and propose novel interdisciplinary approaches to attain translation into the clinical level.
Author Contributions
DO-H, AC-A, JM-G, UG-P, and JM-D equally contributed to the literature search, writing and correcting of this review manuscript.
Funding
This work was financed by the project Atención a Problemas Nacionales 2017-01-6267, CONACYT.
Conflict of Interest
The authors declare that the research was conducted in the absence of any commercial or financial relationships that could be construed as a potential conflict of interest.
Acknowledgments
The authors would like to thank Alexis Olimon and Arturo Calderón for their review of the English transcript and CONACYT for scholarship #634170.
References
Abasi, S., Aggas, J. R., and Guiseppi-Elie, A. (2019). Physiochemical and morphological dependent growth of NIH/3T3 and PC-12 on polyaniline-chloride/chitosan bionanocomposites. Mater. Sci. Eng. C Mater. Biol. Appl. 99, 1304–1312. doi: 10.1016/j.msec.2019.02.018
Abdou, E. M., Kandil, S. M., and Miniawy, H. (2017). Brain targeting efficiency of antimigrain drug loaded mucoadhesive intranasal nanoemulsion. Int. J. Pharm. 529, 667–677. doi: 10.1016/j.ijpharm.2017.07.030
Ali, A., and Ahmed, S. (2018). A review on chitosan and its nanocomposites in drug delivery. Int. J. Biol. Macromol. 109, 273–286. doi: 10.1016/j.ijbiomac.2017.12.078
Ana, R., Mendes, M., Sousa, J., Pais, A., Falcao, A., Fortuna, A., et al. (2019). Rethinking carbamazepine oral delivery using polymer-lipid hybrid nanoparticles. Int. J. Pharm. 554, 352–365. doi: 10.1016/j.ijpharm.2018.11.028
Anderson, M. A., Ao, Y., and Sofroniew, M. V. (2014). Heterogeneity of reactive astrocytes. Neurosci. Lett. 565, 23–29. doi: 10.1016/j.neulet.2013.12.030
Aranaz, I., Mengíbar, M., Harris, R., Paños, I., Miralles, B., Acosta, N., et al. (2009). Functional characterization of chitin and chitosan. Curr. Chem. Biol. 3, 203–230.
Arbia, W., Arbia, L., Adour, L., and Amrane, A. (2013). Chitin extraction from crustacean shells using biological methods – a review. Food Technol. Biotechnol. 51, 12–25.
Bao, M., Xie, J., Katoele, N., Hu, X., Wang, B., Piruska, A., et al. (2019). Cellular volume and matrix stiffness direct stem cell behavior in a 3D microniche. ACS Appl. Mater. Interfaces 11, 1754–1759. doi: 10.1021/acsami.8b19396
Bari, N. K., Fazil, M., Hassan, M. Q., Haider, M. R., Gaba, B., Narang, J. K., et al. (2015). Brain delivery of buspirone hydrochloride chitosan nanoparticles for the treatment of general anxiety disorder. Int. J. Biol. Macromol. 81, 49–59. doi: 10.1016/j.ijbiomac.2015.07.041
Belur, L. R., Temme, A., Podetz-Pedersen, K. M., Riedl, M., Vulchanova, L., Robinson, N., et al. (2017). Intranasal Adeno-associated virus mediated gene delivery and expression of human iduronidase in the central nervous system: a noninvasive and effective approach for prevention of neurologic disease in mucopolysaccharidosis type I. Hum. Gene Ther. 28, 576–587. doi: 10.1089/hum.2017.187
Bhavna, Md, S., Ali, M., Ali, R., Bhatnagar, A., Baboota, S., et al. (2014). Donepezil nanosuspension intended for nose to brain targeting: in vitro and in vivo safety evaluation. Int. J. Biol. Macromol. 67, 418–425. doi: 10.1016/j.ijbiomac.2014.03.022
Bhavsar, C., Momin, M., Gharat, S., and Omri, A. (2017). Functionalized and graft copolymers of chitosan, and its pharmaceutical applications. Expert Opin. Drug Deliv. 14, 1189–1204. doi: 10.1080/17425247.2017.1241230
Blau, H., and Springer, M. (1995). Gene therapy — A novel form of drug delivery. N. Engl. J. Med. 333, 1204–1207. doi: 10.1056/NEJM199511023331808
Boisserand, L. S., Kodama, T., Papassin, J., Auzely, R., Moisan, A., Rome, C., et al. (2016). Biomaterial applications in cell-based therapy in experimental stroke. Stem Cells Int. 2016:6810562. doi: 10.1155/2016/6810562
Bonferoni, M. C., Rossi, S., Sandri, G., Ferrari, F., Gavini, E., Rassu, G., et al. (2019). Nanoemulsions for “Nose-to-Brain” drug delivery. Pharmaceutics 11:84. doi: 10.3390/pharmaceutics11020084
Boni, R., Ali, A., Shavandi, A., and Clarkson, A. N. (2018). Current and novel polymeric biomaterials for neural tissue engineering. J. Biomed. Sci. 25:90. doi: 10.1186/s12929-018-0491-8
Bshara, H., Osman, R., Mansour, S., and El-Shamy Ael, H. (2014). Chitosan and cyclodextrin in intranasal microemulsion for improved brain buspirone hydrochloride pharmacokinetics in rats. Carbohydr. Polym. 99, 297–305. doi: 10.1016/j.carbpol.2013.08.027
Bueter, C. L., Lee, C. K., Rathinam, V. A., Healy, G. J., Taron, C. H., Specht, C. A., et al. (2011). Chitosan but not chitin activates the inflammasome by a mechanism dependent upon phagocytosis. J. Biol. Chem. 286, 35447–35455. doi: 10.1074/jbc.M111.274936
Chatterjee, B., Gorain, B., Mohananaidu, K., Sengupta, P., Mandal, U. K., and Choudhury, H. (2019). Targeted drug delivery to the brain via intranasal nanoemulsion: available proof of concept and existing challenges. Int. J. Pharm. 565, 258–268. doi: 10.1016/j.ijpharm.2019.05.032
Chedly, J., Soares, S., Montembault, A., von Boxberg, Y., Veron-Ravaille, M., Mouffle, C., et al. (2017). Physical chitosan microhydrogels as scaffolds for spinal cord injury restoration and axon regeneration. Biomaterials 138, 91–107. doi: 10.1016/j.biomaterials.2017.05.024
Chen, X., Xu, M. L., Wang, C. N., Zhang, L. Z., Zhao, Y. H., Zhu, C. L., et al. (2018). A partition-type tubular scaffold loaded with PDGF-releasing microspheres for spinal cord repair facilitates the directional migration and growth of cells. Neural Regen. Res. 13, 1231–1240. doi: 10.4103/1673-5374.235061
Chen, Z., Liu, Z., Zhang, M., Huang, W., Li, Z., Wang, S., et al. (2019). EPHA2 blockade reverses acquired resistance to afatinib induced by EPHA2-mediated MAPK pathway activation in gastric cancer cells and avatar mice. Int. J. Cancer 145, 2440–2449. doi: 10.1002/ijc.32313
Choi, J., Wang, S., Brown, T. R., Small, S. A., Duff, K. E. K., and Konofagou, E. E. (2008). Noninvasive and Transient Blood-Brain Barrier Opening in the Hippocampus of Alzheimer’s Double Transgenic Mice Using Focused Ultrasound. Ultrason Imaging 30, 189–200. doi: 10.1177/016173460803000304
Choudhury, S. R., Hudry, E., Maguire, C. A., Sena-Esteves, M., Breakefield, X. O., and Grandi, P. (2017). Viral vectors for therapy of neurologic diseases. Neuropharmacology 120, 63–80. doi: 10.1016/j.neuropharm.2016.02.013
Croisier, F., and Jérôme, C. (2013). Chitosan-based biomaterials for tissue engineering. Eur. Polym. J. 49, 780–792. doi: 10.1016/j.eurpolymj.2012.12.009
Danhier, F., Messaoudi, K., Lemaire, L., Benoit, J. P., and Lagarce, F. (2015). Combined anti-Galectin-1 and anti-EGFR siRNA-loaded chitosan-lipid nanocapsules decrease temozolomide resistance in glioblastoma: in vivo evaluation. Int. J. Pharm. 481, 154–161. doi: 10.1016/j.ijpharm.2015.01.051
Ding, K., Wang, Y., Wang, H., Yuan, L., Tan, M., Shi, X., et al. (2014). 6-O-sulfated chitosan promoting the neural differentiation of mouse embryonic stem cells. ACS Appl. Mater. Interfaces 6, 20043–20050. doi: 10.1021/am505628g
Ding, Y., Qiao, Y., Wang, M., Zhang, H., Li, L., Zhang, Y., et al. (2016). Enhanced neuroprotection of Acetyl-11-Keto-beta-Boswellic Acid (AKBA)-Loaded O-Carboxymethyl chitosan nanoparticles through antioxidant and anti-inflammatory pathways. Mol. Neurobiol. 53, 3842–3853. doi: 10.1007/s12035-015-9333-9
Dong, G. C., Kuan, C. Y., Subramaniam, S., Zhao, J. Y., Sivasubramaniam, S., Chang, H. Y., et al. (2015). A potent inhibition of oxidative stress induced gene expression in neural cells by sustained ferulic acid release from chitosan based hydrogel. Mater. Sci. Eng. C Mater. Biol. Appl. 49, 691–699. doi: 10.1016/j.msec.2015.01.030
Dong, X. (2018). Current strategies for brain drug delivery. Theranostics 8, 1481–1493. doi: 10.7150/thno.21254
Du, J., Tan, E., Kim, H. J., Zhang, A., Bhattacharya, R., and Yarema, K. J. (2014). Comparative evaluation of chitosan, cellulose acetate, and polyethersulfone nanofiber scaffolds for neural differentiation. Carbohydr. Polym. 99, 483–490. doi: 10.1016/j.carbpol.2013.08.050
Duan, H., Weihong, G., Zhang, A., Xi, Y., Chen, Z., Luo, D., et al. (2015). Transcriptome analyses reveal molecular mechanisms underlying functional recovery after spinal cord injury. Proc. Natl. Acad. Sci. U.S.A. 112, 13360–13365. doi: 10.1073/pnas.1510176112
Ebrahimi-Barough, S., Hoveizi, E., Norouzi Javidan, A., and Ai, J. (2015). Investigating the neuroglial differentiation effect of neuroblastoma conditioned medium in human endometrial stem cells cultured on 3D nanofibrous scaffold. J. Biomed. Mater. Res. A 103, 2621–2627. doi: 10.1002/jbm.a.35397
El-hefian, E., Nasef, M., and Yahaya, A. (2011). Chitosan physical forms: a short review. Aust. J. Basic Appl. Sci. 5, 670–677.
El-Knidri, H., Belaabed, R., Addaou, A., Laajeb, A., and Lahsini, A. (2018). Extraction, chemical modification and characterization of chitin and chitosan. Int. J. Biol. Macromol. 120(Pt A), 1181–1189. doi: 10.1016/j.ijbiomac.2018.08.139
Engelhardt, B., Carare, R. O., Bechmann, I., Flügel, A., Laman, J. D., and Weller, R. O. (2016). Vascular, glial, and lymphatic immune gateways of the central nervous system. Acta Neuropathol. 132, 317–338. doi: 10.1007/s00401-016-1606-5
Farrag, M., and Leipzig, N. D. (2018). Subcutaneous maturation of neural stem cell-loaded hydrogels forms region-specific neuroepithelium. Cells 7:173. doi: 10.3390/cells7100173
Feng, X., Lu, X., Huang, D., Xing, J., Feng, G., Jin, G., et al. (2014). 3D porous chitosan scaffolds suit survival and neural differentiation of dental pulp stem cells. Cell. Mol. Neurobiol. 34, 859–870. doi: 10.1007/s10571-014-0063-8
Führmann, T., and Shoichet, M. S. (2018). The role of biomaterials in overcoming barriers to regeneration in the central nervous system. Biomed. Mater. 13:050201. doi: 10.1088/1748-605X/aac2f6
Gáge, F. H., and Temple, S. (2013). Neural stem cells: generating and regenerating the brain. Neuron 80, 588–601. doi: 10.1016/j.neuron.2013.10.037
Gao, S., Zhao, P., Lin, C., Sun, Y., Wang, Y., Zhou, Z., et al. (2014). Differentiation of human adipose-derived stem cells into neuron-like cells which are compatible with photocurable three-dimensional scaffolds. Tissue Eng. Part A 20, 1271–1284. doi: 10.1089/ten.TEA.2012.0773
Gao, Y., Wang, Z. Y., Zhang, J., Zhang, Y., Huo, H., Wang, T., et al. (2014). RVG-peptide-linked trimethylated chitosan for delivery of siRNA to the brain. Biomacromolecules 15, 1010–1018. doi: 10.1021/bm401906p
Ghormade, V., Pathan, E. K., and Deshpande, M. V. (2017). Can fungi compete with marine sources for chitosan production? Int. J. Biol. Macromol. 104(Pt B), 1415–1421. doi: 10.1016/j.ijbiomac.2017.01.112
Giuliani, A., Balducci, A. G., Zironi, E., Colombo, G., Bortolotti, F., Lorenzini, L., et al. (2018). In vivo nose-to-brain delivery of the hydrophilic antiviral ribavirin by microparticle agglomerates. Drug Deliv. 25, 376–387. doi: 10.1080/10717544.2018.1428242
Gnavi, S., Barwig, C., Freier, T., Haastert-Talini, K., Grothe, C., and Geuna, S. (2013). The use of chitosan-based scaffolds to enhance regeneration in the nervous system. Int. Rev. Neurobiol. 109, 1–62. doi: 10.1016/B978-0-12-420045-6.00001-8
Gu, J., Al-Bayati, K., and Ho, E. A. (2017). Development of antibody-modified chitosan nanoparticles for the targeted delivery of siRNA across the blood-brain barrier as a strategy for inhibiting HIV replication in astrocytes. Drug Deliv. Transl. Res. 7, 497–506. doi: 10.1007/s13346-017-0368-5
Gu, Q., Tomaskovic-Crook, E., Lozano, R., Chen, Y., Kapsa, R. M., Zhou, Q., et al. (2016). Functional 3D neural mini-tissues from printed gel-based Bioink and human neural stem cells. Adv. Healthc. Mater. 5, 1429–1438. doi: 10.1002/adhm.201600095
Gupta, P., Agrawal, A., Murali, K., Varshney, R., Beniwal, S., Manhas, S., et al. (2019). Differential neural cell adhesion and neurite outgrowth on carbon nanotube and graphene reinforced polymeric scaffolds. Mater. Sci. Eng. C Mater. Biol. Appl. 97, 539–551. doi: 10.1016/j.msec.2018.12.065
Han, H. W., and Hsu, S. H. (2017). Chitosan derived co-spheroids of neural stem cells and mesenchymal stem cells for neural regeneration. Colloids Surf. B Biointerfaces 158, 527–538. doi: 10.1016/j.colsurfb.2017.07.036
Hao, C., Wang, W., Wang, S., Zhang, L., and Guo, Y. (2017). An overview of the protective effects of chitosan and acetylated chitosan oligosaccharides against neuronal disorders. Mar. Drugs 15:89. doi: 10.3390/md15040089
Hao, P., Duan, H., Hao, F., Chen, L., Sun, M., Fan, K. S., et al. (2017). Neural repair by NT3-chitosan via enhancement of endogenous neurogenesis after adult focal aspiration brain injury. Biomaterials 140, 88–102. doi: 10.1016/j.biomaterials.2017.04.014
He, Q., Liu, J., Liang, J., Liu, X., Li, W., Liu, Z., et al. (2018). Towards improvements for penetrating the blood-brain barrier-recent progress from a material and pharmaceutical perspective. Cells 7:24. doi: 10.3390/cells7040024.
He, Z., and Jin, Y. (2016). Intrinsic control of axon regeneration. Neuron 90, 437–451. doi: 10.1016/j.neuron.2016.04.022
Hoveizi, E., Tavakol, S., and Ebrahimi-Barough, S. (2015). Neuroprotective effect of transplanted neural precursors embedded on PLA/CS scaffold in an animal model of multiple sclerosis. Mol. Neurobiol. 51, 1334–1342. doi: 10.1007/s12035-014-8812-8
Huang, L., Hu, J., Huang, S., Wang, B., Siaw-Debrah, F., Nyanzu, M., et al. (2017). Nanomaterial applications for neurological diseases and central nervous system injury. Prog. Neurobiol. 157, 29–48. doi: 10.1016/j.pneurobio.2017.07.003
Jafarieh, O., Md, S., Ali, M., Baboota, S., Sahni, J. K., Kumari, B., et al. (2015). Design, characterization, and evaluation of intranasal delivery of ropinirole-loaded mucoadhesive nanoparticles for brain targeting. Drug Dev. Ind. Pharm. 41, 1674–1681. doi: 10.3109/03639045.2014.991400
Jain, K. K. (2012). Nanobiotechnology-based strategies for crossing the blood–brain barrier. Nanomedicine 7, 1225–1233. doi: 10.2217/nnm.12.86
Javia, A., and Thakkar, H. (2017). Intranasal delivery of tapentadol hydrochloride-loaded chitosan nanoparticles: formulation, characterisation and its in vivo evaluation. J. Microencapsul. 34, 644–658. doi: 10.1080/02652048.2017.1375038
Jaworska, M. M., and Roberts, G. A. F. (2016). The influence of chitin structure on its enzymatic deacetylation. Chem. Process Eng. 37, 261–267. doi: 10.1515/cpe-2016-0021
Jiang, H. L., Cui, P. F., Xie, R. L., and Cho, C. S. (2014). Chemical modification of chitosan for efficient gene therapy. Adv. Food Nutr. Res. 73, 83–101. doi: 10.1016/B978-0-12-800268-1.00006-8
Joshi, C. R., Labhasetwar, V., and Ghorpade, A. (2017). Destination brain: the past, present, and future of therapeutic gene delivery. J. Neuroimmune Pharmacol. 12, 51–83. doi: 10.1007/s11481-016-9724-3
Karve, I., Taylor, J., and Crack, P. (2015). The contribution of astrocytes and microglia to traumatic brain injury. Br. J. Pharmacol. 173, 692–702. doi: 10.1111/bph.13125
Khan, A., Aqil, M., Imam, S. S., Ahad, A., Sultana, Y., Ali, A., et al. (2018). Temozolomide loaded nano lipid based chitosan hydrogel for nose to brain delivery: characterization, nasal absorption, histopathology and cell line study. Int. J. Biol. Macromol. 116, 1260–1267. doi: 10.1016/j.ijbiomac.2018.05.079
Kievit, F. M., Stephen, Z. R., Wang, K., Dayringer, C. J., Sham, J. G., Ellenbogen, R. G., et al. (2015). Nanoparticle mediated silencing of DNA repair sensitizes pediatric brain tumor cells to gamma-irradiation. Mol. Oncol. 9, 1071–1080. doi: 10.1016/j.molonc.2015.01.006
Kim, S. (2018). Competitive biological activities of Chitosan and its derivatives: antimicrobial, antioxidant, anticancer, and anti-inflammatory activities. Int. J. Polym. Sci. 2018:1708172. doi: 10.1155/2018/1708172
Kim, Y. C., Kim, Y. H., Kim, J. W., and Ha, K. Y. (2016). Transplantation of mesenchymal stem cells for acute spinal cord injury in rats: comparative study between Intralesional injection and scaffold based transplantation. J. Korean Med. Sci. 31, 1373–1382. doi: 10.3346/jkms.2016.31.9.1373
Kumar, M., Pandey, R. S., Patra, K. C., Jain, S. K., Soni, M. L., Dangi, J. S., et al. (2013). Evaluation of neuropeptide loaded trimethyl chitosan nanoparticles for nose to brain delivery. Int. J. Biol. Macromol. 61, 189–195. doi: 10.1016/j.ijbiomac.2013.06.041
Kuo, Y. C., and Chen, C. W. (2017). Neuroregeneration of induced pluripotent stem cells in polyacrylamide-chitosan inverted colloidal crystal scaffolds with Poly(lactide-co-glycolide) nanoparticles and transactivator of transcription von Hippel-Lindau peptide. Tissue Eng. Part A 23, 263–274. doi: 10.1089/ten.TEA.2016.0139
Kuo, Y. C., and Chiu, K. H. (2011). Inverted colloidal crystal scaffolds with laminin-derived peptides for neuronal differentiation of bone marrow stromal cells. Biomaterials 32, 819–831. doi: 10.1016/j.biomaterials.2010.09.057
Kuo, Y. C., Wang, L. J., and Rajesh, R. (2019). Targeting human brain cancer stem cells by curcumin-loaded nanoparticles grafted with anti-aldehyde dehydrogenase and sialic acid: colocalization of ALDH and CD44. Mater. Sci. Eng. C Mater. Biol. Appl. 102, 362–372. doi: 10.1016/j.msec.2019.04.065
Kuo, Y. C., and Yeh, C. F. (2011). Effect of surface-modified collagen on the adhesion, biocompatibility and differentiation of bone marrow stromal cells in poly(lactide-co-glycolide)/chitosan scaffolds. Colloids Surf. B Biointerfaces 82, 624–631. doi: 10.1016/j.colsurfb.2010.10.032
Lakkadwala, S., and Singh, J. (2019). Co-delivery of doxorubicin and erlotinib through liposomal nanoparticles for glioblastoma tumor regression using an in vitro brain tumor model. Colloids Surf. B Biointerfaces 173, 27–35. doi: 10.1016/j.colsurfb.2018.09.047
Leipzig, N. D., Wylie, R. G., Kim, H., and Shoichet, M. S. (2011). Differentiation of neural stem cells in three-dimensional growth factor-immobilized chitosan hydrogel scaffolds. Biomaterials 32, 57–64. doi: 10.1016/j.biomaterials.2010.09.031
Li, H., Koenig, A. M., Sloan, P., and Leipzig, N. D. (2014). In vivo assessment of guided neural stem cell differentiation in growth factor immobilized chitosan-based hydrogel scaffolds. Biomaterials 35, 9049–9057. doi: 10.1016/j.biomaterials.2014.07.038
Li, X., Corbett, A. L., Taatizadeh, E., Tasnim, N., Little, J. P., Garnis, C., et al. (2019). Challenges and opportunities in exosome research—Perspectives from biology, engineering, and cancer therapy. APL Bioeng. 3:011503. doi: 10.1063/1.5087122
Li, X., Yuan, Z., Wei, X., Li, H., Zhao, G., Miao, J., et al. (2016). Application potential of bone marrow mesenchymal stem cell (BMSCs) based tissue-engineering for spinal cord defect repair in rat fetuses with spina bifida aperta. J. Mater. Sci. Mater. Med. 27:77. doi: 10.1007/s10856-016-5684-7
Li, Y., and Ju, D. (2017). “The application, neurotoxicity, and related mechanism of cationic polymers” in Neurotoxicity of Nanomaterials and Nanomedicine, eds X. Jiang and H. Gao (Cambridge, MA: Academic Press), 285–329. doi: 10.1016/b978-0-12-804598-5.00012-x
Liang, K., Bae, K. H., and Kurisawa, M. (2019). Recent advances in the design of injectable hydrogels for stem cell-based therapy. J. Mater. Chem. B 7, 3775–3791. doi: 10.1039/c9tb00485h
Lim, T. C., and Spector, M. (2017). Biomaterials for enhancing CNS repair. Transl. Stroke Res. 8, 57–64. doi: 10.1007/s12975-016-0470-x
Liu, J., Pu, H., Liu, S., Kan, J., and Jin, C. (2017). Synthesis, characterization, bioactivity and potential application of phenolic acid grafted chitosan: a review. Carbohydr. Polym. 174, 999–1017. doi: 10.1016/j.carbpol.2017.07.014
Liu, S., Yang, S., and Ho, P. C. (2018). Intranasal administration of carbamazepine-loaded carboxymethyl chitosan nanoparticles for drug delivery to the brain. Asian J. Pharm. Sci. 13, 72–81. doi: 10.1016/j.ajps.2017.09.001
M Ways, T. M., Lau, W. M., and Khutoryanskiy, V. V. (2018). Chitosan and its derivatives for application in mucoadhesive drug delivery systems. Polymers 10:267. doi: 10.3390/polym10030267
Malhotra, M., Tomaro-Duchesneau, C., and Prakash, S. (2013a). Synthesis of TAT peptide-tagged PEGylated chitosan nanoparticles for siRNA delivery targeting neurodegenerative diseases. Biomaterials 34, 1270–1280. doi: 10.1016/j.biomaterials.2012.10.013
Malhotra, M., Tomaro-Duchesneau, C., Saha, S., and Prakash, S. (2013b). Intranasal, siRNA Delivery to the Brain by TAT/MGF Tagged PEGylated Chitosan Nanoparticles. J. Pharm. 2013:812387. doi: 10.1155/2013/812387
Malmo, J., Sandvig, A., Varum, K., and Strand, S. (2013). Nanoparticle mediated P-glycoprotein silencing for improved drug delivery across the blood-brain barrier: a siRNA-Chitosan approach. PLoS One 8:e54182. doi: 10.1371/journal.pone.0054182
Mao, S., Sun, W., and Kissel, T. (2010). Chitosan-based formulations for delivery of DNA and siRNA. Adv. Drug Deliv. Rev. 62, 12–27. doi: 10.1016/j.addr.2009.08.004
Md, S., Khan, R. A., Mustafa, G., Chuttani, K., Baboota, S., Sahni, J. K., et al. (2013). Bromocriptine loaded chitosan nanoparticles intended for direct nose to brain delivery: pharmacodynamic, pharmacokinetic and scintigraphy study in mice model. Eur. J. Pharm. Sci. 48, 393–405. doi: 10.1016/j.ejps.2012.12.007
Meng, Q., Wang, A., Hua, H., Jiang, Y., Wang, Y., Mu, H., et al. (2018). Intranasal delivery of Huperzine A to the brain using lactoferrin-conjugated N-trimethylated chitosan surface-modified PLGA nanoparticles for treatment of Alzheimer’s disease. Int. J. Nanomedicine 13, 705–718. doi: 10.2147/IJN.S151474
Mittal, D., Md, S., Hasan, Q., Fazil, M., Ali, A., Baboota, S., et al. (2016). Brain targeted nanoparticulate drug delivery system of rasagiline via intranasal route. Drug Deliv. 23, 130–139. doi: 10.3109/10717544.2014.907372
Mohammed, M. A., Syeda, J., Wasan, K. M., and Wasan, E. K. (2017). An overview of chitosan nanoparticles and its application in non-parenteral drug delivery. Pharmaceutics 9, 53–79. doi: 10.3390/pharmaceutics9040053
Moore, L., Skop, N. B., Rothbard, D. E., Corrubia, L. R., and Levison, S. W. (2018). Tethered growth factors on biocompatible scaffolds improve stemness of cultured rat and human neural stem cells and growth of oligodendrocyte progenitors. Methods 133, 54–64. doi: 10.1016/j.ymeth.2017.08.015
Mourya, V. K., and Inamdar, N. N. (2008). Chitosan-modifications and applications: opportunities galore. React. Funct. Polym. 68, 1013–1051. doi: 10.1016/j.reactfunctpolym.2008.03.002
Muanprasat, C., and Chatsudthipong, V. (2017). Chitosan oligosaccharide: biological activities and potential therapeutic applications. Pharmacol. Ther. 170, 80–97. doi: 10.1016/j.pharmthera.2016.10.013
Muñoz, G., and Zuluaga, H. (2017). “Chitosan, chitosan derivatives and their biomedical applications,” in Biological Activities and Application of Marine Polysaccharides, ed. E. Shalaby (Rijeka: InTech), 87–106.
Naghavi-Alhosseini, S., Moztarzadeh, F., Karkhaneh, A., Dodel, M., Khalili, M., Eslami-Arshaghi, T., et al. (2019). Improved cellular response on functionalized polypyrrole interfaces. J. Cell. Physiol. doi: 10.1002/jcp.28173 [Epub ahead of print].
Nagpal, K., Singh, S. K., and Mishra, D. N. (2013). Optimization of brain targeted chitosan nanoparticles of Rivastigmine for improved efficacy and safety. Int. J. Biol. Macromol. 59, 72–83. doi: 10.1016/j.ijbiomac.2013.04.024
Naik, A., and Nair, H. (2014). Formulation and evaluation of thermosensitive biogels for nose to brain delivery of doxepin. Biomed. Res. Int. 2014:847547. doi: 10.1155/2014/847547
Nawrotek, K., Marqueste, T., Modrzejewska, Z., Zarzycki, R., Rusak, A., and Decherchi, P. (2017). Thermogelling chitosan lactate hydrogel improves functional recovery after a C2 spinal cord hemisection in rat. J. Biomed. Mater. Res. A 105, 2004–2019. doi: 10.1002/jbm.a.36067
Nwe, N., Furuike, T., and Tamura, H. (2009). The mechanical and biological properties of Chitosan scaffolds for tissue regeneration templates are significantly enhanced by Chitosan from Gongronella butleri. Materials 2, 374–398. doi: 10.3390/ma2020374
Ong, W. Y., Shalini, S. M., and Constantino, L. (2014). Nose-to-Brain drug delivery by nanoparticles in the treatment of neurological disorders. Curr. Med. Chem. 21, 4247–4256. doi: 10.2174/0929867321666140716103130
Ouyang, Q. Q., Zhao, S., Li, S. D., and Song, C. (2017). Application of Chitosan, Chitooligosaccharide, and their derivatives in the treatment of Alzheimer’s disease. Mar. Drugs 15:322. doi: 10.3390/md15110322
Pangestuti, R., and Kim, S. K. (2010). Neuroprotective properties of chitosan and its derivatives. Mar. Drugs 8, 2117–2128. doi: 10.3390/md8072117
Pardeshi, C. V., and Belgamwar, V. S. (2018). N,N,Ntrimethyl chitosan modified flaxseed oil based mucoadhesive neuronanoemulsions for direct nose to brain drug delivery. Int. J. Biol. Macromol. 120(Pt B), 2560–2571. doi: 10.1016/j.ijbiomac.2018.09.032
Patel, D., Naik, S., Chuttani, K., Mathur, R., Mishra, A. K., and Misra, A. (2013). Intranasal delivery of cyclobenzaprine hydrochloride-loaded thiolated chitosan nanoparticles for pain relief. J. Drug Target. 21, 759–769. doi: 10.3109/1061186X.2013.818676
Patel, D., Naik, S., and Misra, A. (2012). Improved transnasal transport and brain uptake of tizanidine HCl-loaded thiolated chitosan nanoparticles for alleviation of pain. J. Pharm. Sci. 101, 690–706. doi: 10.1002/jps.22780
Payne, S. L., Ballios, B. G., Baumann, M. D., Cooke, M. J., and Shoichet, M. S. (2019). “Central nervous system,” in Principles of Regenerative Medicine, eds A. Atala, R. Lanza, J. Thomson, and R. Nerem (Philadelphia, PA: Elsevier), 1199–1221. doi: 10.1016/b978-0-12-809880-6.00068-0
Pellá, M. C. G., Lima-Tenorio, M. K., Tenorio-Neto, E. T., Guilherme, M. R., Muniz, E. C., and Rubira, A. F. (2018). Chitosan-based hydrogels: from preparation to biomedical applications. Carbohydr. Polym. 196, 233–245. doi: 10.1016/j.carbpol.2018.05.033
Peng, Y. S., Lai, P. L., Peng, S., Wu, H. C., Yu, S., Tseng, T. Y., et al. (2014). Glial cell line-derived neurotrophic factor gene delivery via a polyethylene imine grafted chitosan carrier. Int. J. Nanomedicine 9, 3163–3174. doi: 10.2147/IJN.S60465
Peniche, C., Argüelles-Monal, W., and Goycoolea, F. M. (2008). “Chitin and Chitosan: major sources, properties and applications,” in Monomers, Polymers and Composites from Renewable Resources, eds M. N. Belgacem and A. Gandini (Amsterdam: Elsevier), 517–542.
Pourtalebi-Jahromi, L., Moghaddam-Panah, F., Azadi, A., and Ashrafi, H. (2019). A mechanistic investigation on methotrexate-loaded chitosan-based hydrogel nanoparticles intended for CNS drug delivery: Trojan horse effect or not? Int. J. Biol. Macromol. 125, 785–790. doi: 10.1016/j.ijbiomac.2018.12.093
Qureshi, M., Aqil, M., Imam, S., Ahad, A., and Sultana, Y. (2019). Formulation and evaluation of neuroactive drug loaded Chitosan nanoparticle for nose to brain delivery: in-vitro characterization and in-vivo behavior study. Curr. Drug Deliv. 16, 123–135. doi: 10.2174/1567201815666181011121750
Raj, R., Wairkar, S., Sridhar, V., and Gaud, R. (2018). Pramipexole dihydrochloride loaded chitosan nanoparticles for nose to brain delivery: development, characterization and in vivo anti-Parkinson activity. Int. J. Biol. Macromol. 109, 27–35. doi: 10.1016/j.ijbiomac.2017.12.056
Ramreddy, S., and Janapareddi, K. (2019). Brain targeting of chitosan-based diazepam mucoadhesive microemulsions via nasal route: formulation optimization, characterization, pharmacokinetic and pharmacodynamic evaluation. Drug Dev. Ind. Pharm. 45, 147–158. doi: 10.1080/03639045.2018.1526186
Ransohoff, R. M., and Brown, M. A. (2012). Innate immunity in the central nervous system. J. Clin. Invest. 122, 1164–1171. doi: 10.1002/ana.10764
Rao, J. S., Zhao, C., Zhang, A., Duan, H., Hao, P., Wei, R. H., et al. (2018). NT3-chitosan enables de novo regeneration and functional recovery in monkeys after spinal cord injury. Proc. Natl. Acad. Sci. U.S.A. 115, E5595–E5604. doi: 10.1073/pnas.1804735115
Rassu, G., Soddu, E., Cossu, M., Gavini, E., Giunchedi, P., and Dalpiaz, A. (2016). Particulate formulations based on chitosan for nose-to-brain delivery of drugs, A review. J. Drug Deliv. Sci. Technol. 32, 77–87. doi: 10.1016/j.jddst.2015.05.002
Rassu, G., Soddu, E., Maria, P., Pintus, G., Sarmento, B., Giunchedi, P., et al. (2017). Nose-to-brain delivery of BACE1 siRNA loaded in solid lipid nanoparticles for Alzheimer’s therapy. Colloids Surf. B Biointerfaces 152, 296–301. doi: 10.1016/j.colsurfb.2017.01.031
Rebelo, R., Fernandes, M., and Fangueiro, R. (2017). Biopolymers in medical implants: a brief review. Procedia Eng. 200, 236–243. doi: 10.1016/j.proeng.2017.07.034
Ricks, C. B., Shin, S. S., Becker, C., and Grandhi, R. (2014). Extracellular matrices, artificial neural scaffolds and the promise of neural regeneration. Neural Regen. Res. 9, 1573–1577. doi: 10.4103/1673-5374.141778
Rinaldi, F., Seguella, L., Gigli, S., Hanieh, P. N., Del Favero, E., Cantu, L., et al. (2019). inPentasomes: an innovative nose-to-brain pentamidine delivery blunts MPTP parkinsonism in mice. J. Control. Release 294, 17–26. doi: 10.1016/j.jconrel.2018.12.007
Rodriguez-Vazquez, M., Vega-Ruiz, B., Ramos-Zuniga, R., Saldana-Koppel, D. A., and Quinones-Olvera, L. F. (2015). Chitosan and Its potential use as a scaffold for tissue engineering in regenerative medicine. Biomed Res. Int. 2015:821279. doi: 10.1155/2015/821279
Ruppen, W. (2015). Comparison of Oral Morphine Versus Nasal Ketamine Spray with Chitosan in Cancer Pain Outpatients (ONKEMI). Basel: University Hospital.
Sadeghi, A., Moztarzadeh, F., and Aghazadeh Mohandesi, J. (2019). Investigating the effect of chitosan on hydrophilicity and bioactivity of conductive electrospun composite scaffold for neural tissue engineering. Int. J. Biol. Macromol. 121, 625–632. doi: 10.1016/j.ijbiomac.2018.10.022
Sanchez-Ramos, J., Song, S., Kong, X., Foroutan, P., Martinez, G., Dominguez-Viqueria, W., et al. (2018). Chitosan-Mangafodipir nanoparticles designed for intranasal delivery of siRNA and DNA to brain. J. Drug Deliv. Sci. Technol. 43, 453–460. doi: 10.1016/j.jddst.2017.11.013
Sarvaiya, J., and Agrawal, Y. K. (2015). Chitosan as a suitable nanocarrier material for anti-Alzheimer drug delivery. Int. J. Biol. Macromol. 72, 454–465. doi: 10.1016/j.ijbiomac.2014.08.052
Scanga, V. I., Goraltchouk, A., Nussaiba, N., Shoichet, M. S., and Morshead, C. M. (2010). Biomaterials for neural-tissue engineering — Chitosan supports the survival, migration, and differentiation of adult-derived neural stem and progenitor cells. Can. J. Chem. 88, 277–287. doi: 10.1139/v09-171
Sedeky, A. S., Khalil, I. A., Hefnawy, A., and El-Sherbiny, I. M. (2018). Development of core-shell nanocarrier system for augmenting piperine cytotoxic activity against human brain cancer cell line. Eur. J. Pharm. Sci. 118, 103–112. doi: 10.1016/j.ejps.2018.03.030
Shah, B., Khunt, D., Misra, M., and Padh, H. (2016). Non-invasive intranasal delivery of quetiapine fumarate loaded microemulsion for brain targeting: formulation, physicochemical and pharmacokinetic consideration. Eur. J. Pharm. Sci. 91, 196–207. doi: 10.1016/j.ejps.2016.05.008
Shah, B., Khunt, D., Misra, M., and Padh, H. (2018). Formulation and in-vivo pharmacokinetic consideration of intranasal microemulsion and mucoadhesive microemulsion of rivastigmine for brain targeting. Pharm. Res. 35:8. doi: 10.1007/s11095-017-2279-z
Shariatinia, Z. (2018). Carboxymethyl chitosan: properties and biomedical applications. Int. J. Biol. Macromol. 120(Pt B), 1406–1419. doi: 10.1016/j.ijbiomac.2018.09.131
Shariatinia, Z., and Jalali, A. M. (2018). Chitosan-based hydrogels: preparation, properties and applications. Int. J. Biol. Macromol. 115, 194–220. doi: 10.1016/j.ijbiomac.2018.04.034
Sharma, S., Lohan, S., and Murthy, R. S. R. (2014). Formulation and characterization of intranasal mucoadhesive nanoparticulates and thermo-reversible gel of levodopa for brain delivery. Drug Dev. Ind. Pharm. 40, 869–878. doi: 10.3109/03639045.2013.789051
Singh, D., Rashid, M., Hallan, S. S., Mehra, N. K., Prakash, A., and Mishra, N. (2016). Pharmacological evaluation of nasal delivery of selegiline hydrochloride-loaded thiolated chitosan nanoparticles for the treatment of depression. Artif. Cells Nanomed. Biotechnol. 44, 865–877. doi: 10.3109/21691401.2014.998824
Skop, N. B., Calderon, F., Cho, C. H., Gandhi, C. D., and Levison, S. W. (2016). Optimizing a multifunctional microsphere scaffold to improve neural precursor cell transplantation for traumatic brain injury repair. J. Tissue Eng. Regen. Med. 10, E419–E432. doi: 10.1002/term.1832
Skop, N. B., Calderon, F., Levison, S. W., Gandhi, C. D., and Cho, C. H. (2013). Heparin crosslinked chitosan microspheres for the delivery of neural stem cells and growth factors for central nervous system repair. Acta Biomater. 9, 6834–6843. doi: 10.1016/j.actbio.2013.02.043
Skop, N. B., Singh, S., Antikainen, H., Saqcena, C., Calderon, F., Rothbard, D. E., et al. (2019). Subacute transplantation of native and genetically engineered neural progenitors seeded on microsphere scaffolds promote repair and functional recovery after traumatic brain injury. ASN Neuro 11:1759091419830186. doi: 10.1177/1759091419830186
Sreenivas, S. A., and Pai, K. V. (2008). Thiolated chitosans: novel polymers for Mucoadhesive drug delivery – a review. Trop. J. Pharm. Res. 7, 1077–1088.
Sridhar, V., Gaud, R., Bajaj, A., and Wairkar, S. (2018). Pharmacokinetics and pharmacodynamics of intranasally administered selegiline nanoparticles with improved brain delivery in Parkinson’s disease. Nanomedicine 14, 2609–2618. doi: 10.1016/j.nano.2018.08.004
Stewart, S. A., Dominguez-Robles, J., Donnelly, R. F., and Larraneta, E. (2018). Implantable polymeric drug delivery devices: classification, manufacture, materials, and clinical applications. Polymers 10:E1379. doi: 10.3390/polym10121379
Sugai, K., Nishimura, S., Kato-Negishi, M., Onoe, H., Iwanaga, S., Toyama, Y., et al. (2015). Neural stem/progenitor cell-laden microfibers promote transplant survival in a mouse transected spinal cord injury model. J. Neurosci. Res. 93, 1826–1838. doi: 10.1002/jnr.23636
Sun, Y., Yang, C., Zhu, X., Wang, J. J., Liu, X. Y., Yang, X. P., et al. (2019). 3D printing collagen/chitosan scaffold ameliorated axon regeneration and neurological recovery after spinal cord injury. J. Biomed. Mater. Res. A 107, 1898–1908. doi: 10.1002/jbm.a.36675
Sunena, Singh, S. K., and Mishra, D. N. (2019). Nose to brain delivery of Galantamine loaded nanoparticles: in-vivo pharmacodynamic and biochemical study in mice. Curr. Drug Deliv. 16, 51–58. doi: 10.2174/1567201815666181004094707
Sung, C. Y., Yang, C. Y., Chen, W. S., Wang, Y. K., Yeh, J. A., and Cheng, C. M. (2015). Probing neural cell behaviors through micro-/nano-patterned chitosan substrates. Biofabrication 7:045007. doi: 10.1088/1758-5090/7/4/045007
Tan, K., Wang, X., Zhang, J., Zhuang, Z., and Dong, T. (2018). Effect of chitosan porous scaffolds combined with bone marrow mesenchymal stem cells in repair of neurological deficit after traumatic brain injury in rats. Zhongguo Xiu Fu Chong Jian Wai Ke Za Zhi 32, 745–752. doi: 10.7507/1002-1892.201712047
Tedeschi, A., and Bradke, F. (2017). Spatial and temporal arrangement of neuronal intrinsic and extrinsic mechanisms controlling axon regeneration. Curr. Opin. Neurobiol. 42, 118–127. doi: 10.1016/j.conb.2016.12.005
Tong, G. F., Qin, N., and Sun, L. W. (2017). Development and evaluation of Desvenlafaxine loaded PLGA-chitosan nanoparticles for brain delivery. Saudi Pharm. J. 25, 844–851. doi: 10.1016/j.jsps.2016.12.003
Tseng, T. C., Tao, L., Hsieh, F. Y., Wei, Y., Chiu, I. M., and Hsu, S. H. (2015). An injectable, self-healing hydrogel to repair the central nervous system. Adv. Mater. 27, 3518–3524. doi: 10.1002/adma.201500762
Turabee, M. H., Jeong, T. H., Ramalingam, P., Kang, J. H., and Ko, Y. T. (2019). N,N,N-trimethyl chitosan embedded in situ Pluronic F127 hydrogel for the treatment of brain tumor. Carbohydr. Polym. 203, 302–309. doi: 10.1016/j.carbpol.2018.09.065
Tysseling, V. M., and Kessler, J. A. (2017). “6.19 biomaterials for central nervous system regeneration,” in Comprehensive Biomaterials, ed. P. Ducheyne (Amsterdam: Elsevier), 321–333. doi: 10.1016/b978-0-08-100691-7.00036-7
Tzeyung, A. S., Md, S., Bhattamisra, S. K., Madheswaran, T., Alhakamy, N. A., Aldawsari, H. M., et al. (2019). Fabrication, optimization, and evaluation of rotigotine-loaded Chitosan nanoparticles for nose-to-brain delivery. Pharmaceutics 11:26. doi: 10.3390/pharmaceutics11010026
Upadhyay, R. K. (2014). Drug delivery systems, CNS protection, and the blood brain barrier. Biomed Res. Int. 2014:869269. doi: 10.1155/2014/869269
Upadhyaya, L., Singh, J., Agarwal, V., and Tewari, R. (2014). The implications of recent advances in carboxymethyl chitosan based targeted drug delivery and tissue engineering applications. J. Control. Release 186, 54–87. doi: 10.1016/j.jconrel.2014.04.043
Van Woensel, M., Mathivet, T., Wauthoz, N., Rosiere, R., Garg, A. D., Agostinis, P., et al. (2017). Sensitization of glioblastoma tumor micro-environment to chemo- and immunotherapy by Galectin-1 intranasal knock-down strategy. Sci. Rep. 7:1217. doi: 10.1038/s41598-017-01279-1
Van Woensel, M., Wauthoz, N., Rosiere, R., Mathieu, V., Kiss, R., Lefranc, F., et al. (2016). Development of siRNA-loaded chitosan nanoparticles targeting Galectin-1 for the treatment of glioblastoma multiforme via intranasal administration. J. Control. Release 227, 71–81. doi: 10.1016/j.jconrel.2016.02.032
Varan, C., and Bilensoy, E. (2017). Cationic PEGylated polycaprolactone nanoparticles carrying post-operation docetaxel for glioma treatment. Beilstein J. Nanotechnol. 8, 1446–1456. doi: 10.3762/bjnano.8.144
Wahba, S. M., Darwish, A. S., and Kamal, S. M. (2016). Ceria-containing uncoated and coated hydroxyapatite-based galantamine nanocomposites for formidable treatment of Alzheimer’s disease in ovariectomized albino-rat model. Mater. Sci. Eng. C Mater. Biol. Appl. 65, 151–163. doi: 10.1016/j.msec.2016.04.041
Wang, S., Guan, S., Li, W., Ge, D., Xu, J., Sun, C., et al. (2018). 3D culture of neural stem cells within conductive PEDOT layer-assembled chitosan/gelatin scaffolds for neural tissue engineering. Mater. Sci. Eng. C Mater. Biol. Appl. 93, 890–901. doi: 10.1016/j.msec.2018.08.054
Wang, S., Sun, C., Guan, S., Li, W., Xu, J., Ge, D., et al. (2017). Chitosan/gelatin porous scaffolds assembled with conductive poly(3,4-ethylenedioxythiophene) nanoparticles for neural tissue engineering. J. Mater. Chem. B 5, 4774–4788. doi: 10.1039/c7tb00608j
Wang, Y., Tan, H., and Hui, X. (2018). Biomaterial scaffolds in regenerative therapy of the central nervous system. Biomed Res. Int. 2018:7848901. doi: 10.1155/2018/7848901
Watanabe, T. K. (2018). A review of stem cell therapy for acquired brain injuries and neurodegenerative central nervous system diseases. PM R 10(9 Suppl. 2), S151–S156. doi: 10.1016/j.pmrj.2018.07.008
Wei, H., Lai, S., Wei, J., Yang, L., Jiang, N., Wang, Q., et al. (2018). A novel delivery method of Cyclovirobuxine D for brain-targeting: chitosan coated nanoparticles loading Cyclovirobuxine D by Intranasal administration. J. Nanosci. Nanotechnol.. 18, 5274–5282. doi: 10.1166/jnn.2018.15371
Weil, Z. M., Norman, G. J., DeVries, A. C., and Nelson, R. J. (2008). The injured nervous system: a Darwinian perspective. Prog. Neurobiol. 86, 48–59. doi: 10.1016/j.pneurobio.2008.06.001
Xu, C., Guan, S., Wang, S., Gong, W., Liu, T., Ma, X., et al. (2018). Biodegradable and electroconductive poly(3,4-ethylenedioxythiophene)/carboxymethyl chitosan hydrogels for neural tissue engineering. Mater. Sci. Eng. C Mater. Biol. Appl. 84, 32–43. doi: 10.1016/j.msec.2017.11.032
Xu, H., Nie, X., Wu, L., Zhu, X., Yi, W., and Huang, S. (2015). Down-Regulation of MRP1 Expression in C6/VP16 Cells by Chitosan-MRP1-siRNA Nanoparticles. Cell Biochem. Biophys. 72, 227–233. doi: 10.1007/s12013-014-0442-2
Xu, Y., Xia, D., Han, J., Yuan, S., Lin, H., and Zhao, C. (2017). Design and fabrication of porous chitosan scaffolds with tunable structures and mechanical properties. Carbohydr. Polym. 177, 210–216. doi: 10.1016/j.carbpol.2017.08.069
Yan, F., Li, M., Zhang, H. Q., Li, G. L., Hua, Y., Shen, Y., et al. (2019). Collagen-chitosan scaffold impregnated with bone marrow mesenchymal stem cells for treatment of traumatic brain injury. Neural Regen. Res. 14, 1780–1786. doi: 10.4103/1673-5374.257533
Yan, F., Yue, W., Zhang, Y. L., Mao, G. C., Gao, K., Zuo, Z. X., et al. (2015). Chitosan-collagen porous scaffold and bone marrow mesenchymal stem cell transplantation for ischemic stroke. Neural Regen. Res. 10, 1421–1426. doi: 10.4103/1673-5374.163466
Yang, H. C., and Hon, M. H. (2009). The effect of the molecular weight of chitosan nanoparticles and its application on drug delivery. Microchem. J. 92, 87–91. doi: 10.1016/j.microc.2009.02.001
Yao, Z. A., Chen, F. J., Cui, H. L., Lin, T., Guo, N., and Wu, H. G. (2018). Efficacy of chitosan and sodium alginate scaffolds for repair of spinal cord injury in rats. Neural Regen. Res. 13, 502–509. doi: 10.4103/1673-5374.228756
Yi, X., Jin, G., Tian, M., Mao, W., and Qin, J. (2011). Porous chitosan scaffold and NGF promote neuronal differentiation of neural stem cells in vitro. Neuroendocrinol. Lett. 32, 705–710.
Younes, I., and Rinaudo, M. (2015). Chitin and chitosan preparation from marine sources, Structure, properties and applications. Mar. Drugs 13, 1133–1174. doi: 10.3390/md13031133
Youssef, A. E. H., Dief, A. E., El Azhary, N. M., Abdelmonsif, D. A., and El-Fetiany, O. S. (2019). LINGO-1 siRNA nanoparticles promote central remyelination in ethidium bromide-induced demyelination in rats. J. Physiol. Biochem. 75, 89–99. doi: 10.1007/s13105-018-00660-6
Yu, L. M., Kazazian, K., and Shoichet, M. S. (2007). Peptide surface modification of methacrylamide chitosan for neural tissue engineering applications. J. Biomed. Mater. Res. A 82, 243–255. doi: 10.1002/jbm.a.31069
Yu, S., Xu, X., Feng, J., Liu, M., and Hu, K. (2019). Chitosan and chitosan coating nanoparticles for the treatment of brain disease. Int. J. Pharm. 560, 282–293. doi: 10.1016/j.ijpharm.2019.02.012
Zargar, V., Asghari, M., and Dashti, A. (2015). A review on chitin and chitosan polymers: structure, chemistry, solubility, derivatives, and applications. ChemBioEng Rev. 2, 204–226. doi: 10.1002/cben.201400025
Zhang, J., Lu, X., Feng, G., Gu, Z., Sun, Y., Bao, G., et al. (2016). Chitosan scaffolds induce human dental pulp stem cells to neural differentiation: potential roles for spinal cord injury therapy. Cell Tissue Res. 366, 129–142. doi: 10.1007/s00441-016-2402-1
Keywords: chitosan, chitosan derivatives, central nervous system, drug delivery, tissue engineering, regenerative medicine
Citation: Ojeda-Hernández DD, Canales-Aguirre AA, Matias-Guiu J, Gomez-Pinedo U and Mateos-Díaz JC (2020) Potential of Chitosan and Its Derivatives for Biomedical Applications in the Central Nervous System. Front. Bioeng. Biotechnol. 8:389. doi: 10.3389/fbioe.2020.00389
Received: 02 December 2019; Accepted: 07 April 2020;
Published: 05 May 2020.
Edited by:
John Forsythe, Monash University, AustraliaReviewed by:
Giovanna Rassu, University of Sassari, ItalyPaolo Giunchedi, University of Sassari, Italy
Ryan James Gilbert, Rensselaer Polytechnic Institute, United States
Copyright © 2020 Ojeda-Hernández, Canales-Aguirre, Matias-Guiu, Gomez-Pinedo and Mateos-Díaz. This is an open-access article distributed under the terms of the Creative Commons Attribution License (CC BY). The use, distribution or reproduction in other forums is permitted, provided the original author(s) and the copyright owner(s) are credited and that the original publication in this journal is cited, in accordance with accepted academic practice. No use, distribution or reproduction is permitted which does not comply with these terms.
*Correspondence: Juan C. Mateos-Díaz, jcmateos@ciatej.mx