Meniscal Regenerative Scaffolds Based on Biopolymers and Polymers: Recent Status and Applications
- 1The First Medical Center, Chinese PLA General Hospital, Institute of Orthopedics, Beijing, China
- 2Beijing Key Lab of Regenerative Medicine in Orthopedics, Beijing, China
- 3Key Laboratory of Musculoskeletal Trauma and War Injuries PLA, Beijing, China
- 4School of Medicine, Nankai University, Tianjin, China
- 5Department of Bone and Joint Surgery, Renji Hospital, School of Medicine, Shanghai Jiao Tong University, Shanghai, China
Knee menisci are structurally complex components that preserve appropriate biomechanics of the knee. Meniscal tissue is susceptible to injury and cannot heal spontaneously from most pathologies, especially considering the limited regenerative capacity of the inner avascular region. Conventional clinical treatments span from conservative therapy to meniscus implantation, all with limitations. There have been advances in meniscal tissue engineering and regenerative medicine in terms of potential combinations of polymeric biomaterials, endogenous cells and stimuli, resulting in innovative strategies. Recently, polymeric scaffolds have provided researchers with a powerful instrument to rationally support the requirements for meniscal tissue regeneration, ranging from an ideal architecture to biocompatibility and bioactivity. However, multiple challenges involving the anisotropic structure, sophisticated regenerative process, and challenging healing environment of the meniscus still create barriers to clinical application. Advances in scaffold manufacturing technology, temporal regulation of molecular signaling and investigation of host immunoresponses to scaffolds in tissue engineering provide alternative strategies, and studies have shed light on this field. Accordingly, this review aims to summarize the current polymers used to fabricate meniscal scaffolds and their applications in vivo and in vitro to evaluate their potential utility in meniscal tissue engineering. Recent progress on combinations of two or more types of polymers is described, with a focus on advanced strategies associated with technologies and immune compatibility and tunability. Finally, we discuss the current challenges and future prospects for regenerating injured meniscal tissues.
Introduction
The importance of the meniscus in knee homeostasis has been widely acknowledged; unfortunately, meniscus-related injurie are quite common. According to the epidemiologic data reported by Logerstedt et al. (2010), the incidence rate of meniscus injury was 12–14%, and the prevalence was 61 cases per 100,000 persons. In the United States, injuries to the menisci are the most common injury to the knee, and 10–20% of all orthopedic surgeries involve surgical procedures to the meniscus (Montgomery et al., 2013). Nearly one million meniscal surgeries are conducted annually in the United States, most of which consist of partial or total meniscectomy, and the total cost for inpatient stays ranges from $500 million to $5 billion (Jones et al., 2012; Fillingham et al., 2017). Of the two genders, men were the most likely to experience meniscal tears, with reported ratios between 3:1 and 4:1 (Murphy et al., 2019).
Given the severe physical and psychological burden on individuals and the socioeconomic burden brought about by meniscal injuries, studies on the treatment and pathology of meniscal injuries are worthwhile endeavors. Meniscal tears, similar to many other musculoskeletal diseases, mainly occur in sports-related activities (32%) and non-sports-related activities (38%) and can arise from any specific event (28%) (Drosos and Pozo, 2004). As a result of the combination of axial impact forces and rotational forces between the femoral condyles and the tibial plateau, shear force may cause acute and degenerative tears of the meniscus, and these injuries are more likely to occur in the medial meniscus (Twomey-Kozak and Jayasuriya, 2020). Tears occurring in the inner avascular region of the meniscus are commonly complex and thorny and are often associated with a poor prognosis after surgical repair (Makris et al., 2011). In addition to the symptoms and motor dysfunctions, knee osteoarthritis (OA) is a common pathological response to meniscal injury (Englund et al., 2012). Briefly, meniscal degeneration or meniscectomy results in consistent articular cartilage overloading, leading to the development of OA (Katz et al., 2013). Therefore, preserving as much meniscal tissue as possible has become a widely prevailing trend (Crema et al., 2010).
Recently, considerable efforts have been put into meniscal regeneration rather than meniscal resection. The complex array of meniscal tissue structure and avascularity presents quite a thorny problem for clinicians; thus, tissue engineering aimed at tissue remodeling and functional restoration seems to be an alternative strategy (Twomey-Kozak and Jayasuriya, 2020). From traditional allograft menisci to currently used polymer materials, tissue-engineered meniscus scaffolds have been continuously progressing. First, the primary goal of meniscal tissue engineering is to develop a bioartificial substitute presenting the same level of components and architectures as native menisci. The design of structural-composition biomimetic scaffolds based on natural polymers, synthetic polymers, and a combination of multiple polymers has demonstrated fascinating meniscal regenerative capabilities. Furthermore, one major concern regarding successful meniscus regeneration was that how the polymeric scaffold reconstructs the zonal difference in the red and white zones of the meniscus. Generally, the tissue-engineered scaffold should mimic the zone-dependent arrangement of collagen fibers, ECM composition, and different bioactive inducers. In recent, intensive researches have been developed via advanced techniques and achieved promising repairing results. Therefore, we will subsequently focus on the recent development of various kinds of polymeric scaffolds in meniscal repair, with additional attention paid to reproduce zonal variations of meniscus.
Second, despite the importance of tissue-engineered scaffolds with characteristics that recapitulate the structure and composition of the meniscus, there are still many obstacles for the use of current materials to recreate a natural inducive microenvironment close to native meniscal tissue (Chen M. et al., 2019). The general design criteria of novel polymeric scaffolds are thus to recreate the main properties of the native microenvironment in terms of microarchitecture, components, and pro-regenerative features in order to stimulate cellular growth and maintain cell phenotype (da Silva Morais et al., 2020). Among several processing technologies, three-dimensional (3D) printing is one of the most appropriate for meniscal scaffold construction due to its highly accurate control of scaffold microstructures and compositions, making it possible to meet the primary requirements of meniscal tissue engineering. In addition, polymeric scaffolds not only act as temporary templates for neotissue formation and integration but also interact with cells and bioactive factors to orchestrate tissue remodeling (Zhang and King, 2020). 3D printing has also been applied in drug delivery (Qu et al., 2021); ideally, with personalized 3D architectures and programmed drug release profiles, these engineered meniscal scaffolds are very promising for enhancing meniscal regeneration. Despite the advances of numerous polymeric biomaterials, the immunocompatibility and immunomodulation of meniscal grafts have not been developed and require further exploration. Clearly, all these important polymers and some advances in meniscal tissue engineering thus need to be introduced, along with a richer knowledge base in this field, so that we can design a biomaterial-based meniscal scaffold that functionally recreates almost all of the aspects needed.
In this review, the relevant polymers involved in meniscal repair and regeneration are presented after a brief introduction to the anatomy, biochemical content, cells, and biomechanical properties of the meniscus, as well as a summary of conventional therapies. Then, we provide an overview of the different polymers and relevant scaffolds studied to date, with particular attention given to discussing the strategies reported recently on how to construct hybrid scaffolds to achieve versatile functions. Subsequently, we introduce additive manufacturing technologies used to promote the meniscus in vitro and in vivo, the recent advancement on zonal meniscal reconstruction and the effect of the applied biopolymers on the immune response and tissue regeneration were also discussed. Finally, we describe the main challenges and future development directions in advancing meniscal regeneration approaches.
Meniscus Anatomy, Physiology, and Conventional Treatments
Meniscus Anatomy and Cellular Components
The meniscus is a pair of crescent, wedge-shaped fibrocartilaginous pads located between the femoral condyle and tibial plateau that serve a variety of functions, such as distributing loads, absorbing shock, maintaining stability, and contributing to cartilage lubrication and nutrition (Makris et al., 2011; Rongen et al., 2014). From a macroscopic point of view, the medial and lateral menisci possess their own anatomical variations, but the anterior horns of both are connected by the anterior intermeniscal ligament (Rongen et al., 2014; Figure 1A). Since vascularization of the meniscus decreases as the meniscus matures, the limited healing capacity of the inner zone of the meniscus is directly related to the poor blood supply, and nutrients can only be received from passive synovial fluid diffusion (Arnoczky and Warren, 1982; Petersen and Tillmann, 1995; Makris et al., 2011). Microscopically, it is reasonable to distinguish the peripheral red zone from the inner avascular white zone (Murphy et al., 2019). The inner zone is characterized by chondrocyte-like cells embedded in collagen type II and glycosaminoglycans (GAGs). In contrast to the inner zone, the peripheral zone presents abundant collagen type I deposition and many more elongated fibroblast-like cells (Makris et al., 2011; Jacob et al., 2020). In addition, the cells within the superficial zone are postulated to produce and secrete lubricant and anti-adhesive proteins or act as progenitor cells with regenerative potential (Lee et al., 2008; Jacob et al., 2020; Figure 1B).
Meniscus Mechanical Properties and Functions
Generally, the meniscus plays a critical role in maintaining normal knee joint mechanics and functions. A number of studies have been performed to measure the mechanical strength of meniscal tissue in humans (Table 1). The specific mechanical properties of the meniscus are mainly determined by highly spatially oriented collagen fibers (Rongen et al., 2014). Indeed, the most important role of the collagen-proteoglycan meniscal matrix is its capacity to provide mechanical support, such as resistance to tension, compression and shear stress (Fithian et al., 1990). Specifically, the meniscus transfers 50–90% of the joint reaction forces under weight-bearing conditions (Walker and Erkman, 1975; Ahmed and Burke, 1983), with load transfer and absorption occurring via well-characterized mechanisms. In general, circumferential stresses within meniscal tissue are generated after joint surface contact, transferring compressive loads into horizontal tensile stress. Excessive energy being absorbed by collagen can also be released via the expulsion of synovial fluid (Storm et al., 2005; Rongen et al., 2014). Other important functions of the meniscus include providing lubrication, supplying nutrients to the cartilage and supporting proprioception (Jacob et al., 2020).
Meniscus Pathologies and Conventional Therapies
In addition to trauma, other risk factors affect meniscal tissue, such as genetic susceptibility, obesity and knee malalignment (Englund et al., 2012). Twisting or shearing motions with a varus or valgus force account for the mechanism of most meniscal tears (Pihl et al., 2017). For younger patients, acute traumatic injury is a major cause of meniscal tears, and as in elderly patients, degenerative meniscal tears might act as key factors in the development of knee OA (Englund et al., 2007, 2012). The healing capacity of the meniscus after injury is basically dictated by the tear pattern and location. For instance, horizontal and radial tears involving the inner zone are thought to have the least healing potential owing to incursion into the avascular inner zone (Kwon et al., 2019). Unfortunately, meniscal injury is often followed by knee OA, which is known as the “meniscal pathway.” Briefly, loss of meniscal mechanical support leads to dramatically increased structural stress on articular cartilage, causing loss of cartilage, subchondral bone changes, and bone marrow lesions (Englund et al., 2012). In addition, the subsequent increased proinflammatory state within the knee joint after meniscal tears contributes to the progression of OA (Bigoni et al., 2017).
Conventional therapies for meniscal tears include both non-surgical and surgical approaches (Li et al., 2020a). Arthroscopic meniscectomy is the most commonly used surgical procedure for meniscal injuries (Katz and Martin, 2009; Kim et al., 2011b). However, it inevitably results in progressive cartilage degeneration and OA, and the curative effect on degenerative meniscal tears remains a matter of debate (Fairbank, 1948; Roos et al., 1998; Monk et al., 2017). Meniscal allograft transplantation (MAT) may further restore knee function, but this advantage is countered by the disadvantages of the insufficient number of donors and risk of disease transmission, immune rejection and non-matching (Noyes and Barber-Westin, 2010; Noyes and Barber-Westin, 2016; Parkinson et al., 2016). Chondroprotective evidence also needs to be validated (Vrancken et al., 2013). In search of a clinical solution for meniscal injury and joint homeostasis restoration, tissue engineering and scaffold-based regenerative medicine strategies have become some of the most promising approaches (Bandyopadhyay and Mandal, 2019). In this context, this review focuses on details of the polymeric aspects of meniscal therapy and advanced, novel polymeric scaffold-based strategies for meniscal repair and regeneration.
Polymeric Scaffold-Based Strategies for Meniscal Regeneration
Various Factors Involved in Polymeric Scaffold-Based Strategies
Tissue engineering techniques often involve the application of scaffolds, cells and biochemical and biomechanical stimuli to create engineered tissues (Kwon et al., 2019). These three main components collectively form many combinations and have obtained some promising advances. Therefore, to better understand the interaction among these three main components, cells and physical and biochemical signals all need to be introduced. Cells are important players in meniscal tissue engineering. Stem/progenitor/multipotent cell sources in meniscal tissue engineering can be obtained from various tissues, including bone marrow, synovium, and adipose tissue (Bilgen et al., 2018). Another large family of cells originates from mature connective tissue, such as the meniscus and cartilage (Peretti et al., 2004; Zellner et al., 2017). Biochemical stimuli also play an important role in engineering meniscal scaffolds. To increase extracellular matrix (ECM) production in engineered meniscal tissue, the administration of biochemical stimuli, such as growth factors, has long been used. A variety of growth factors, including platelet-derived growth factor (PDGF), bone morphogenetic protein-2 (BMP-2), transforming growth factor-β (TGF-β), insulin-like growth factor-1 (IGF-1), and fibroblast growth factor (FGF), have shown efficacy in improving meniscal regeneration (Bhargava et al., 1999; Pangborn and Athanasiou, 2005; Gunja and Athanasiou, 2010; Puetzer et al., 2013). Changes in oxygen tension have also yielded mixed effects on engineered meniscal tissue, which showed improved ACAN and COL2A1 expression under hypoxic conditions (Liang et al., 2017). On the other hand, the development of biomechanical stimuli for meniscal tissue engineering has focused on replicating heterogeneity and matrix-level arrangement of the tissue (Kwon et al., 2019). For example, compression and hydrostatic pressure have been used to improve the functional properties of meniscal neotissue (MacBarb et al., 2013; Zellner et al., 2015; Puetzer and Bonassar, 2016).
Consideration of Polymeric Scaffold Design
Generally, scaffold design is of pivotal importance to accelerate meniscal tissue repair and regeneration. Polymer selection and biophysical and biochemical properties all need to be taken into consideration when designing an optimal tissue-engineered meniscal scaffold.
It is well known that biocompatibility and bioactivity are major considerations that may lead to scar tissue formation if not achieved (Murphy et al., 2018). In meniscal tissue engineering, a microenvironment conducive to cell adhesion, cell proliferation and matrix synthesis is necessary (Tan and Cooper-White, 2011). Natural ECM is a sophisticated 3D network that can support cells and control cellular responses, such as migration, proliferation and differentiation, via autocrine and paracrine mechanisms (Murphy et al., 2018; Subbiah and Guldberg, 2019). Therefore, compositionally, the scaffold needs to create an ECM-mimicking microenvironment with biocompatibility and minor immune rejection and degrade into harmless products along with meniscal tissue growth. The biophysical properties of natural ECM can also modulate cell behaviors (Elliott et al., 2019; Gaharwar et al., 2020). In regard to the architecture, the anisotropic orientation as well as suitable pore size and porosity are required to provide an optimal structure for cell ingrowth. In addition, scaffolds are also required to have appropriate mechanical properties, which enable the scaffold to preserve the normal contact biomechanics of the knee. In summary, biomaterial design and fabrication should mimic the biomechanics and components of natural ECM in meniscal regeneration (Ma, 2008; Zhou and Lee, 2011). Furthermore, the processing techniques should be convenient and versatile enough for clinically customized application. The specific design criteria of meniscal scaffolds are summarized in Table 2. To apply polymeric scaffold-based regenerative strategies in the context of the meniscus, clarification of the key role of scaffolds in meniscal tissue remodeling and maturation is needed. Collectively, a polymeric scaffold should not only provide a supportive microenvironment but also favor the migration, proliferation and differentiation of meniscogenic cells (Figure 2). In this review, we will summarize recent developments in polymeric scaffolds in terms of compositions, structures, processing technologies and bioactivities.
Polymers for Meniscal Tissue Engineering Applications
Researchers consistently utilize natural and synthetic polymers or their combinations as engineered scaffolds and have demonstrated their promising properties for meniscal regeneration (Murphy et al., 2018). The advantages and limitations of commonly used polymers for meniscal tissue engineering are summarized in Table 3. Considering their excellent biocompatibility, processability and ECM-mimicking cues, natural polymers, such as collagen, silk fibroin (SF), and chitosan, present defined advantages for tissue engineering but are restricted by poor mechanical properties and non-tunable degradation (Prabhath et al., 2018; Tong et al., 2020). Therefore, synthetic polymers with favorable mechanical properties, simple fabrication methods and predictable degradation have been used as alternatives. However, synthetic polymers suffer from low cell affinity and require modification by biomolecules to improve their bioactivity (Makris et al., 2011; da Silva Morais et al., 2020). Moreover, the biodegradation of polymers is of the utmost importance since the degradation rate needs to be tuned in accordance with the required initial mechanical support, sustained drug delivery and space for neotissue formation (Engineer et al., 2011; Prabhath et al., 2018). In addition, numerous studies have been conducted to investigate the therapeutic effects of hybrid polymeric scaffolds, which combine the advantages of two or more natural and synthetic polymers, enabling the realization of comprehensive defined biophysical properties and bioactivity.
Natural Polymers
Natural polymers, such as polysaccharides and proteins, are considered to have great potential for meniscal tissue engineering due to their excellent biocompatibility, processability and bioactivity (Murphy et al., 2018). These polymers are also characterized by some drawbacks, including limited tunability, uncontrollable degradation, undesirable immunogenicity and poor mechanical properties, and are thus susceptible to failure in meniscal repair and regeneration (Chocholata et al., 2019; Li et al., 2020a; Table 3).
Proteins
Collagen is the most prevalent component of the meniscus ECM (Murphy et al., 2018). In general, it has a triple-helix structure, forms a highly organized 3D architecture, and plays a crucial role in maintaining the biological and structural integrity of the ECM (Cen et al., 2008; da Silva Morais et al., 2020). Since collagen is the main component of hard tissues and fibrous tissue and has excellent biocompatibility and degradability and low antigenicity, a large number of tissue engineering studies have utilized it in scaffolds for orthopedic applications, such as those in bone (Sarkar et al., 2006), cartilage (Zhang et al., 2013), tendons (Caliari et al., 2011), and intervertebral discs (Wilke et al., 2006). Collagen is widely used in the construction of meniscal cartilage tissue engineering scaffolds in various manufacturing methods. Recently, Filardo et al. (2019) used magnetic resonance imaging (MRI) and 3D bioprinting technology to design and create a cell-laden, collagen-rich and bioengineered medial meniscal tissue model, which could help optimize the custom design of damaged meniscus implants. The application of collagen in electrospun scaffolds has also been investigated for meniscal regeneration. Baek et al. (2018) produced a multilayer structure consisting of a collagen type I scaffold and tricomponent gel (collagen type II, chondroitin, hyaluronan) loaded with different types of cells. This electrospun collagen scaffold was reported to be able to promote cell adhesion and proliferation and meniscus-like extracellular matrix secretion. With regard to clinical use, Marcheggiani Muccioli et al. (2020) recently presented a 10-year follow-up study on soccer players who had received arthroscopically implanted lateral collagen meniscus implants (CMIs). The results showed promising recovery of knee joint function, and obvious cartilage thinning was not observed on imaging (Marcheggiani Muccioli et al., 2020).
However, collagen scaffolds displayed poor mechanical properties and much faster degradation rates than scaffolds consisting of polysaccharides and synthetic polymers; thus, the combination of collagen with other natural polymers and biomolecules was investigated (Subbiah and Guldberg, 2019; da Silva Morais et al., 2020). For example, a collagen/hyaluronan-infused, 3D-printed polymeric scaffold for partial meniscus replacement showed enhanced mechanical properties that could simultaneously satisfy the requirements for resistance to axial compression and circumferential tension (Ghodbane et al., 2019).
As a modified and degraded form of collagen, gelatin is a natural polymer derived from the hydrolysis of animal collagen (Santoro et al., 2014; Aoki and Saito, 2020). Since the digestive process enables gelatin to lose the triple-helix structure of collagen and confers low antigenicity, high biocompatibility and convenient fabrication, it is widely used in tissue engineering (Van Den Bulcke et al., 2000; Hoque et al., 2015). Narita et al. (2012) incorporated fibroblast growth factor 2 (FGF-2) into a gelatin hydrogel and observed an increased meniscal cell density after its application in horizontal meniscal tears in rabbits. In another study, rabbit platelet-rich plasma (PRP) was impregnated into a freeze-dried gelatin hydrogel to repair a circular meniscal defect in the anterior portion of the inner zone. The results showed that PRP strongly enhanced the healing process of the avascular meniscal zone (Ishida et al., 2007). However, the disadvantages of rapid degradation and dissatisfactory mechanical properties limit the application of gelatin alone in meniscal regeneration. Correspondingly, gelatin could be functionalized with methacrylamide (GelMA) groups to enable photocrosslinking by UV, potentially with the assistance of photoinitiators (Van Den Bulcke et al., 2000). Thus, gelatin modified with GelMA has also been studied as a polymeric meniscal scaffold. A study showed that GelMA in the construct significantly enhanced the adhesion of chondrocytes and the secretion of type II collagen (Bahcecioglu et al., 2019, 2019a).
Silk is a natural protein fiber produced by insect larvae for cocoons, and Bombyx mori silkworm cocoons are the most predominant source of silk (Murphy et al., 2018; Kashirina et al., 2019). The silkworm cocoon is mainly composed of silk sericin (SS) and SF, and the latter possesses impressive mechanical properties, elasticity, favorable biocompatibility, low immunogenicity and predictable biodegradability (Altman et al., 2003; Guziewicz et al., 2011; Kashirina et al., 2019). SF sponges have been found to enhance energy absorption and protect chondrocytes due to their favorable elasticity and low interfacial shear force (Li et al., 2020d). A silk-based platform has already been used as a substitute for meniscectomy. Yan and coworkers optimized the combination of a silk sponge and collagen coating in a rabbit meniscectomy model by applying coated collagen internally and externally to enhance the biocompatibility and initial frictional properties, respectively. Silk-collagen composites induced the formation of more meniscus-like tissue and reduced cartilage wear (Yan et al., 2019). More recently, a PCL/SF/Sr2+ scaffold for total meniscal repair, whereby the scaffold was manufactured by 3D wet electrospinning, showed enhanced meniscal regeneration. The structural components and mechanical properties of the neomeniscus almost rivaled those of the native meniscus 6 months after implantation (Li et al., 2020b). Concerning the necessity of stable scaffold fixation to the meniscus, a study conducted by Cengiz proposed a highly interconnected, suturable scaffold composed of SF and 3D-printed poly(ε-caprolactone) (PCL) mesh. This composite porous scaffold improved the suture retention strength by up to 4-fold and exhibited favorable tissue infiltration and blood vessel invasion after subcutaneous implantation in vivo (Cengiz et al., 2019).
Polysaccharides
Among natural polymers, agarose represents a natural and neutral, transparent polysaccharide that has excellent water solubility, biocompatibility, tunable mechanical properties and controllable self-gelation properties (Salati et al., 2020a) and plays an important role in the inner region in meniscal tissue repair (Bahcecioglu et al., 2019, 2019a,b). Experiments have shown attractive GAG expression in the agarose-impregnated interior meniscal region. Dynamic compression under 10% strain was also confirmed to increase GAG production in agarose (Bahcecioglu et al., 2019,b). A mixture of agarose and GelMA hydrogel was found to induce aggrecan expression and produce a high ratio of collagen type II/collagen type I in human fibrochondrocyte-hydrogel constructs. Moreover, the construct consisting of the blended hydrogel combined with the PCL scaffold perfectly mimicked the natural meniscal interior region (Bahcecioglu et al., 2019).
Hyaluronic acid (HA) is a natural hydrophilic GAG that is found in connective tissue, such as cartilage ECM, and is especially abundant in synovial fluid (Zamboni et al., 2018; Shah et al., 2019). HA is capable of water absorption and retention and lubrication and is an ideal molecule for promoting cartilage formation (Kim et al., 2011a; Zhang et al., 2014). In addition, functional groups can be introduced to the backbone of HA to mediate the formation of crosslinked hydrogels (Collins and Birkinshaw, 2013). For example, Song et al. (2019) successfully fabricated a crosslinked methacrylated hyaluronic acid (MeHA) fibrous scaffold by methacrylate modification, followed by an electrospinning process. The soft and stiff fibrous mesh network was sandwiched between meniscal tissue, and subcutaneous implantation in athymic rats showed that the stiffer MeHA fibrous network exhibited more obvious cellular invasion and enhanced collagen deposition (Song et al., 2019). In addition, Murakami et al. (2019) evaluated the effects of HA on human inner and outer meniscal cells and found that cell migration and proliferation were both accelerated by HA in a concentration-dependent manner. This finding suggested the possibility of meniscal regeneration without the need for growth factors, as HA alone could inhibit apoptosis and promote cell migration and proliferation.
Alginate, obtained from brown algae, is an anionic polysaccharide that exhibits remarkably good scaffold-forming properties and is biocompatible, inexpensive and abundant (Lee and Mooney, 2012). Furthermore, alginate hydrogels can be crosslinked by various materials (e.g., Ca2+) for bioactive agents and cell delivery (Lee and Mooney, 2012; Venkatesan et al., 2015; Kim et al., 2019). By combining collagen, alginate (A) and oxidized alginate (ADA), Gupta’s group designed self-healing interpenetrating network (IPN) hydrogel-loaded scaffolds with dual crosslinking [Ca2+-based ionic crosslinking and Schiff base reaction crosslinking (A-A, A-ADA)] capabilities, which revealed great potential for supporting fibrochondrocyte behavior and chondrogenesis in vitro (Gupta et al., 2020a). Alginate has also been used in minimally invasive meniscal tissue engineering applications. For example, Kim et al. (2019) fabricated an ultrapurified alginate (UPAL) gel that was dicationically crosslinked by CaCl2 and injected into rabbit meniscal defects. The reparative tissues in the UPAL gel group had a mean stiffness of 27.8 ± 6.2 N/mm, which was significantly greater than that in the control group at 12 weeks (Kim et al., 2019). Other researchers have often used alginate in combination with other polysaccharides. Recently, Resmi et al. (2020) synthesized an injectable, self-crosslinking hydrogel from alginate dialdehyde and gelatin, and ex vivo application of this hydrogel in pig meniscal tears showed good integration with host meniscal tissue.
In contrast to alginate, chitosan is a linear, positively charged copolymer derived from deacetylated chitin, which can be found in the exoskeleton of fungal cell walls (Shah et al., 2019; Donnaloja et al., 2020). Chitosan has been extensively used in skin (Sandri et al., 2019), bone (Cui et al., 2019), cartilage (Izzo et al., 2019), and tendon (Depres-Tremblay et al., 2019) regeneration, as it demonstrates excellent biodegradability, and it is worth noting that the degradation rate can be regulated by the molecular mass and deacetylation degree (Vårum et al., 1997; Mi et al., 2002). A decellularized meniscal extracellular matrix (DMECM) and gelatin/chitosan (G/C) composite scaffold with a high elastic modulus and low cytotoxicity was reported by Yu et al. (2019). Chitosan has also been freeze dried in the fabrication of porous scaffolds for cell transplantation and tissue regeneration (Suh and Matthew, 2000). To further investigate the impact of the molar content of chitosan on the chondrogenic potential of mesenchymal stem cells (MSCs), a comparative study was performed. Chitosan (Ch) at different molar ratios was crosslinked with polyvinyl alcohol (PVA) using urethane prepolymer (PPU) chains, and articular chondrocytes (ACs) and adipose tissue-derived mesenchymal stem cells (ASCs) were then isolated and cultured. The authors confirmed that the AC-seeded PVA/Ch/PPU (1:4:1) scaffold showed higher expression levels of ECM components, superior meniscus regeneration and lower levels of cartilage degeneration than the comparator scaffolds (Moradi et al., 2017b).
Synthetic Polymers
Owing to their poor mechanical strength, unstable degradation and limited sources, natural polymers are still insufficient for meniscal repair and regeneration. Therefore, synthetic polymers with favorable mechanical properties, reproducibility and controllable degradation have been widely used to produce meniscal scaffolds (Makris et al., 2011; da Silva Morais et al., 2020; Table 3). However, one of the limitations of synthetic scaffolds is their paucity of bioactive cues, which could be overcome by adding biological coatings (Silva et al., 2020).
As a biodegradable polymer with excellent biocompatibility and mechanical strength, poly(glycolic acid) (PGA) has been widely used in the biomechanical and medical fields since the 1970s and can serve as a scaffolding material to repair articular cartilage in clinical practice (Siclari et al., 2018; Otsuki et al., 2019; Cojocaru et al., 2020). In meniscal tissue engineering, a 3D, meniscal-like, PGA-hyaluronan implant with high porosity was developed, and this scaffold showed high biocompatibility and improved the expression of chondrogenic genes during coculture with human meniscal cells in vitro. In addition, in a sheep model, the scaffold showed greater proteoglycan and collagen type I production than the control group (Cojocaru et al., 2020). For in vivo evaluation, a meniscus-shaped scaffold made of PGA covered with a polylactic acid/caprolactone [P(LA/CL)] sponge was implanted into the right knee in a medial meniscus resection minipig model. The results showed that the scaffold provided appropriate initial strength and could prevent cartilage degeneration with relatively low inflammation. However, the compressive stress and elastic modulus of the scaffold were significantly inferior to those of the native meniscus (Otsuki et al., 2019).
Poly(lactic acid) (PLA) is a thermoplastic aliphatic polyester obtained from the polymerization of lactic acid and/or the ring-opening polymerization of lactide, which has suitable biocompatibility and biodegradability but also some drawbacks, such as a high cost, long degradation time, low utility and limited molecular weight (Castro-Aguirre et al., 2016; Murariu and Dubois, 2016). In an interesting study, core-shell coaxial nanofibrous scaffolds were prepared by electrospinning. The PLA core provided mechanical strength, while the collagen shell facilitated cellular adhesion and matrix synthesis. In vivo experiments showed excellent integration between the scaffold and native tissue (Baek et al., 2019). The filamentous PLA structure was printed by 3D printing and modified by surface modification with active functional groups. Then, IPN hydrogels populated with hMSCs were applied to the surface-modified PLA structure for in vitro and in vivo research. At 28 days after implantation in a rat model, the structure of PLA remained relatively intact, which was consistent with the degradation curve in vitro. The integrity of the PLA scaffold ensured minimal mechanical stress on the hMSCs, allowing optimal function to be achieved (Gupta et al., 2020a).
Compared with that of PLA, the degradation time of poly(lactic-co-glycolic acid) (PLGA) can be controlled according to the glycolic acid content, while the higher the ethyl ester ratio is, the easier the polymer is to degrade (Zhou et al., 2012). PLGA is a linear copolymer composed of different proportions of glycolic acid and lactic acid monomers (Gentile et al., 2014). PLGA is an attractive polymer used in drug delivery and tissue engineering due to its favorable biodegradability, flexible processability, tunable degradation, surface functionalization and targeted drug delivery (Danhier et al., 2012; Mir et al., 2017; Zhao et al., 2021). PLGA is biodegradable because its ester linkages can be hydrolyzed in aqueous solution, and as byproducts, glycolic acid and lactic acid can be cleared from the body via normal metabolic pathways (Lü et al., 2009). Gu et al. (2012) used cartilage-derived morphogenetic protein-2 (CDMP-2) and TGF-β1 to preculture autologous myoblasts and then construct myoblast cell-seeded PLGA scaffolds, which presented accelerated healing after meniscal defect implantation. Regarding functionalized PLGA scaffolds, Kwak et al. (2017) reported a PRP-pretreated PLGA mesh scaffold seeded with human ACs to regenerate meniscal tissue. At 6 weeks after subcutaneous implantation, increased cell attachment and cartilaginous tissue formation were observed in the cell-seeded scaffold between native devitalized meniscal discs (Kwak et al., 2017). Other researchers have developed several PLGA-based approaches as drug delivery platforms. For instance, TGF-β3 and connective tissue growth factor (CTGF) loaded in PLGA microparticles were incorporated into 3D-printed PCL anatomical meniscal scaffolds and demonstrated promising reparative results (Lee et al., 2014; Figure 3).
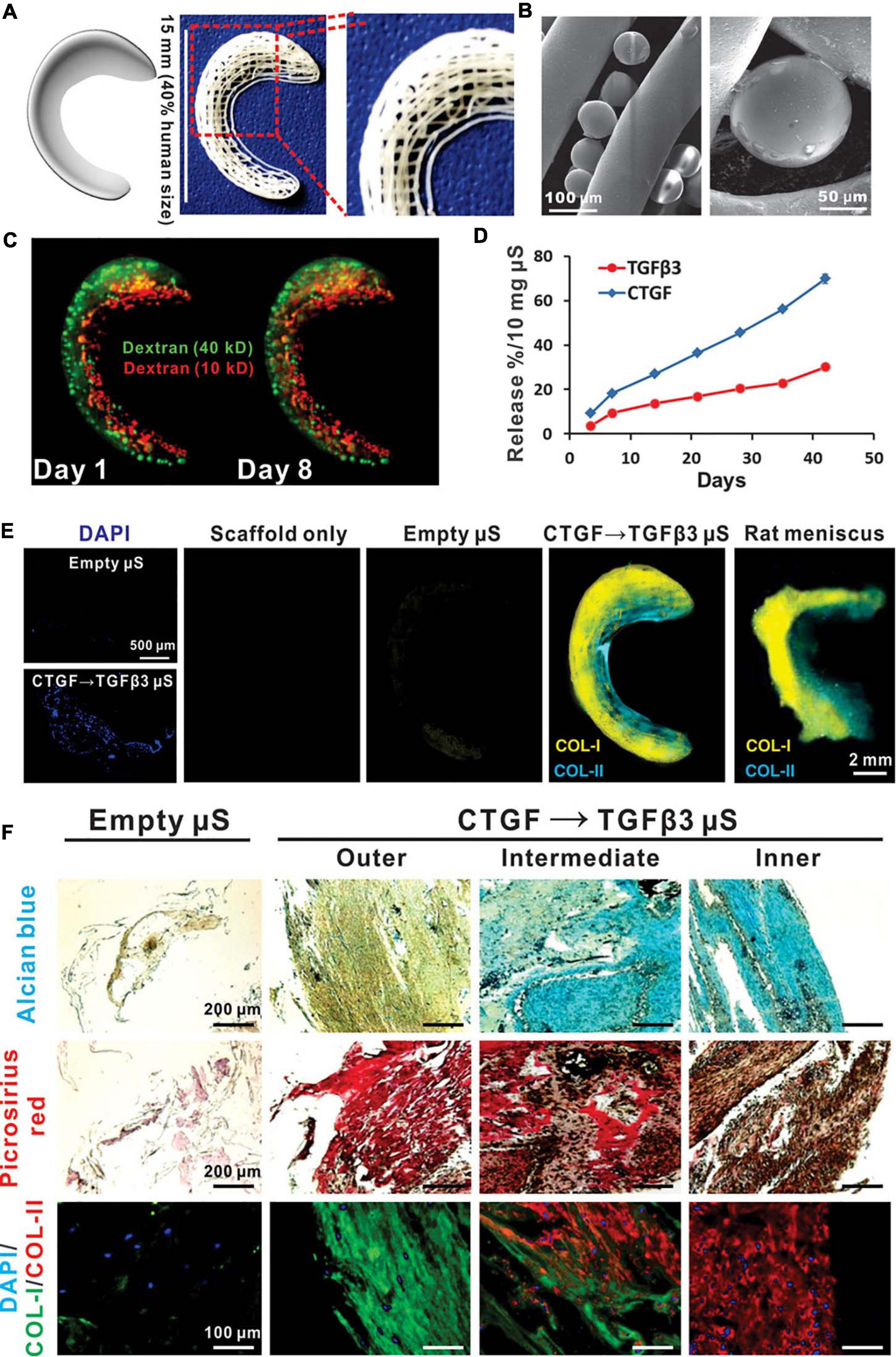
Figure 3. Spatiotemporally released rhCTGF and rhTGF-β3 induced fibrocartilage-like matrix formation in 3D-printed, porous scaffolds. (A) Anatomical reconstruction of human meniscus. Human meniscal scaffolds were 3D printed with the layer-by-layer deposition of PCL fibers (100 mm in diameter), forming 100- to 200-mm channels. (B) PLGA microspheres (μS) encapsulating rhCTGF and rhTGF-β3 were in physical contact with PCL microfibers. (C) Fluorescent dextran simulating CTGF (green, 40 kD) and TGF-β3 (red, 10 kD) was delivered into the outer and inner zones, respectively, of human meniscal scaffolds to show scaffold loading. The distribution of dextran was maintained from day 1 to day 8. (D) rhCTGF and rhTGF-β3 release from the PCL scaffolds over time in vitro. (E) When the scaffolds were incubated atop human synovial MSC monolayers for 6 weeks, spatiotemporally delivered rhCTGF- and rhTGF-β3 induced cells to form zone-specific collagen type I and II matrices, similar to those in the native rat meniscus. (F) Scaffolds with empty mS showed little matrix formation after 6 weeks of coculture with a 1:1 mixture of fibrogenic and chondrogenic supplements (no growth factors in medium). Spatiotemporal delivery of rhCTGF and rhTGF-β3 induced fibrocartilaginous matrix formation, consisting of alcian blue-positive, collagen II-rich cartilaginous matrix in the inner zone and picrosirius red-positive, collagen I-rich fibrous matrix in the outer zone. A total of five replicates were tested, with representative images selected from the same scaffold (reprinted from Lee et al., 2014 with permission from AAAS).
PCL is a hydrophobic polyester with a low melting point (56–61°C), slow degradation, favorable compatibility and satisfactory mechanical strength (Abedalwafa et al., 2013; Teng et al., 2014). However, its hydrophobicity and inadequate wettability may lead to poor cell attachment and proliferation (Mondal et al., 2016; Silva et al., 2020). For that reason, the surface modification of PCL is crucial for its biological application (Mondal et al., 2016). In one study, galactose was incorporated into electrospun PCL nanofibrous scaffolds for meniscal cell culture, whereas the composite scaffold resulted in increased cell attachment and proliferation (Gopinathan et al., 2015). In addition, PCL has been used to prepare nanofiber scaffolds with a high effective surface area-to-volume ratio to facilitate the release of biomolecules and ensure greater interaction between seeded cells and biomolecules (Qu et al., 2019). For example, Qu et al. (2019) developed an aligned protein-containing scaffold based on electrospun PCL-PLGA fibers using bovine serum albumin (BSA) to stabilize the loaded TGF-β3. Compared with the high-dose TGF-β3-loaded scaffold, the low-dose TGF-β3-loaded nanofiber scaffold effectively activated the fibrochondrogenic differentiation of synovium-derived stem cells (SDSCs). The results indicated that fibrochondrogenesis and chondrogenesis differed by growth factor concentration, with the former requiring a lower dose (Qu et al., 2019).
Polyurethane (PU) possesses elasticity, thermoplasticity and excellent biocompatibility and has already been applied in meniscal tissue engineering (Venkatesan and Kim, 2014; Koch et al., 2018; Vedicherla et al., 2018; Bharadwaz and Jayasuriya, 2020). For example, Actifit® implants have been studied as a cellular component delivery vehicle. Vedicherla et al. (2018) developed a cell-seeded PU scaffold. Fresh chondrocytes (FCs) and minced cartilage (MC) were cultured on the scaffold, and the tissue integration effect was evaluated in a caprine meniscal explant model. The results exhibited better matrix deposition and tissue integration in both the FC and MC groups than in the acellular scaffold group (Vedicherla et al., 2018). MSCs are also promising for meniscal repair due to their potential for fibrochondrogenesis and their ability to secrete reparative growth factors (Koch et al., 2018). An MSC-loaded PU scaffold was produced as a replacement for large, full-thickness meniscal defects. At 12 weeks after surgery, the vessel density in the scaffold group was superior to that in the cell-free groups. Additionally, significantly greater proteoglycan deposition and integration with the surrounding meniscal tissue were observed in the MSC-loaded group than in the acellular group. However, this advantage of MSC loading disappeared after 12 weeks (Koch et al., 2018). It has also been reported that MSC-seeded PU scaffolds exhibit little additional clinical benefit in the protection of articular cartilage (Olivos-Meza et al., 2019).
Another synthetic polymer that should be addressed is polycarbonate urethane (PCU). PCU is a flexible, biocompatible, biostable and wear-resistant material that can be incorporated in 3D-printed, porous structural scaffolds (Williams et al., 2015; Abar et al., 2020). In addition, as a hydrophilic material, PCU can mimic the lubrication mechanism in native synovial joints (Wan et al., 2020). A medial meniscus PCU prosthesis, called NUsurface® (Active Implants Corp., Memphis, TN, United States), has been undergoing clinical trials and has become available on the market (Drobnič et al., 2019). Another novel meniscus-shaped, wear-resistant full implant made of PCU was also developed. The study showed that the posterior horn of the implant was under maximum pressure at 3 months, and the deformation at 12 months after implantation was acceptable. However, one implant failed due to a complete tear during posterior angular extension. Therefore, it is essential to strengthen the posterior horn of the implant to prevent fixation failure of one horn under extension. The damage progression in the implant group was similar to that in the allograft group but significantly worse than that in the non-operated group (Vrancken et al., 2017).
PVA is a polymer synthesized from partially or completely hydroxylated polyvinyl acetate (Baker et al., 2012). As a bioinert, non-carcinogenic, moist, biocompatible composite material (Hayes and Kennedy, 2016; Marrella et al., 2018), PVA possesses good formability, mechanical properties and manufacturability (Kobayashi et al., 2005; Moradi et al., 2017a; Marrella et al., 2018) and has been widely used in the field of regenerative tissue engineering. Polyvinyl alcohol hydrogel (PVA-H) has viscoelastic properties similar to those of cartilage and meniscal cartilage and does not wear out even after millions of compression cycles (Moradi et al., 2017a). As early as 2005, Kobayashi et al. (2005) developed an artificial meniscus with high-water-content (90%) PVA-H and conducted a preliminary study in a rabbit model. This study showed that the articular cartilage of the knee was still in good condition 2 years after the operation (Kobayashi et al., 2005). As a physically crosslinked gel, PVA-H does not contain toxic monomers that may be present after chemical crosslinking. However, its poor tensile properties limit its practical use, especially in strong fibrous tissues, such as the meniscus, tendons and ligaments (Holloway et al., 2010, 2014). Therefore, it is particularly important to modify PVA-H with new materials. A 3D biomimetic meniscal scaffold was designed using 3:1 SF/PVA. Autoclaved eggshell membrane (AESM) powder (1–3% w/v) was used as a biomechanical enhancer, and the composite scaffold presented with good load-bearing performance and improved meniscal tissue regeneration (Pillai et al., 2018).
In addition, various copolymers based on poly(ethylene oxide) (PEO) have been developed and applied in the field of drug delivery due to their good biocompatibility and fast, non-toxic degradation (Kim et al., 2014). PEO can be used as a sacrificial, water-soluble scaffold material to increase porosity and promote cell infiltration (Baker et al., 2008). As Qu’s group reported, collagenase-loaded PEO electrospun fibers may trigger matrix degradation. When applied in meniscal tears in vitro, PEO degradation was accompanied by the release of enzymes in the local wound edge and successfully increased both tissue porosity and cell migration (Qu et al., 2013).
Decellularized Materials
The decellularization method has historically been used to isolate the ECM via cell removal and is widely applied in tissue regeneration (Gilbert et al., 2006). Due to their meniscus-specific chemical composition and architecture, implants derived from decellularized materials have been used as scaffolds in meniscal tissue engineering (Murphy et al., 2018; Ruprecht et al., 2019). Recently, decellularized materials have been explored for use in decellularized meniscal scaffolds (DMSs) to support the regeneration of meniscal tissue. For instance, Stabile et al. (2010) developed fresh-frozen meniscus allografts with increased porosity and promising mechanical integrity that presented potential for clinical application. DMECM presents components similar to those of the native meniscus and can be formed into various structures, thereby promoting cell infiltration and remodeling (Chen M. et al., 2019; Ruprecht et al., 2019). Researchers have demonstrated that meniscus-derived matrix scaffolds are capable of promoting the infiltration of endogenous meniscal cells and MSCs (Ruprecht et al., 2019). Although numerous studies have proven that decellularized matrix (DCM) may be able to regulate stem cell differentiation, Liang et al. (2018) demonstrated that synovial fluid-derived mesenchymal stem cell (SF-MSC)-loaded meniscus-derived DCM was incapable of inducing the differentiation of SF-MSCs into MFCs without TGF-β3 and IGF-1 supplementation. However, DCM materials suffer from poor performance in load-bearing applications. To tackle this problem, our team previously developed a hybrid scaffold for regenerating the meniscus in a rabbit model, combining acellular meniscus extracellular matrix (AMECM) and demineralized cancellous bone (DCB). The AMECM/DCB constructs demonstrated favorable mechanical properties and a promising capacity to promote fibrochondrocyte proliferation and GAG secretion (Yuan et al., 2016). Another common solution to improve the stiffness of implants is to hybridize them with other synthetic polymers. For example, Guo and coworkers used DMECM and a 3D-printed PCL scaffold to create a hybrid construct for rabbit meniscal regeneration. The hybrid scaffold displayed biomechanical strength similar to that of the native meniscus and facilitated whole meniscal regeneration in both rabbit and sheep meniscus repair models (Guo et al., 2021). Additionally, as another tissue engineering approach, DMECM-based injectable hydrogels have been developed. One early work by Yuan et al. (2017) developed hMSC-loaded DMECM hydrogels and found that the cell-laden DMECM hydrogel successfully retained the viability of hMSCs in nude rat meniscal injury for 8 weeks, resulting in neomeniscal tissue formation and preventing joint space narrowing, pathological mineralization and OA development.
Hybrid Polymeric Scaffold for Meniscal Tissue Engineering
Recently, researchers have fabricated numerous hybrid scaffolds made from two or more types of polymeric materials. While a single natural or synthetic polymer can only provide limited advantages for tissue-engineered meniscal scaffolds, the final product produced by a mixture of natural and synthetic polymers tends to possess comprehensive advantages that no single polymer can provide (Bakhshandeh et al., 2017). For example, natural polymers, such as chitosan, collagen and gelatin, usually contain biological molecules that can interact with cells, providing superior biological performance for hybrid polymeric scaffolds (Donnaloja et al., 2020), while synthetic polymers provide tunable physical properties such as mechanical support and a controllable degradation rate (Zhou et al., 2012; Figure 4).
Loading hybrid scaffolds with tissue-derived cells has the advantage of the encapuslated cells replenishing ECM loss and filling in defects as scaffold degradation occurs over time (Bilgen et al., 2018). For example, Chen M. et al. (2019) constructed a wedge-like, 3D-printed, MFC-loaded hybrid scaffold with a PCL scaffold as a backbone and then injected an optimized MECM-based Ca-alginate hydrogel (2%). In vivo experiments confirmed that the PCL-hydrogel-MFC group was similar to the sham group in terms of biochemical content, histological structures and biomechanical properties, which demonstrated an ideal capability for regeneration of the whole meniscus (Chen M. et al., 2019). In another study, by Cengiz et al. (2020), to meet the requirements of biomimetic meniscal scaffolds and cell coculture, PCL was blended with SF and entrapped in a 3D-printed cage (EiC) scaffold. Human stem cells or meniscocytes were cultured on the EiC scaffold and implanted subcutaneously in nude mice. The SF-based EiC scaffold showed better cell infiltration as well as a milder inflammatory response (Cengiz et al., 2020).
On the other hand, hybrid scaffolds combining polymers and biofactors can locally deliver signal molecules to create a favorable microenvironment. For instance, a PCL/SF hybrid scaffold based on 3D printing was developed by Li et al. (2020d) and exhibited a balance between the mechanical properties and degradation rate. The SF sponge provided cartilage protection due to its high elasticity and low interfacial shear force, the PCL provided excellent mechanical support, and the conjugation of a peptide with SMSC-specific affinity (LTHPRWP; L7) increased cellular recruitment and retention. In vivo experiments showed that this meticulously tailored scaffold greatly enhanced meniscal regeneration while protecting cartilage (Li et al., 2020d). In summary, hybrid scaffolds have been used for meniscal tissue engineering. The general design strategy consists of utilizing synthetic polymers as a supporting framework, with natural polymers more likely serving as an additive microenvironment to mimic extracellular microenvironments, while cells and bioactive factors may further assist in improving cell recruitment, proliferation, and differentiation and ultimately improve regeneration.
Recent Advancements
3D Printing
Promising advances have been made in technology for the fabrication of meniscal scaffolds in terms of particulate leaching, freeze drying, solvent casting, electrospinning, and 3D printing (Esposito et al., 2013; Sun et al., 2017; Li et al., 2020e). Table 4 is a summary of the most relevant examples of meniscal scaffold fabrication techniques. Currently, in the fabrication of sponge scaffolds using physical and/or chemical treatments, the porous scaffold can provide a desirable microenvironment for the cells culturing, however, the pore size, porosity and interconnectivity cannot be controlled, and these scaffolds are often hindered by pore blockage effects in vivo (Sun et al., 2017). Another alternative manufacturing technology, electrospinning technology can be used to produce collagen-mimicking nanoscale fibers but is also limited in terms of controlling the structure and porosity of the construct (Megelski et al., 2002; Sun et al., 2017).
As an emerging additive manufacturing technology, 3D printing is able to produce components with ideal shapes and structures, allowing the accurate construction of specified 3D hierarchical structures (Bahcecioglu et al., 2019; Fu et al., 2020). Hence, among all of these scaffolding technologies, 3D printing is more promising in meniscal tissue engineering. Indeed, 3D bioprinting is a branch of the development of 3D printing technology and represents the technology’s entry into the field of tissue engineering and regenerative medicine (Matai et al., 2020). 3D bioprinting is an automated, tissue-friendly manufacturing method that very accurately simulates the actual arrangement of the cells and ECM of the targeted tissue, with the ability to construct the 3D tissue equivalent of the desired shape and structure, reproducing the complexity of human tissue (Murphy et al., 2018; Chae et al., 2021). Generally, bioprinting technologies mainly applied in meniscus/cartilage tissue engineering can be classified as follows: 3D plotting/direct ink writing, stereolithography (SLA), selective laser sintering (SLS), fused deposition modeling (FDM), and extrusion-based bioprinting (Moroni et al., 2018). Table 5 presents several commonly used bioprinting techniques and provides some comparative information. At present, there are many studies using FDM to produce a PCL framework and then combining hydrogels to fabricate composite meniscal scaffolds. However, FDM techniques suffer from poor surface quality and difficulties in combining biopolymers owing to their high extrusion temperatures (Agarwal et al., 2021). The SLA technique is characterized by high resolution; however, a limited selection of photopolymers restricts its application in tissue engineering, and it has not yet been applied in meniscal tissue engineering (Qu et al., 2021). Tissue-engineered meniscal scaffolds produced by extrusion bioprinting techniques have high yields and excellent structural integrity, and this technique is the most common 3D bioprinting technology in the field of meniscal regeneration.
From a polymeric materials science point of view, numerous polymers have been extensively investigated to serve as bioinks in 3D printing for tissue engineering. Bioinks, mainly hydrogels that contain cells and various biological components, play an important role in creating a compatible microenvironment for cellular activity (Roseti et al., 2018; Samadian et al., 2020). Some single-component polymer bioinks, such as SF (Bandyopadhyay and Mandal, 2019), alginate (Narayanan et al., 2018), and GelMA (Grogan et al., 2013) bioinks, have been used for 3D meniscal bioprinting. However, a single polymer cannot reproduce the complexity of ECM in natural tissue and thereby provide a good microenvironment for cells in vivo. Researchers have made immense efforts to develop an optimal bioink that simultaneously meets the requirements of biocompatibility and printability. Recently, tissue-specific meniscal dECM (me-dECM) bioinks based on 3D cell printing were designed by Chae et al. (2021) and helped preserve the complexity of the natural ECM, thus demonstrating the potential for tissue regeneration and special biological functions. This printable bioink supported the proliferation and differentiation of encapsulated stem cells, and induced fibrochondrocytes were also observed in vitro (Chae et al., 2021). In addition to combining hydrogels and solid polymers to mimic the biphasic structure of the meniscus and produce an optimal cellular microenvironment, 3D bioprinting has also been used to incorporate growth factors and cells in hydrogel-based scaffolds (Caterson et al., 2001; Sun et al., 2017). In a pilot in vivo study performed by Sun et al. (2020), a ready-to-implant anisotropic meniscal scaffold fabricated by 3D bioprinting was implanted in a goat meniscus transplantation model. This 3D-bioprinted meniscus substitute contained microspheres encapsulating CTGF and TGF-β3 and was mixed with BMSCs. The in vitro and in vivo results demonstrated cell phenotypes and matrix formation resembling those of the native meniscus, as well as chondroprotection of the goat articular cartilage (Sun et al., 2020).
In addition to the implications of the aforementioned traditional bioprinting techniques for the engineering of meniscus substitutes, some advanced techniques have also generated enthusiasm about their potential applications in meniscal tissue engineering. For example, the inkjet technique, which is suitable for printing cellularized scaffolds, can be used to print customized cellularized menisci. The process of inkjet printing usually consists of two parts: droplet formation on the target substrate and droplet interaction with related materials (Li et al., 2020c). Recently, in a proof-of-concept study, a cellularized human meniscus was produced by a microvalve-based inkjet technique. Primary human bone marrow mesenchymal stem cells were isolated, embedded with collagen bioink and customized inkjet printing, resulting in a patient-specific meniscal scaffold with superior anatomical structure and favorable cell compatibility (Filardo et al., 2019). In addition, some new bioprinting technologies based on non-contact (acoustoelectric, optical and magnetic) guidance of cell chemotaxis are gradually coming into play. Traditional scaffold technology guides the migration, adhesion and orientation of cells through the conformation of its own scaffold fibers and controls the expression of extracellular matrix according to the orientation of the fibers. A new acoustic electrophoretic three-dimensional (3D) biomanufacturing method, which uses radiation forces generated by superimposing ultrasonic bulk acoustic waves (BAWs) to preferentially organize arrays of cells in monolayer and multilayer hydrogel structures, was developed. The researchers investigated the effects of the parameters on the cell array configuration by controlling the frequency and amplitude of the ultrasonic wave, the signal voltage, the viscosity of the bioink, and the time of action. Finally, a physiologically related 3D construction of the meniscus was presented (Chansoria et al., 2019).
In conclusion, the excellent ability of 3D bioprinting technology to precisely control the fiber diameter, orientation, and microarchitecture endows the resulting constructs with promising mechanical properties and favorable biological functions. Therefore, it is one of the most attractive and promising technologies for tissue engineering applications, particularly meniscal regeneration.
Meniscal Zonal Reconstruction Studies
Restoration the heterogeneities in anatomical, structural, mechanical and biochemical characteristics of meniscus is still a challenging goal albeit significant advances achieved in biomaterials science. Meniscal regeneration involves the two distinct regions that with different composition, structures and function. Hence, it requires an in-depth understanding of the zonal difference within meniscus and thus design such an optimal scaffold possessed with the anisotropic variation of (i) biomaterial composition, (ii) microarchitectures, and (iii) regional-specific pro-regenerative effects.
In this context, recent 3D fabrication techniques which can construct zone-dependent features, combined with polymeric biomaterials, have shed light on this topic. Firstly, biomimicry of meniscal spatial variation can be achieved by varying scaffolding materials. For example, in a study by Bahcecioglu et al. (2019), anatomically shaped PCL meniscal scaffolds were impregnated with cell-laden GelMA-agarose in the inner region and GelMA in the outer region. The in vitro results showed that inner GelMA-agarose hydrogel enhance chondrogenic differentiation, while the increasing COL I and decreasing COL II expression in the outer region was observed (Bahcecioglu et al., 2019). This structurally and biochemically correct scaffold presented with an advantageous solution on meniscus zonal reconstruction. Anatomical selected meniscus ECM were also exhibiting potential to develop anisotropic scaffolds able to induce the zonal fibrocartilage differentiation (Rothrauff et al., 2017). In addition to the biopolymers selections, the cell-loaded meniscal constructs in bioreactors is another approach to fulfill the zonal construction requirements. The application of bioreactors is commonly referred to applying controlled biophysical stimulus, especially valuable for hard tissue regeneration (Aguilar et al., 2019). Ideally, a zone-dependent mechanical stimulation in terms of tensile stimulus dominating on outer zone and compressive stimulus dominating on inner region can be a promising strategy for zonal differentiation (Puetzer and Bonassar, 2016). As an example, in Bahcecioglu et al. (2019a), fibrochondrocytes loaded in PCL scaffold were arranged to form scaffolds. The authors tested the effect of different cell-laden PCL/hydrogel using a custom-made bioreactor and compared the outcomes against static methods. The scaffolds treated with the dynamic stimulation resulted in increased ratio of COL II expression in the inner region (agarose impregnated along with compressive method), and enhanced ratio of COL I expression in the outer region (GelMA impregnated along with tensile method). From this instance, it is evident that bioreactor combined with spatial biopolymer incorporation enhance the formation of zone-specific tissues compared to the static seeding.
Furthermore, the region-specific infusion of various grow factors has also been explored for meniscal zonal reconstruction. For example, Lee et al. (2014) designed a 3D printed anatomic-mimicking PCL scaffold infused with different growth factors (Figure 3). The spatial-temporal releasing growth factors allowed dual induction of chondrogenic and fibrochondrogenic differentiation in the same construct (Lee et al., 2014). Another example is shown in Figure 5 where a dual growth factors functionalized scaffold was placed within a perfusion bioreactor (Zhang et al., 2019). The bioreactor was used to provide a consistent dynamic biomechanical stimulus to accelerate the spatial regulation of fibrochondrocyte differentiation. The authors used this biomechanical and biochemical combined strategy in an attempt to construct a physiological anisotropic meniscus. Their results showed that the cells expressed zonal-specific differences in the expression of the COL I and COL II, and also presented with a long-term chondroprotection of the knee joint when implanted into a rabbit model.
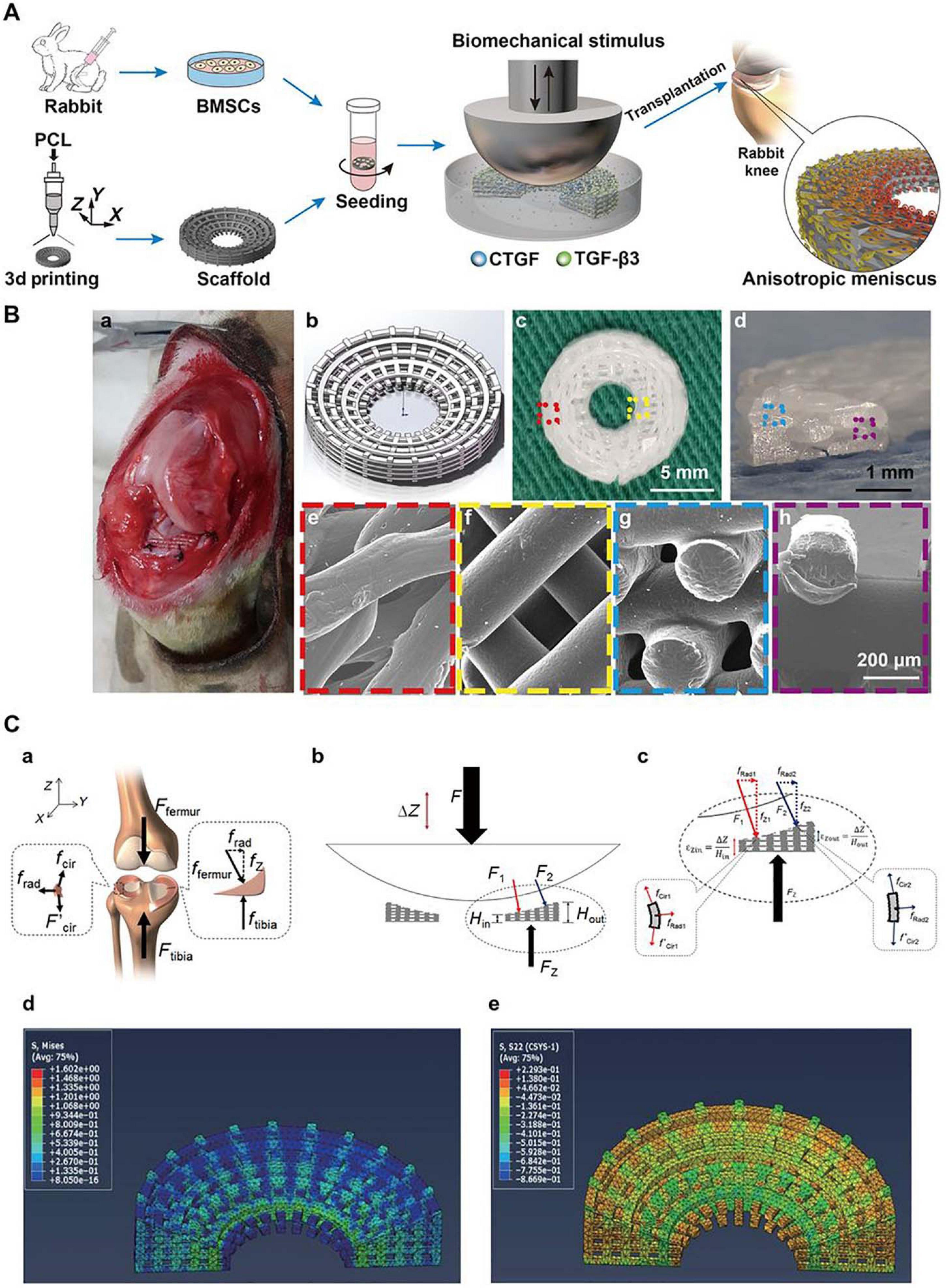
Figure 5. Schematic diagrams for the reconstruction of a functional anisotropic meniscus. (A) Flowchart of stem cell-based strategies for the construction of a tissue-engineered meniscus with an anisotropic structure. BMSCs, bone marrow-derived stem cells. (B) 3D-printed PCL scaffold for implantation. (a) Photograph of rabbit knee during implantation. (b) 3D scaffold design model. (c,d) Top and cross-sectional views of the wedge-shaped circular PCL scaffold. (e,f) Top views of the outer and inner regions of the PCL scaffold obtained by SEM. (g,h) Cross-sectional views of the outer and inner regions of the PCL scaffold obtained by SEM. (e–h) Higher magnification images of regions outlined in c and d. (C) Biomechanical simulation. (a) Forces typically transduced by the knee meniscus in the human body. (b,c) Applied loading forces across the meniscal construct. (d,e) Calculated stress fields across the meniscal construct at 10% displacement of the loading plate. (d) von Mises stress distribution with a gradual decrease in stress from the internal to external rings. (e) Compressive circumferential stress in the internal rings and tensile circumferential stress in the external rings (reprinted from Zhang et al., 2019 with permission from AAAS).
In short, relevant studies have brought hope on zonal meniscal reconstruction which indicate that more attempts should paid on biomaterials innovations, manufacturing technologies, biomechanical stimulation, and other novel techniques.
Immune Compatibility and Tunability Studies
Polymeric materials science of the past focused on biocompatibility, including foreign body reactions (FBRs), degradability, and toxicity. However, current studies are aimed at solving one hurdle in terms of tissue engineering, that is, immune compatibility. In general, more advanced polymeric scaffolds are needed not only to fulfill the criteria of the present studies but also to regulate both the innate and adaptive immune responses.
Synthetic polymers, such as polyesters, are frequently used as cellular frameworks to support neotissue formation (Lee et al., 2019; Patel et al., 2019). For a long time, the immune compatibility of synthetic polymers focused on reducing the unwanted effects of FBR, fibrotic encapsulation, and toxicity (Chung et al., 2017). Numerous studies have confirmed that FBR may be a consistent process involved in neutrophil infiltration, monocyte/macrophage influx, fibroblast-mediated collagen and capillary bed formation, and the systemic response may also contribute to this process (Chung et al., 2017). On the other hand, natural polymers are manipulated not to induce an FBR response but to be a primary promoter for the regenerative process. For example, Ji’s and Lei’s groups found that glycidyl methacrylate-modified thermosensitive hydroxypropyl chitin hydrogels polarize macrophages both in vitro and in vivo at the cartilage defect site to accelerate a pro-regenerative process (Ji et al., 2020). It is believed that the bioactive motifs within ECM or ECM-like biological polymers can directly modulate the immune response (Rowley et al., 2019). For instance, the fibronectin sites FNI10–12 and FNII1–5 have been demonstrated to be able to modulate cellular responses in the regenerative cascade (Sawicka et al., 2015).
Apart from the composition of polymers, the factors involved in the immune response to biomaterials require further investigation. Interestingly, Veiseh et al. (2015) proved that spherical polymers (≥1.5 mm) in diameter triggered more severe fibrotic reactions than smaller particles or other shaped constructs, which indicated that the geometry and dimension of biomaterials are also vital impact factors of the FBR response. Currently, polymeric scaffolds are emerging as orchestrators to modulate the immune response that can influence tissue regeneration and repair processes (Chung et al., 2017). Recent research on biomaterial-mediated immunomodulation has two main directions. First, we focused on the modification of engineering scaffolds. A variety of surface chemical features have been studied, including the functional groups, surface charge, hydrophilicity, and molecular weight of the compound (Lee et al., 2019). Delivering anti-inflammatory drugs or cytokines is a second direction for engineered scaffolds to stimulate immunomodulation responses. For example, decellularized cartilage extracellular matrix has been decorated with the anti-inflammatory cytokine IL-4, and this modified scaffold promoted M2 macrophage polarization and osteochondral repair in a rat model (Tian et al., 2021). In addition, acellular cartilage matrix scaffolds functionalized with Wharton’s jelly mesenchymal stem cell-derived exosomes successfully reduced the inflammatory response in the joint cavity of a rabbit osteochondral model and improved cell proliferation, migration and polarization in vitro.
In summary, in recent decades, various biomaterial-mediated meniscal tissue engineering strategies have mainly focused on modulating the activity of different cells involved in meniscal regeneration to induce meniscal tissue formation. However, more attention should be given to the topic of biomaterial design to modulate the immune response and avoid undesirable inflammatory responses (Lee et al., 2019). To date, there are no systematic studies on the impact of immunomodulatory biomaterials on meniscal regeneration. We believe that this kind of engineered scaffold could have a profound impact on meniscal regeneration and associated patient recovery.
Challenges and Future Prospects
Our increasing understanding of the crucial functions of the meniscus in knee homeostasis propels clinicians and scientists to treat meniscal tears with more promising strategies. A successful regenerative strategy for meniscal tissue engineering requires a profound understanding of the structure and composition of native menisci. First, the investigation and reconstruction of the structure-composition-function relationship remains a primary challenge of meniscal regeneration. The capability of the meniscus to withstand mechanical functions in terms of resistance to tension, compression and shear stress is mostly attributed to the collagen-proteoglycan meniscal matrix; however, complete reconstruction of this structure has not yet been attained. With decades of development of biomaterial science, a range of polymers spanning from natural to synthetic have been optimized in the search for meniscal scaffolds with favorable indications in animal studies. In this context, recent efforts have focused on meniscal tissue engineering, which relies on well-designed scaffolds for regeneration and replacement purposes. Engineered menisci with more elaborate design and better biomimicry have emerged along with a deepening understanding of the composition and architecture, cellular biology and biomechanics of the meniscus.
The optimal scaffold should be characterized by many biophysical and biochemical properties, as well as bioactivity, to guarantee an ECM-like microenvironment for cell survival and differentiation and restoration of anatomical and mechanical properties of native meniscus. Generally, selecting polymers remains a knotty problem since natural polymers present better biocompatibility and intrinsic bioactivity, whereas synthetic polymers have superior mechanical properties. However, single-polymer scaffolds are insufficient to mimic the meniscal composition and structure. To address this problem, in preliminary studies, natural and synthetic polymer combinations have been developed and have shown promising therapeutic results. More importantly, meniscal tissue is a connective tissue with zonal variations in mechanical properties, cellular composition and vascularization. Thus, zonal meniscal regeneration represents another major challenge since individual size and shape variations and stable implant fixation also present difficulties to be overcome. For this purpose, improvements in manufacturing technologies, especially bioreactors and 3D bioprinting, are set to overcome the challenges mentioned above. Bioreactors used in tissue engineering have been proved to provide efficient biomechanical stimulus and thus accelerate region-specific differentiation, demonstrated to be a beneficial way in zonal meniscal reconstruction. Alternatively, 3D bioprinting technologies can be used to manufacturing zonally variant meniscus. 3D bioprinting techniques can ideally help mimic the specific anatomical size and shape of native menisci, and the macro/microporous properties of these fiber-reinforced constructs are also beneficial for guiding ECM deposition and cell distribution. Indeed, advanced bioinks are capable of producing constructs with a composition resembling that of the native meniscus as closely as possible. Furthermore, 3D-printed scaffolds can be loaded with multiple cells and bioactive molecules within bioinks to further simulate dynamic regeneration in vivo. For all these reasons, 3D bioprinting represents one of the most promising strategies in meniscal tissue engineering, although the suboptimal ability of this approach to construct zone-specific scaffolds requires further improvement.
Second, considering the limited self-repair capacity of the meniscus, which is mainly due to a lack of blood vessels, nerves and lymphatic tissue, investigating the innate regeneration process of the meniscus is required (Li et al., 2020a). The top priority for meniscal tissue engineering is still the formation of neotissue at the site of injury, especially the avascular zone of the meniscus, which has no self-healing ability. In the natural healing process, once tissue is injured, endogenous stem cells respond to biochemical signals, migrate to damaged sites, differentiate into somatic cells and restore their morphology and function (Yang et al., 2020). Ideally, these reparative cells usually mobilize upon the provision of chemotactic gradients. However, a major concern for meniscal scaffolds is that almost all endogenous cells are hampered by the surrounding dense ECM, which hinders cell migration (Patel et al., 2019). One additional concern for meniscal scaffolds is limited infiltration into the center of the scaffold. Therefore, polymeric materials that are able to induce cell migration and provide a suitable microenvironment for cell adhesion and proliferation have been shown to be promising candidates for use in meniscal regeneration. For example, Zhang et al. (2016) demonstrated that a 3D-printed PCL scaffold with a mean pore size of 215 μm significantly enhanced seeded cell colonization and further improved meniscal regeneration. Aside from changing the scaffold porosity, the incorporation of chemotactic chemokines could be a promising avenue (Tarafder et al., 2019; Baek et al., 2020; Zhu et al., 2020).
Apart from an amendable interfacial design and proper recruitment signals, relevant stimuli for regulating chondrogenesis are also needed. The discovery and exploration of types of biophysical and biochemical stimuli recently demonstrated promising contributions to meniscal scaffolds for meniscal repair. However, the meniscus exhibits spatial variations in cell phenotypes and components. Therefore, biofactor-functionalized scaffolds capable of spatiotemporal release hold great promise. A series of studies demonstrated that CTGF and TGF-β3 can induce zone-specific matrix formation in vitro and in vivo and may be a suitable combination for meniscal regeneration (Lee et al., 2014). Despite the type of material, biophysical properties, such as mechanical strength and matrix stiffness, can also influence cell fate (Wei et al., 2020). To mimic the development of neomeniscal tissue, the physical stimuli attributed to meniscal regeneration were proven by Zhang et al. (2019). In their study, a customized dynamic tension-compression loading system was developed to accelerate meniscal tissue formation in vitro and achieved anisotropic reconstruction of the meniscus in vivo (Zhang et al., 2019; Figure 5). This technique may inspire the future development and application of polymeric materials. Based on these findings, we believe that the optimization of materials, the selection of bioactive molecules and stimuli and the synergistic effect of their combinations as a bridge over the vicious cycle of chronic meniscal damage and support meniscal regeneration will most likely be future directions (Figure 6).
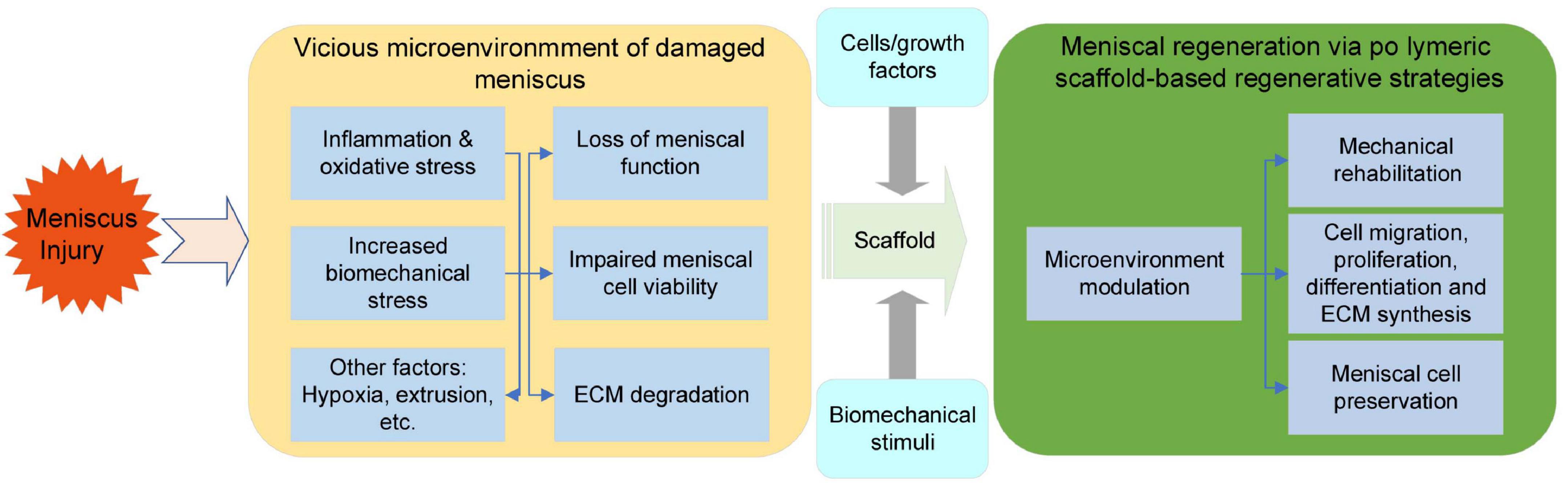
Figure 6. Hostile microenvironment of damaged meniscal tissue and the regenerative process initiated via polymeric scaffolds.
Finally, the success of polymeric biomaterials for meniscal regeneration depends on how well scaffolds interact with the in vivo local meniscal microenvironment and modulate the healing process. The immune response to biomaterials is another major barrier between successful application and scaffold implantation. Meniscal injury is characterized by the activation of inflammation and catabolism. For instance, synovitis after a meniscal tear often leads to mild to moderate inflammation within the joint and acts as a predictor of postmeniscectomy joint dysfunction (Riera et al., 2011; Scanzello et al., 2013). In recent years, we have gained a better understanding of the immunomodulatory effects of biomaterials in tissue regeneration and have paid more attention to designing and manufacturing “immune reprogramming” biomaterials rather than simply “immune friendly” biomaterials. Although previous studies have not yet extensively analyzed or experimented with immunomodulatory biomaterials in the meniscus, we believe that the current biomaterial-mediated immunomodulatory approaches that realize surface modification with bioactive factors will inevitably be beneficial for meniscal tissue engineering in the future.
Currently, we cannot obtain a tissue-engineered meniscus that meets all requirements and is functionalized as it is in its native state. However, a more profound understanding and the application of supportive polymeric materials in combination with other approaches to initiate endogenous meniscal regeneration would provide precious advances for meniscal regeneration (Figure 6).
Conclusion
Treatment of meniscal injuries currently remains a challenge, which calls for an advanced treatment strategy based on an understanding of the pathophysiology of damaged tissue. There is an urgent clinical need for more applicable therapeutics that are able to recapitulate not only the native microarchitecture and components but also the spatiotemporal signaling cascades in the healing process of meniscal tissue. In this progress report, we highlight recent developments in the field of polymeric biomaterials science and associated manufacturing technology for the repair and regeneration of meniscal tissue. Further investigation is required to produce, select, and combine ideal polymeric biomaterials that are repeatable, non-toxic, non-immunogenic, biodegradable, and have the ability to provide biophysical and/or biochemical cues for the regeneration of meniscal tissue. The formulation of a hybrid polymeric scaffold aimed at providing an optimal microenvironment, mechanical support, and delivery bioactivities proved to be a synergistically advanced approach. In addition, current scaffold manufacturing technologies based on 3D printing are proving promising. At the same time, we envision that the zonal meniscal reconstruction and immune compatibility of the scaffold are also vital factor that should be investigated in meniscal tissue engineering. In short, future exploration should be focused on the development of optimum biomaterials and advanced scaffold manufacturing technologies as well as the implications of biomaterial-directed immunomodulation that orchestrates the pro-regenerative process and finally promotes the anisotropic reconstruction of the native, predamaged meniscus.
Author Contributions
HL and PL designed the manuscript and wrote part of it. ZYa wrote the part of the manuscript with insights from all other authors. LF and CG conceptualized and generated Tables 1–4. TZ, WC, ZL, YP, and FC conceptualized and generated Figures 1, 2, 4, 6. XS, ZYu, SL, and QG revised and finalized the manuscript. All authors read and approved the final manuscript, contributed to manuscript revision, and read and approved the submitted version.
Funding
This work was supported by the National Natural Science Foundation of China (81972070) and the National Key R&D Program of China (2019YFA0110600).
Conflict of Interest
The authors declare that the research was conducted in the absence of any commercial or financial relationships that could be construed as a potential conflict of interest.
References
Abar, B., Alonso-Calleja, A., Kelly, A., Kelly, C., Gall, K., and West, J. L. (2020). 3D printing of high-strength, porous, elastomeric structures to promote tissue integration of implants. J. Biomed. Mater. Res. Part A 109, 54–63. doi: 10.1002/jbm.a.37006
Abedalwafa, M., Wang, F., Wang, L., and Li, C. (2013). Biodegradable poly-epsilon-caprolactone (PCL) for tissue engineering applications: a review. Rev. Adv. Mater. Sci. 34, 123–140.
Agarwal, T., Chiesa, I., Presutti, D., Irawan, V., Vajanthri, K. Y., Costantini, M., et al. (2021). Recent advances in bioprinting technologies for engineering different cartilage-based tissues. Mater. Sci. Eng. C Mater. Biol. Appl. 123:112005. doi: 10.1016/j.msec.2021.112005
Aguilar, L. M. C., Silva, S. M., and Moulton, S. E. (2019). Growth factor delivery: defining the next generation platforms for tissue engineering. J. Control. Release 306, 40–58. doi: 10.1016/j.jconrel.2019.05.028
Ahmed, A., and Burke, D. (1983). In-vitro of measurement of static pressure distribution in synovial joints—Part I: tibial surface of the knee. J. Biomech. Eng. 105, 216–225. doi: 10.1115/1.3138409
Altman, G. H., Diaz, F., Jakuba, C., Calabro, T., Horan, R. L., Chen, J., et al. (2003). Silk-based biomaterials. Biomaterials 24, 401–416.
Aoki, K., and Saito, N. (2020). Biodegradable polymers as drug delivery systems for bone regeneration. Pharmaceutics 12:95. doi: 10.3390/pharmaceutics12020095
Arnoczky, S. P., and Warren, R. F. (1982). Microvasculature of the human meniscus. Am. J. Sports Med. 10, 90–95. doi: 10.1177/036354658201000205
Baek, J., Lee, E., Lotz, M. K., and D’Lima, D. D. (2020). Bioactive proteins delivery through core-shell nanofibers for meniscal tissue regeneration. Nanomed. Nanotechnol. Biol. Med. 23:102090. doi: 10.1016/j.nano.2019.102090
Baek, J., Lotz, M. K., and D’Lima, D. D. (2019). Core–Shell nanofibrous scaffolds for repair of meniscus tears. Tissue Eng. Part A 25, 1577–1590. doi: 10.1089/ten.tea.2018.0319
Baek, J., Sovani, S., Choi, W., Jin, S., Grogan, S. P., and D’Lima, D. D. (2018). Meniscal tissue engineering using aligned collagen fibrous scaffolds: comparison of different human cell sources. Tissue Eng. Part A 24, 81–93. doi: 10.1089/ten.TEA.2016.0205
Bahcecioglu, G., Bilgen, B., Hasirci, N., and Hasirci, V. (2019). Anatomical meniscus construct with zone specific biochemical composition and structural organization. Biomaterials 218:119361. doi: 10.1016/j.biomaterials.2019.119361
Bahcecioglu, G., Hasirci, N., Bilgen, B., and Hasirci, V. (2019a). A 3D printed PCL/hydrogel construct with zone-specific biochemical composition mimicking that of the meniscus. Biofabrication 11:025002. doi: 10.1088/1758-5090/aaf707
Bahcecioglu, G., Hasirci, N., Bilgen, B., and Hasirci, V. (2019b). Hydrogels of agarose, and methacrylated gelatin and hyaluronic acid are more supportive for in vitro meniscus regeneration than three dimensional printed polycaprolactone scaffolds. Int. J. Biol. Macromol. 122, 1152–1162. doi: 10.1016/j.ijbiomac.2018.09.065
Baker, B. M., Gee, A. O., Metter, R. B., Nathan, A. S., Marklein, R. A., Burdick, J. A., et al. (2008). The potential to improve cell infiltration in composite fiber-aligned electrospun scaffolds by the selective removal of sacrificial fibers. Biomaterials 29, 2348–2358. doi: 10.1016/j.biomaterials.2008.01.032
Baker, M. I., Walsh, S. P., Schwartz, Z., and Boyan, B. D. (2012). A review of polyvinyl alcohol and its uses in cartilage and orthopedic applications. J. Biomed. Mater. Res. B Appl. Biomater. 100, 1451–1457. doi: 10.1002/jbm.b.32694
Bakhshandeh, B., Zarrintaj, P., Oftadeh, M. O., Keramati, F., Fouladiha, H., Sohrabi-Jahromi, S., et al. (2017). Tissue engineering; strategies, tissues, and biomaterials. Biotechnol. Genet. Eng. Rev. 33, 144–172.
Bandyopadhyay, A., and Mandal, B. B. (2019). A three-dimensional printed silk-based biomimetic tri-layered meniscus for potential patient-specific implantation. Biofabrication 12:015003. doi: 10.1088/1758-5090/ab40fa
Bharadwaz, A., and Jayasuriya, A. C. (2020). Recent trends in the application of widely used natural and synthetic polymer nanocomposites in bone tissue regeneration. Mater. Sci. Eng. C 110:110698. doi: 10.1016/j.msec.2020.110698
Bhargava, M. M., Attia, E. T., Murrell, G. A., Dolan, M. M., Warren, R. F., and Hannafin, J. A. (1999). The effect of cytokines on the proliferation and migration of bovine meniscal cells. Am. J. Sports Med. 27, 636–643. doi: 10.1177/03635465990270051601
Bigoni, M., Turati, M., Sacerdote, P., Gaddi, D., Piatti, M., Castelnuovo, A., et al. (2017). Characterization of synovial fluid cytokine profiles in chronic meniscal tear of the knee. J. Orthop. Res. 35, 340–346. doi: 10.1002/jor.23272
Bilgen, B., Jayasuriya, C. T., and Owens, B. D. (2018). Current concepts in meniscus tissue engineering and repair. Adv. Healthcare Mater. 7:1701407. doi: 10.1002/adhm.201701407
Caliari, S. R., Ramirez, M. A., and Harley, B. A. (2011). The development of collagen-GAG scaffold-membrane composites for tendon tissue engineering. Biomaterials 32, 8990–8998. doi: 10.1016/j.biomaterials.2011.08.035
Castro-Aguirre, E., ñiguez-Franco, F. I., Samsudin, H., Fang, X., and Auras, R. (2016). Poly(lactic acid)—Mass production, processing, industrial applications, and end of life. Adv. Drug Delivery Rev. 107, 333–366. doi: 10.1016/j.addr.2016.03.010
Caterson, E. J., Nesti, L. J., Li, W. J., Danielson, K. G., Albert, T. J., Vaccaro, A. R., et al. (2001). Three-dimensional cartilage formation by bone marrow-derived cells seeded in polylactide/alginate amalgam. J. Biomed. Mater. Res. Official J. Soc. Biomater. Japanese Soc. Biomater. Australian Soc. Biomater. Korean Soc. Biomater. 57, 394–403. doi: 10.1002/1097-4636(20011205)57:3<394::aid-jbm1182>3.0.co;2-9
Cen, L., Liu, W., Cui, L., Zhang, W., and Cao, Y. (2008). Collagen tissue engineering: development of novel biomaterials and applications. Pediatric Res. 63, 492–496. doi: 10.1203/pdr.0b013e31816c5bc3
Cengiz, I. F., Maia, F. R., da Silva Morais, A., Silva-Correia, J., Pereira, H., Canadas, R. F., et al. (2020). Entrapped in cage (EiC) scaffolds of 3D-printed polycaprolactone and porous silk fibroin for meniscus tissue engineering. Biofabrication 12:025028. doi: 10.1088/1758-5090/ab779f
Cengiz, I. F., Pereira, H., Espregueira-Mendes, J., Kwon, I. K., Reis, R. L., and Oliveira, J. M. (2019). Suturable regenerated silk fibroin scaffold reinforced with 3D-printed polycaprolactone mesh: biomechanical performance and subcutaneous implantation. J. Mater. Sci. Mater. Med. 30:63.
Chae, S., Lee, S. S., Choi, Y. J., Hong, D. H., Gao, G., Wang, J. H., et al. (2021). 3D cell-printing of biocompatible and functional meniscus constructs using meniscus-derived bioink. Biomaterials 267:120466. doi: 10.1016/j.biomaterials.2020.120466
Chansoria, P., Narayanan, L. K., Schuchard, K., and Shirwaiker, R. (2019). Ultrasound-assisted biofabrication and bioprinting of preferentially aligned three-dimensional cellular constructs. Biofabrication 11:035015. doi: 10.1088/1758-5090/ab15cf
Chen, M., Feng, Z., Guo, W., Yang, D., Gao, S., Li, Y., et al. (2019). Hybrid scaffolds and meniscal fibrochondrocytes promote whole meniscus regeneration in a rabbit meniscectomy model. ACS Appl. Mater. Interfaces 11, 41626–41639. doi: 10.1021/acsami.9b13611
Chen, P., Zheng, L., Wang, Y., Tao, M., Xie, Z., Xia, C., et al. (2019). Desktop-stereolithography 3D printing of a radially oriented extracellular matrix/mesenchymal stem cell exosome bioink for osteochondral defect regeneration. Theranostics 9:2439. doi: 10.7150/thno.31017
Chocholata, P., Kulda, V., and Babuska, V. (2019). Fabrication of scaffolds for bone-tissue regeneration. Materials 12:568. doi: 10.3390/ma12040568
Chung, L., Maestas, D. R. Jr., Housseau, F., and Elisseeff, J. H. (2017). Key players in the immune response to biomaterial scaffolds for regenerative medicine. Adv. Drug Delivery Rev. 114, 184–192. doi: 10.1016/j.addr.2017.07.006
Cojocaru, D. G., Hondke, S., Kruger, J. P., Bosch, C., Croicu, C., Florescu, S., et al. (2020). Meniscus-shaped cell-free polyglycolic acid scaffold for meniscal repair in a sheep model. J. Biomed. Mater. Res. B Appl. Biomater. 108, 809–818. doi: 10.1002/jbm.b.34435
Collins, M. N., and Birkinshaw, C. (2013). Hyaluronic acid based scaffolds for tissue engineering—A review. Carbohydrate Polymers 92, 1262–1279. doi: 10.1016/j.carbpol.2012.10.028
Corbel, S., Dufaud, O., and Roques-Carmes, T. (2011). Materials for Stereolithography, Stereolithography. Berlin: Springer, 141–159.
Crema, M., Guermazi, A., Li, L., Nogueira-Barbosa, M., Marra, M., Roemer, F., et al. (2010). The association of prevalent medial meniscal pathology with cartilage loss in the medial tibiofemoral compartment over a 2-year period. Osteoarthritis Cartilage 18, 336–343. doi: 10.1016/j.joca.2009.11.003
Cui, Z.-K., Kim, S., Baljon, J. J., Wu, B. M., Aghaloo, T., and Lee, M. (2019). Microporous methacrylated glycol chitosan-montmorillonite nanocomposite hydrogel for bone tissue engineering. Nat. Commun. 10:3523.
da Silva Morais, A., Vieira, S., Zhao, X., Mao, Z., Gao, C., Oliveira, J. M., et al. (2020). Advanced biomaterials and processing methods for liver regeneration: state-of-the-art and future trends. Adv. Healthcare Mater. 9:1901435. doi: 10.1002/adhm.201901435
Danhier, F., Ansorena, E., Silva, J. M., Coco, R., Le Breton, A., and Préat, V. (2012). PLGA-based nanoparticles: an overview of biomedical applications. J. Control. Release 161, 505–522. doi: 10.1016/j.jconrel.2012.01.043
Depres-Tremblay, G., Chevrier, A., Snow, M., Rodeo, S., and Buschmann, M. D. (2019). Freeze-dried chitosan-platelet-rich plasma implants improve supraspinatus tendon attachment in a transosseous rotator cuff repair model in the rabbit. J. Biomater. Appl. 33, 792–807. doi: 10.1177/0885328218811386
Donnaloja, F., Jacchetti, E., Soncini, M., and Raimondi, M. T. (2020). Natural and synthetic polymers for bone scaffolds optimization. Polymers (Basel) 12:905. doi: 10.3390/polym12040905
Drobnič, M., Ercin, E., Gamelas, J., Papacostas, E. T., Slynarski, K., Zdanowicz, U., et al. (2019). Treatment options for the symptomatic post-meniscectomy knee. Knee Surg. Sports Traumatol. Arthroscopy 27, 1817–1824. doi: 10.1007/s00167-019-05424-3
Drosos, G., and Pozo, J. (2004). The causes and mechanisms of meniscal injuries in the sporting and non-sporting environment in an unselected population. Knee 11, 143–149. doi: 10.1016/s0968-0160(03)00105-4
Echave, M. C., Burgo, L. S., Pedraz, J. L., and Orive, G. (2017). Gelatin as biomaterial for tissue engineering. Curr. Pharm. Design 23, 3567–3584.
Elliott, M. B., Ginn, B., Fukunishi, T., Bedja, D., Suresh, A., Chen, T., et al. (2019). Regenerative and durable small-diameter graft as an arterial conduit. Proc. Natl. Acad. Sci. U.S.A. 116, 12710–12719. doi: 10.1073/pnas.1905966116
Engineer, C., Parikh, J., and Raval, A. (2011). Review on hydrolytic degradation behavior of biodegradable polymers from controlled drug delivery system. Trends Biomater. Artificial Organs 25, 79–85.
Englund, M., Niu, J., Guermazi, A., Roemer, F., Hunter, D., Lynch, J., et al. (2007). Effect of meniscal damage on the development of frequent knee pain, aching, or stiffness. Arthritis Rheumatism Official J. Am. Coll. Rheumatol. 56, 4048–4054. doi: 10.1002/art.23071
Englund, M., Roemer, F. W., Hayashi, D., Crema, M. D., and Guermazi, A. (2012). Meniscus pathology, osteoarthritis and the treatment controversy. Nat. Rev. Rheumatol. 8:412. doi: 10.1038/nrrheum.2012.69
Esposito, A. R., Moda, M., Cattani, S.M.d.M, de Santana, G. M., Barbieri, J. A., Munhoz, M. M., et al. (2013). PLDLA/PCL-T scaffold for meniscus tissue engineering. BioRes. Open Access 2, 138–147. doi: 10.1089/biores.2012.0293
Fairbank, T. (1948). Knee joint changes after meniscectomy. J. Bone Joint Surg. 30, 664–670. doi: 10.1302/0301-620x.30b4.664
Filardo, G., Petretta, M., Cavallo, C., Roseti, L., Durante, S., Albisinni, U., et al. (2019). Patient-specific meniscus prototype based on 3D bioprinting of human cell-laden scaffold. Bone Joint Res. 8, 101–106. doi: 10.1302/2046-3758.82.bjr-2018-0134.r1
Fillingham, Y. A., Riboh, J. C., Erickson, B. J., Bach, B. R. Jr., and Yanke, A. B. (2017). Inside-out versus all-inside repair of isolated meniscal tears: an updated systematic review. Am. J. Sports Med. 45, 234–242. doi: 10.1177/0363546516632504
Fithian, D. C., Kelly, M. A., and Mow, V. C. (1990). Material properties and structure-function relationships in the menisci. Clin. Orthop. Related Res. 252, 19–31.
Fu, L., Yang, Z., Gao, C., Li, H., Yuan, Z., Wang, F., et al. (2020). Advances and prospects in biomimetic multilayered scaffolds for articular cartilage regeneration. Regen. Biomater. 7, 527–542. doi: 10.1093/rb/rbaa042
Gaharwar, A. K., Singh, I., and Khademhosseini, A. (2020). Engineered biomaterials for in situ tissue regeneration. Nat. Rev. Mater. 5, 686–705. doi: 10.1038/s41578-020-0209-x
Gentile, P., Chiono, V., Carmagnola, I., and Hatton, P. V. (2014). An overview of poly (lactic-co-glycolic) acid (PLGA)-based biomaterials for bone tissue engineering. Int. J. Mol. Sci. 15, 3640–3659. doi: 10.3390/ijms15033640
Ghodbane, S. A., Patel, J. M., Brzezinski, A., Lu, T. M., Gatt, C. J., and Dunn, M. G. (2019). Biomechanical characterization of a novel collagen-hyaluronan infused 3D-printed polymeric device for partial meniscus replacement. J. Biomed. Mater. Res. Part B Appl. Biomater. 107, 2457–2465. doi: 10.1002/jbm.b.34336
Gilbert, T. W., Sellaro, T. L., and Badylak, S. F. (2006). Decellularization of tissues and organs. Biomaterials 27, 3675–3683.
Gopinathan, J., Mano, S., Elakkiya, V., Pillai, M. M., Sahanand, K. S., Rai, B. D., et al. (2015). Biomolecule incorporated poly-ε-caprolactone nanofibrous scaffolds for enhanced human meniscal cell attachment and proliferation. RSC Adv. 5, 73552–73561. doi: 10.1039/c5ra14315b
Gregor, A., Filová, E., Novák, M., Kronek, J., Chlup, H., Buzgo, M., et al. (2017). Designing of PLA scaffolds for bone tissue replacement fabricated by ordinary commercial 3D printer. J. Biol. Eng. 11:31.
Grogan, S. P., Chung, P. H., Soman, P., Chen, P., Lotz, M. K., Chen, S., et al. (2013). Digital micromirror device projection printing system for meniscus tissue engineering. Acta Biomater. 9, 7218–7226. doi: 10.1016/j.actbio.2013.03.020
Gu, Y., Zhu, W., Hao, Y., Lu, L., Chen, Y., and Wang, Y. (2012). Repair of meniscal defect using an induced myoblast-loaded polyglycolic acid mesh in a canine model. Exp. Therap. Med. 3, 293–298. doi: 10.3892/etm.2011.403
Gui, L., Zhao, L., Spencer, R. W., Burghouwt, A., Taylor, M. S., Shalaby, S. W., et al. (2011). Development of novel biodegradable polymer scaffolds for vascular tissue engineering. Tissue Eng. Part A 17, 1191–1200. doi: 10.1089/ten.tea.2010.0508
Gunja, N. J., and Athanasiou, K. A. (2010). Additive and synergistic effects of bFGF and hypoxia on leporine meniscus cell-seeded PLLA scaffolds. J. Tissue Eng. Regen. Med. 4, 115–122. doi: 10.1002/term.221
Guo, W., Chen, M., Wang, Z., Tian, Y., Zheng, J., Gao, S., et al. (2021). 3D-printed cell-free PCL–MECM scaffold with biomimetic micro-structure and micro-environment to enhance in situ meniscus regeneration. Bioact. Mater. 6, 3620–3633. doi: 10.1016/j.bioactmat.2021.02.019
Gupta, S., Sharma, A., Kumar, J. V., Sharma, V., Gupta, P. K., and Verma, R. S. (2020b). Meniscal tissue engineering via 3D printed PLA monolith with carbohydrate based self-healing interpenetrating network hydrogel. Int. J. Biol. Macromol. 162, 1358–1371.
Gupta, S., Sharma, A., Vasantha Kumar, J., Sharma, V., Gupta, P. K., and Verma, R. S. (2020a). Meniscal tissue engineering via 3D printed PLA monolith with carbohydrate based self-healing interpenetrating network hydrogel. Int. J. Biol. Macromol. 162, 1358–1371. doi: 10.1016/j.ijbiomac.2020.07.238
Guziewicz, N., Best, A., Perez-Ramirez, B., and Kaplan, D. L. (2011). Lyophilized silk fibroin hydrogels for the sustained local delivery of therapeutic monoclonal antibodies. Biomaterials 32, 2642–2650. doi: 10.1016/j.biomaterials.2010.12.023
Hayes, J. C., and Kennedy, J. E. (2016). An evaluation of the biocompatibility properties of a salt-modified polyvinyl alcohol hydrogel for a knee meniscus application. Mater. Sci. Eng. C Mater. Biol. Appl. 59, 894–900. doi: 10.1016/j.msec.2015.10.052
Holloway, J. L., Lowman, A. M., and Palmese, G. R. (2010). Mechanical evaluation of poly(vinyl alcohol)-based fibrous composites as biomaterials for meniscal tissue replacement. Acta Biomater. 6, 4716–4724. doi: 10.1016/j.actbio.2010.06.025
Holloway, J. L., Lowman, A. M., VanLandingham, M. R., and Palmese, G. R. (2014). Interfacial optimization of fiber-reinforced hydrogel composites for soft fibrous tissue applications. Acta Biomater. 10, 3581–3589. doi: 10.1016/j.actbio.2014.05.004
Hoque, M. E., Nuge, T., Yeow, T. K., Nordin, N., and Prasad, R. (2015). Gelatin based scaffolds for tissue engineering-a review. Polymers Res. J. 9, 15–32.
Ishida, K., Kuroda, R., Miwa, M., Tabata, Y., Hokugo, A., Kawamoto, T., et al. (2007). The regenerative effects of platelet-rich plasma on meniscal cells in vitro and its in vivo application with biodegradable gelatin hydrogel. Tissue Eng. 13, 1103–1112. doi: 10.1089/ten.2006.0193
Izzo, D., Palazzo, B., Scalera, F., Gullotta, F., Lapesa, V., Scialla, S., et al. (2019). Chitosan scaffolds for cartilage regeneration: influence of different ionic crosslinkers on biomaterial properties. Int. J. Polym. Mater. Polym. Biomater. 68, 936–945. doi: 10.1080/00914037.2018.1525538
Jacob, G., Shimomura, K., Krych, A. J., and Nakamura, N. (2020). The meniscus tear: a review of stem cell therapies. Cells 9:92. doi: 10.3390/cells9010092
Ji, X., Lei, Z., Yuan, M., Zhu, H., Yuan, X., Liu, W., et al. (2020). Cartilage repair mediated by thermosensitive photocrosslinkable TGFβ1-loaded GM-HPCH via immunomodulating macrophages, recruiting MSCs and promoting chondrogenesis. Theranostics 10:2872. doi: 10.7150/thno.41622
Jones, J. C., Burks, R., Owens, B. D., Sturdivant, R. X., Svoboda, S. J., and Cameron, K. L. (2012). Incidence and risk factors associated with meniscal injuries among active-duty US military service members. J. Athletic Train. 47, 67–73. doi: 10.4085/1062-6050-47.1.67
Kashirina, A., Yao, Y., Liu, Y., and Leng, J. (2019). Biopolymers as bone substitutes: a review. Biomater. Sci. 7, 3961–3983. doi: 10.1039/c9bm00664h
Katz, J. N., and Martin, S. D. (2009). Meniscus–friend or foe: epidemiologic observations and surgical implications. Arthritis Rheumatism 60:633. doi: 10.1002/art.24363
Katz, J. N., Brophy, R. H., Chaisson, C. E., De Chaves, L., Cole, B. J., Dahm, D. L., et al. (2013). Surgery versus physical therapy for a meniscal tear and osteoarthritis. N. Engl. J. Med. 368, 1675–1684.
Kim, I. L., Mauck, R. L., and Burdick, J. A. (2011a). Hydrogel design for cartilage tissue engineering: a case study with hyaluronic acid. Biomaterials 32, 8771–8782. doi: 10.1016/j.biomaterials.2011.08.073
Kim, J. K., Kim, H. J., Chung, J.-Y., Lee, J.-H., Young, S.-B., and Kim, Y.-H. (2014). Natural and synthetic biomaterials for controlled drug delivery. Arch. Pharm. Res. 37, 60–68.
Kim, S., Bosque, J., Meehan, J. P., Jamali, A., and Marder, R. (2011b). Increase in outpatient knee arthroscopy in the United States: a comparison of National Surveys of Ambulatory Surgery, 1996 and 2006. JBJS 93, 994–1000. doi: 10.2106/jbjs.i.01618
Kim, W., Onodera, T., Kondo, E., Kawaguchi, Y., Terkawi, M. A., Baba, R., et al. (2019). Effects of ultra-purified alginate gel implantation on meniscal defects in rabbits. Am. J. Sports Med. 47, 640–650. doi: 10.1177/0363546518816690
Kobayashi, M., Chang, Y. S., and Oka, M. (2005). A two year in vivo study of polyvinyl alcohol-hydrogel (PVA-H) artificial meniscus. Biomaterials 26, 3243–3248. doi: 10.1016/j.biomaterials.2004.08.028
Koch, M., Achatz, F. P., Lang, S., Pfeifer, C. G., Pattappa, G., Kujat, R., et al. (2018). Tissue engineering of large full-size meniscus defects by a polyurethane scaffold: accelerated regeneration by mesenchymal stromal cells. Stem Cells Int. 2018:8207071.
Kwak, H. S., Nam, J., Lee, J.h, Kim, H. J., and Yoo, J. J. (2017). Meniscal repair in vivo using human chondrocyte-seeded PLGA mesh scaffold pretreated with platelet-rich plasma. J. Tissue Eng. Regen. Med. 11, 471–480. doi: 10.1002/term.1938
Kwon, H., Brown, W. E., Lee, C. A., Wang, D., Paschos, N., Hu, J. C., et al. (2019). Surgical and tissue engineering strategies for articular cartilage and meniscus repair. Nat. Rev. Rheumatol. 15, 550–570. doi: 10.1038/s41584-019-0255-1
Lam, T., Dehne, T., Krüger, J. P., Hondke, S., Endres, M., Thomas, A., et al. (2019). Photopolymerizable gelatin and hyaluronic acid for stereolithographic 3D bioprinting of tissue-engineered cartilage. J. Biomed. Mater. Res. Part B Appl. Biomater. 107, 2649–2657. doi: 10.1002/jbm.b.34354
Lee, C. H., Rodeo, S. A., Fortier, L. A., Lu, C., Erisken, C., and Mao, J. J. (2014). Protein-releasing polymeric scaffolds induce fibrochondrocytic differentiation of endogenous cells for knee meniscus regeneration in sheep. Sci. Transl. Med. 6:266ra171. doi: 10.1126/scitranslmed.3009696
Lee, J., Byun, H., Madhurakkat Perikamana, S. K., Lee, S., and Shin, H. (2019). Current advances in immunomodulatory biomaterials for bone regeneration. Adv. Healthcare Mater. 8:1801106. doi: 10.1002/adhm.201801106
Lee, K. Y., and Mooney, D. J. (2012). Alginate: properties and biomedical applications. Progr. Polymer Sci. 37, 106–126. doi: 10.1016/j.progpolymsci.2011.06.003
Lee, S. Y., Niikura, T., and Reddi, A. H. (2008). Superficial zone protein (lubricin) in the different tissue compartments of the knee joint: modulation by transforming growth factor beta 1 and interleukin-1 beta. Tissue Eng. Part A 14, 1799–1808. doi: 10.1089/ten.tea.2007.0367
Levengood, S. K. L., and Zhang, M. (2014). Chitosan-based scaffolds for bone tissue engineering. J. Mater. Chem. B 2, 3161–3184.
Li, H., Yang, Z., Fu, L., Yuan, Z., Gao, C., Sui, X., et al. (2020a). Advanced polymer-based drug delivery strategies for meniscal regeneration. Tissue Eng. Part B Rev. doi: 10.1089/ten.TEB.2020.0156 [Epub ahead of print].
Li, X., Liu, B., Pei, B., Chen, J., Zhou, D., Peng, J., et al. (2020c). Inkjet bioprinting of biomaterials. Chem. Rev. 120, 10793–10833. doi: 10.1021/acs.chemrev.0c00008
Li, Y., Chen, M., Zhou, W., Gao, S., Luo, X., Peng, L., et al. (2020b). Cell-free 3D wet-electrospun PCL/silk fibroin/Sr2+ scaffold promotes successful total meniscus regeneration in a rabbit model. Acta Biomater. 113, 196–209. doi: 10.1016/j.actbio.2020.06.017
Li, Z., Wu, N., Cheng, J., Sun, M., Yang, P., Zhao, F., et al. (2020d). Biomechanically, structurally and functionally meticulously tailored polycaprolactone/silk fibroin scaffold for meniscus regeneration. Theranostics 10, 5090–5106.
Li, Z., Wu, N., Cheng, J., Sun, M., Yang, P., Zhao, F., et al. (2020e). Biomechanically, structurally and functionally meticulously tailored polycaprolactone/silk fibroin scaffold for meniscus regeneration. Theranostics 10:5090. doi: 10.7150/thno.44270
Liang, Y., Idrees, E., Andrews, S. H., Labib, K., Szojka, A., Kunze, M., et al. (2017). Plasticity of human meniscus fibrochondrocytes: a study on effects of mitotic divisions and oxygen tension. Sci. Rep. 7, 1–13.
Liang, Y., Idrees, E., Szojka A. R., Andrews, S. H., Kunze, M., Mulet-Sierra, A., et al. (2018). Chondrogenic differentiation of synovial fluid mesenchymal stem cells on human meniscus-derived decellularized matrix requires exogenous growth factors. Acta Biomater. 80, 131–143. doi: 10.1016/j.actbio.2018.09.038
Logerstedt, D. S., Snyder-Mackler, L., Ritter, R. C., Axe, M. J., Godges, J., Altman, R. D., et al. (2010). Knee pain and mobility impairments: meniscal and articular cartilage lesions: clinical practice guidelines linked to the international classification of functioning, disability, and health from the orthopaedic section of the American Physical Therapy Association. J. Orthop. Sports Phys. Ther. 40, A1–A35.
Lü, J.-M., Wang, X., Marin-Muller, C., Wang, H., Lin, P. H., Yao, Q., et al. (2009). Current advances in research and clinical applications of PLGA-based nanotechnology. Expert Rev. Mol. Diagnostics 9, 325–341. doi: 10.1586/erm.09.15
MacBarb, R. F., Chen, A. L., Hu, J. C., and Athanasiou, K. A. (2013). Engineering functional anisotropy in fibrocartilage neotissues. Biomaterials 34, 9980–9989. doi: 10.1016/j.biomaterials.2013.09.026
Makris, E. A., Hadidi, P., and Athanasiou, K. A. (2011). The knee meniscus: structure–function, pathophysiology, current repair techniques, and prospects for regeneration. Biomaterials 32, 7411–7431. doi: 10.1016/j.biomaterials.2011.06.037
Marcheggiani Muccioli, G. M., Lullini, G., Cammisa, E., Leardini, A., and Zaffagnini, S. (2020). A professional athlete functionally active 10 years after an arthroscopic lateral collagen meniscus implant. Knee Surg. Sports Traumatol. Arthroscopy 29, 117–119. doi: 10.1007/s00167-020-05876-y
Marrella, A., Lagazzo, A., Dellacasa, E., Pasquini, C., Finocchio, E., Barberis, F., et al. (2018). 3D Porous Gelatin/PVA hydrogel as meniscus substitute using alginate micro-particles as porogens. Polymers (Basel) 10:380. doi: 10.3390/polym10040380
Matai, I., Kaur, G., Seyedsalehi, A., McClinton, A., and Laurencin, C. T. (2020). Progress in 3D bioprinting technology for tissue/organ regenerative engineering. Biomaterials 226:119536. doi: 10.1016/j.biomaterials.2019.119536
Megelski, S., Stephens, J. S., Chase, D. B., and Rabolt, J. F. (2002). Micro-and nanostructured surface morphology on electrospun polymer fibers. Macromolecules 35, 8456–8466. doi: 10.1021/ma020444a
Mi, F.-L., Tan, Y.-C., Liang, H.-F., and Sung, H.-W. (2002). In vivo biocompatibility and degradability of a novel injectable-chitosan-based implant. Biomaterials 23, 181–191. doi: 10.1016/s0142-9612(01)00094-1
Mir, M., Ahmed, N., and ur Rehman, A. (2017). Recent applications of PLGA based nanostructures in drug delivery. Colloids Surfaces B Biointerfaces 159, 217–231. doi: 10.1016/j.colsurfb.2017.07.038
Mondal, D., Griffith, M., and Venkatraman, S. S. (2016). Polycaprolactone-based biomaterials for tissue engineering and drug delivery: current scenario and challenges. Int. J. Polym. Mater. Polym. Biomater. 65, 255–265. doi: 10.1080/00914037.2015.1103241
Monk, P., Garfjeld Roberts, P., Palmer, A. J., Bayliss, L., Mafi, R., Beard, D., et al. (2017). The urgent need for evidence in arthroscopic meniscal surgery: a systematic review of the evidence for operative management of meniscal tears. Am. J. Sports Med. 45, 965–973. doi: 10.1177/0363546516650180
Montgomery, S. R., Zhang, A., Ngo, S. S., Wang, J. C., and Hame, S. L. (2013). Cross-sectional analysis of trends in meniscectomy and meniscus repair. Orthopedics 36, e1007–e1013.
Moradi, L., Vasei, M., Dehghan, M. M., Majidi, M., Farzad Mohajeri, S., and Bonakdar, S. (2017a). Regeneration of meniscus tissue using adipose mesenchymal stem cells-chondrocytes co-culture on a hybrid scaffold: in vivo study. Biomaterials 126, 18–30.
Moradi, L., Vasei, M., Dehghan, M. M., Majidi, M., Mohajeri, S. F., and Bonakdar, S. (2017b). Regeneration of meniscus tissue using adipose mesenchymal stem cells-chondrocytes co-culture on a hybrid scaffold: in vivo study. Biomaterials 126, 18–30. doi: 10.1016/j.biomaterials.2017.02.022
Moroni, L., Boland, T., Burdick, J. A., De Maria, C., Derby, B., Forgacs, G., et al. (2018). Biofabrication: a guide to technology and terminology. Trends Biotechnol. 36, 384–402. doi: 10.1016/j.tibtech.2017.10.015
Murakami, T., Otsuki, S., Okamoto, Y., Nakagawa, K., Wakama, H., Okuno, N., et al. (2019). Hyaluronic acid promotes proliferation and migration of human meniscus cells via a CD44-dependent mechanism. Connect. Tissue Res. 60, 117–127. 2, doi: 10.1080/03008207.2018.1465053
Murariu, M., and Dubois, P. (2016). PLA composites: from production to properties. Adv. Drug Delivery Rev. 107, 17–46. doi: 10.1016/j.addr.2016.04.003
Murphy, C. A., Costa, J. B., Silva-Correia, J., Oliveira, J. M., Reis, R. L., and Collins, M. N. (2018). Biopolymers and polymers in the search of alternative treatments for meniscal regeneration: state of the art and future trends. Appl. Mater. Today 12, 51–71. doi: 10.1016/j.apmt.2018.04.002
Murphy, C. A., Garg, A. K., Silva-Correia, J., Reis, R. L., Oliveira, J. M., and Collins, M. N. (2019). The meniscus in normal and osteoarthritic tissues: facing the structure property challenges and current treatment trends. Ann. Rev. Biomed. Eng. 21, 495–521. doi: 10.1146/annurev-bioeng-060418-052547
Narayanan, L. K., Thompson, T. L., Shirwaiker, R. A., and Starly, B. (2018). Label free process monitoring of 3D bioprinted engineered constructs via dielectric impedance spectroscopy. Biofabrication 10:035012. doi: 10.1088/1758-5090/aaccbf
Narita, A., Takahara, M., Sato, D., Ogino, T., Fukushima, S., Kimura, Y., et al. (2012). Biodegradable gelatin hydrogels incorporating fibroblast growth factor 2 promote healing of horizontal tears in rabbit meniscus. Arthroscopy J. Arthroscopic Related Surg. 28, 255–263. doi: 10.1016/j.arthro.2011.08.294
Noyes, F. R., and Barber-Westin, S. D. (2010). Repair of complex and avascular meniscal tears and meniscal transplantation. JBJS 92, 1012–1029.
Noyes, F. R., and Barber-Westin, S. D. (2016). Long-term survivorship and function of meniscus transplantation. Am. J. Sports Med. 44, 2330–2338. doi: 10.1177/0363546516646375
Ogay, V., Mun, E. A., Kudaibergen, G., Baidarbekov, M., Kassymbek, K., Zharkinbekov, Z., et al. (2020). Progress and prospects of polymer-based drug delivery systems for bone tissue regeneration. Polymers 12:2881. doi: 10.3390/polym12122881
Olivos-Meza, A., érez Jiménez, F. J. P., Granados-Montiel, J., Landa-Solís, C., Cortés González, S., Jiménez Aroche, C. A., et al. (2019). First clinical application of polyurethane meniscal scaffolds with mesenchymal stem cells and assessment of cartilage quality with T2 Mapping at 12 Months. Cartilage doi: 10.1177/1947603519852415 [Epub ahead of print].
Otsuki, S., Nakagawa, K., Murakami, T., Sezaki, S., Sato, H., Suzuki, M., et al. (2019). Evaluation of meniscal regeneration in a mini pig model treated with a novel polyglycolic acid meniscal scaffold. Am. J. Sports Med. 47, 1804–1815. doi: 10.1177/0363546519850578
Pangborn, C. A., and Athanasiou, K. A. (2005). Effects of growth factors on meniscal fibrochondrocytes. Tissue Eng. 11, 1141–1148. doi: 10.1089/ten.2005.11.1141
Parkinson, B., Smith, N., Asplin, L., Thompson, P., and Spalding, T. (2016). Factors predicting meniscal allograft transplantation failure. Orthop. J. Sports Med. 4:2325967116663185.
Patel, J. M., Saleh, K. S., Burdick, J. A., and Mauck, R. L. (2019). Bioactive factors for cartilage repair and regeneration: improving delivery, retention, and activity. Acta Biomater. 93, 222–238. doi: 10.1016/j.actbio.2019.01.061
Peretti, G. M., Gill, T. J., Xu, J.-W., Randolph, M. A., Morse, K. R., and Zaleske, D. J. (2004). Cell-based therapy for meniscal repair: a large animal study. Am. J. Sports Med. 32, 146–158. doi: 10.1177/0095399703258790
Petersen, W., and Tillmann, B. (1995). Age-related blood and lymph supply of the knee menisci: a cadaver study. Acta Orthop. Scand. 66, 308–312. doi: 10.3109/17453679508995550
Pihl, K., Englund, M., Lohmander, L. S., Jørgensen, U., Nissen, N., Schjerning, J., et al. (2017). Signs of knee osteoarthritis common in 620 patients undergoing arthroscopic surgery for meniscal tear. Acta Orthop. 88, 90–95. doi: 10.1080/17453674.2016.1253329
Pillai, M. M., Gopinathan, J., Senthil Kumar, R., Sathish Kumar, G., Shanthakumari, S., Sahanand, K. S., et al. (2018). Tissue engineering of human knee meniscus using functionalized and reinforced silk-polyvinyl alcohol composite three-dimensional scaffolds: Understanding the in vitro and in vivo behavior. J. Biomed. Mater. Res. A 106, 1722–1731. doi: 10.1002/jbm.a.36372
Prabhath, A., Vernekar, V. N., Sanchez, E., and Laurencin, C. T. (2018). Growth factor delivery strategies for rotator cuff repair and regeneration. Int. J. Pharm. 544, 358–371. doi: 10.1016/j.ijpharm.2018.01.006
Puetzer, J. L., and Bonassar, L. J. (2016). Physiologically distributed loading patterns drive the formation of zonally organized collagen structures in tissue-engineered meniscus. Tissue Eng. Part A 22, 907–916. doi: 10.1089/ten.tea.2015.0519
Puetzer, J. L., Brown, B. N., Ballyns, J. J., and Bonassar, L. J. (2013). The effect of IGF-I on anatomically shaped tissue-engineered menisci. Tissue Eng. Part A 19, 1443–1450. doi: 10.1089/ten.tea.2012.0645
Qu, D., Zhu, J. P., Childs, H. R., and Lu, H. H. (2019). Nanofiber-based transforming growth factor-β3 release induces fibrochondrogenic differentiation of stem cells. Acta Biomater. 93, 111–122. doi: 10.1016/j.actbio.2019.03.019
Qu, F., Lin, J.-M. G., Esterhai, J. L., Fisher, M. B., and Mauck, R. L. (2013). Biomaterial-mediated delivery of degradative enzymes to improve meniscus integration and repair. Acta Biomater. 9, 6393–6402. doi: 10.1016/j.actbio.2013.01.016
Qu, M., Wang, C., Zhou, X., Libanori, A., Jiang, X., Xu, W., et al. (2021). Multi-Dimensional Printing for Bone Tissue Engineering. Adv. Healthcare Mater. 10:2001986.
Resmi, R., Parvathy, J., John, A., and Joseph, R. (2020). Injectable self-crosslinking hydrogels for meniscal repair: a study with oxidized alginate and gelatin. Carbohydr. Polym. 234:115902. doi: 10.1016/j.carbpol.2020.115902
Riera, K. M., Rothfusz, N. E., Wilusz, R. E., Weinberg, J., Guilak, F., and McNulty, A. L. (2011). Interleukin-1, tumor necrosis factor-alpha, and transforming growth factor-beta 1 and integrative meniscal repair: influences on meniscal cell proliferation and migration. Arthritis Res. Ther. 13:R187.
Rongen, J. J., van Tienen, T. G., van Bochove, B., Grijpma, D. W., and Buma, P. (2014). Biomaterials in search of a meniscus substitute. Biomaterials 35, 3527–3540. doi: 10.1016/j.biomaterials.2014.01.017
Roos, H., Laurén, M., Adalberth, T., Roos, E. M., Jonsson, K., and Lohmander, L. S. (1998). Knee osteoarthritis after meniscectomy: prevalence of radiographic changes after twenty-one years, compared with matched controls. Arthritis Rheumatism Official J. Am. Coll. Rheumatol. 41, 687–693. doi: 10.1002/1529-0131(199804)41:4<687::aid-art16>3.0.co;2-2
Roseti, L., Cavallo, C., Desando, G., Parisi, V., Petretta, M., Bartolotti, I., et al. (2018). Three-dimensional bioprinting of cartilage by the use of stem cells: a strategy to improve regeneration. Materials (Basel) 11:1749. doi: 10.3390/ma11091749
Rothrauff, B. B., Shimomura, K., Gottardi, R., Alexander, P. G., and Tuan, R. S. (2017). Anatomical region-dependent enhancement of 3-dimensional chondrogenic differentiation of human mesenchymal stem cells by soluble meniscus extracellular matrix. Acta Biomater. 49, 140–151. doi: 10.1016/j.actbio.2016.11.046
Rowley, A. T., Nagalla, R. R., Wang, S. W., and Liu, W. F. (2019). Extracellular matrix-based strategies for immunomodulatory biomaterials engineering. Adv. Healthcare Mater. 8:1801578. doi: 10.1002/adhm.201801578
Ruprecht, J. C., Waanders, T. D., Rowland, C. R., Nishimuta, J. F., Glass, K. A., Stencel, J., et al. (2019). Meniscus-derived matrix scaffolds promote the integrative repair of meniscal defects. Sci. Rep. 9, 1–13.
Salati, M. A., Khazai, J., Tahmuri, A. M., Samadi, A., Taghizadeh, A., Taghizadeh, M., et al. (2020a). Agarose-based biomaterials: opportunities and challenges in cartilage tissue engineering. Polymers 12:1150. doi: 10.3390/polym12051150
Salati, M. A., Khazai, J., Tahmuri, A. M., Samadi, A., Taghizadeh, A., Taghizadeh, M., et al. (2020b). Agarose-based biomaterials: opportunities and challenges in cartilage tissue engineering. Polymers 12:1150.
Samadian, H., Maleki, H., Allahyari, Z., and Jaymand, M. (2020). Natural polymers-based light-induced hydrogels: promising biomaterials for biomedical applications. Coord. Chem. Rev. 420:213432. doi: 10.1016/j.ccr.2020.213432
Sandri, G., Rossi, S., Bonferoni, M. C., Miele, D., Faccendini, A., Del Favero, E., et al. (2019). Chitosan/glycosaminoglycan scaffolds for skin reparation. Carbohydr. Polym. 220, 219–227. doi: 10.1016/j.carbpol.2019.05.069
Santoro, M., Tatara, A. M., and Mikos, A. G. (2014). Gelatin carriers for drug and cell delivery in tissue engineering. J. Controll. Release 190, 210–218. doi: 10.1016/j.jconrel.2014.04.014
Sarkar, M. R., Augat, P., Shefelbine, S. J., Schorlemmer, S., Huber-Lang, M., Claes, L., et al. (2006). Bone formation in a long bone defect model using a platelet-rich plasma-loaded collagen scaffold. Biomaterials 27, 1817–1823. doi: 10.1016/j.biomaterials.2005.10.039
Sawicka, K. M., Seeliger, M., Musaev, T., Macri, L. K., and Clark, R. A. (2015). Fibronectin interaction and enhancement of growth factors: importance for wound healing. Adv. Wound Care 4, 469–478. doi: 10.1089/wound.2014.0616
Scanzello, C. R., Albert, A. S., DiCarlo, E., Rajan, K. B., Kanda, V., Asomugha, E. U., et al. (2013). The influence of synovial inflammation and hyperplasia on symptomatic outcomes up to 2 years post-operatively in patients undergoing partial meniscectomy. Osteoarthr. Cartilage 21, 1392–1399. doi: 10.1016/j.joca.2013.05.011
Shah, S. A., Sohail, M., Minhas, M. U., Khan, S., de Matas, M., Sikstone, V., et al. (2019). Biopolymer-based biomaterials for accelerated diabetic wound healing: a critical review. Int. J. Biol. Macromol. 139, 975–993. doi: 10.1016/j.ijbiomac.2019.08.007
Siclari, A., Krueger, J. P., Endres, M., and Boux, E. (2018). A 24-month follow-up after treatment of hallux rigidus with resection arthroplasty in combination with a resorbable polymer-based implant and platelet-rich plasma. Foot Ankle Surg. 24, 389–393. doi: 10.1016/j.fas.2017.04.007
Silva, M., Ferreira, F. N., Alves, N. M., and Paiva, M. C. (2020). Biodegradable polymer nanocomposites for ligament/tendon tissue engineering. J. Nanobiotechnol. 18:23.
Song, K. H., Heo, S. J., Peredo, A. P., Davidson, M. D., Mauck, R. L., and Burdick, J. A. (2019). Influence of fiber stiffness on meniscal cell migration into dense fibrous networks. Adv. Healthcare Mater. 9:1901228. doi: 10.1002/adhm.201901228
Stabile, K. J., Odom, D., Smith, T. L., Northam, C., Whitlock, P. W., Smith, B. P., et al. (2010). An acellular, allograft-derived meniscus scaffold in an ovine model. Arthroscopy J. Arthroscopic Related Surg. 26, 936–948. doi: 10.1016/j.arthro.2009.11.024
Storm, C., Pastore, J. J., MacKintosh, F. C., Lubensky, T. C., and Janmey, P. A. (2005). Nonlinear elasticity in biological gels. Nature 435, 191–194. doi: 10.1038/nature03521
Subbiah, R., and Guldberg, R. E. (2019). Materials science and design principles of growth factor delivery systems in tissue engineering and regenerative medicine. Adv. Healthcare Mater. 8, 1801000. doi: 10.1002/adhm.201801000
Suh, J.-K. F., and Matthew, H. W. (2000). Application of chitosan-based polysaccharide biomaterials in cartilage tissue engineering: a review. Biomaterials 21, 2589–2598. doi: 10.1016/s0142-9612(00)00126-5
Sun, J., Vijayavenkataraman, S., and Liu, H. (2017). An overview of scaffold design and fabrication technology for engineered knee meniscus. Materials 10:29. doi: 10.3390/ma10010029
Sun, Y., You, Y., Jiang, W., Wu, Q., Wang, B., and Dai, K. (2020). Generating ready-to-implant anisotropic menisci by 3D-bioprinting protein-releasing cell-laden hydrogel-polymer composite scaffold. Appl. Mater. Today 18:100469. doi: 10.1016/j.apmt.2019.100469
Sweigart, M., Zhu, C., Burt, D., DeHoll, P., Agrawal, C., Clanton, T., et al. (2004). Intraspecies and interspecies comparison of the compressive properties of the medial meniscus. Ann. Biomed. Eng. 32, 1569–1579. doi: 10.1114/b:abme.0000049040.70767.5c
Tan, G.-K., and Cooper-White, J. J. (2011). Interactions of meniscal cells with extracellular matrix molecules: towards the generation of tissue engineered menisci. Cell Adhesion Migration 5, 220–226. doi: 10.4161/cam.5.3.14463
Tarafder, S., Gulko, J., Kim, D., Sim, K. H., Gutman, S., Yang, J., et al. (2019). Effect of dose and release rate of CTGF and TGFβ3 on avascular meniscus healing. J. Orthop. Res. 37, 1555–1562. doi: 10.1002/jor.24287
Teng, C. P., Mya, K. Y., Win, K. Y., Yeo, C. C., Low, M., He, C., et al. (2014). Star-shaped polyhedral oligomeric silsesquioxane-polycaprolactone-polyurethane as biomaterials for tissue engineering application. NPG Asia Mater. 6:e142. doi: 10.1038/am.2014.102
Tian, G., Jiang, S., Li, J., Wei, F., Li, X., Ding, Y., et al. (2021). Cell-free decellularized cartilage extracellular matrix scaffolds combined with interleukin 4 promote osteochondral repair through immunomodulatory macrophages: in vitro and in vivo preclinical study. Acta Biomater. 127, 131–145. doi: 10.1016/j.actbio.2021.03.054
Tissakht, M., and Ahmed, A. (1995). Tensile stress-strain characteristics of the human meniscal material. J. Biomech. 28, 411–422. doi: 10.1016/0021-9290(94)00081-e
Tong, X., Pan, W., Su, T., Zhang, M., Dong, W., and Qi, X. (2020). Recent advances in natural polymer-based drug delivery systems. React. Funct. Polymers 148:104501. doi: 10.1016/j.reactfunctpolym.2020.104501
Twomey-Kozak, J., and Jayasuriya, C. T. (2020). Meniscus repair and regeneration: A systematic review from a basic and translational science perspective. Clin. Sports Med. 39, 125–163.
Van Den Bulcke, A. I., Bogdanov, B., De Rooze, N., Schacht, E. H., Cornelissen, M., and Berghmans, H. (2000). Structural and rheological properties of methacrylamide modified gelatin hydrogels. Biomacromolecules 1, 31–38. doi: 10.1021/bm990017d
Vårum, K. M., Myhr, M. M., Hjerde, R. J., and Smidsrød, O. (1997). In vitro degradation rates of partially N-acetylated chitosans in human serum. Carbohydr. Res. 299, 99–101. doi: 10.1016/s0008-6215(96)00332-1
Vedicherla, S., Romanazzo, S., Kelly, D. J., Buckley, C. T., and Moran, C. J. (2018). Chondrocyte-based intraoperative processing strategies for the biological augmentation of a polyurethane meniscus replacement. Connect. Tissue Res. 59, 381–392. doi: 10.1080/03008207.2017.1402892
Veiseh, O., Doloff, J. C., Ma, M., Vegas, A. J., Tam, H. H., Bader, A. R., et al. (2015). Size-and shape-dependent foreign body immune response to materials implanted in rodents and non-human primates. Nat. Mater. 14, 643–651. doi: 10.1038/nmat4290
Venkatesan, J., and Kim, S.-K. (2014). Nano-hydroxyapatite composite biomaterials for bone tissue engineering—a review. J. Biomed. Nanotechnol. 10, 3124–3140. doi: 10.1166/jbn.2014.1893
Venkatesan, J., Bhatnagar, I., Manivasagan, P., Kang, K.-H., and Kim, S.-K. (2015). Alginate composites for bone tissue engineering: a review. Int. J. Biol. Macromol. 72, 269–281. doi: 10.1016/j.ijbiomac.2014.07.008
Vrancken, A. C. T., Buma, P., and van Tienen, T. G. (2013). Synthetic meniscus replacement: a review. Int. Orthop. 37, 291–299.
Vrancken, A. C. T., Hannink, G., Madej, W., Verdonschot, N., van Tienen, T. G., and Buma, P. (2017). In Vivo performance of a novel, anatomically shaped, total meniscal prosthesis made of polycarbonate urethane: a 12-Month Evaluation in Goats. Am. J. Sports Med. 45, 2824–2834. doi: 10.1177/0363546517713687
Walker, P. S., and Erkman, M. J. (1975). The role of the menisci in force transmission across the knee. Clin. Orthop. Related Res. 109, 184–192. doi: 10.1097/00003086-197506000-00027
Wan, H., Zhao, X., Lin, C., Kaper, H. J., and Sharma, P. K. (2020). Nanostructured coating for biomaterial lubrication through biomacromolecular recruitment. ACS Appl. Mater. Interfaces 12, 23726–23736. doi: 10.1021/acsami.0c04899
Wang, Z., Han, L., Sun, T., Ma, J., Sun, S., Ma, L., et al. (2020). Extracellular matrix derived from allogenic decellularized bone marrow mesenchymal stem cell sheets for the reconstruction of osteochondral defects in rabbits. Acta Biomater. 118, 54–68. doi: 10.1016/j.actbio.2020.10.022
Wei, W., Ma, Y., Yao, X., Zhou, W., Wang, X., Li, C., et al. (2020). Advanced hydrogels for the repair of cartilage defects and regeneration. Bioact. Mater. 6, 998–1011. doi: 10.1016/j.bioactmat.2020.09.030
Wilke, H.-J., Heuer, F., Neidlinger-Wilke, C., and Claes, L. (2006). Is a collagen scaffold for a tissue engineered nucleus replacement capable of restoring disc height and stability in an animal model? Eur. Spine J. 15, 433–438. doi: 10.1007/s00586-006-0177-x
Williams, B. O., Vrancken, A. C. T., Madej, W., Hannink, G., Verdonschot, N., van Tienen, T. G., et al. (2015). Short term evaluation of an anatomically shaped polycarbonate urethane total meniscus replacement in a goat model. PLoS One 10:e0133138. doi: 10.1371/journal.pone.0133138
Yan, R., Chen, Y., Gu, Y., Tang, C., Huang, J., Hu, Y., et al. (2019). A collagen-coated sponge silk scaffold for functional meniscus regeneration. J. Tissue Eng. Regen. Med. 13, 156–173. doi: 10.1002/term.2777
Yang, Z., Li, H., Yuan, Z., Fu, L., Jiang, S., Gao, C., et al. (2020). Endogenous cell recruitment strategy for articular cartilage regeneration. Acta Biomater. 114, 31–52. doi: 10.1016/j.actbio.2020.07.008
Yu, Z., Lili, J., Tiezheng, Z., Li, S., Jianzhuang, W., Haichao, D., et al. (2019). Development of decellularized meniscus extracellular matrix and gelatin/chitosan scaffolds for meniscus tissue engineering. Biomed. Mater. Eng. 30, 125–132. doi: 10.3233/bme-191038
Yuan, X., Wei, Y., Villasante, A., Ng, J. J., Arkonac, D. E., and Chao, P.-h.G, et al. (2017). Stem cell delivery in tissue-specific hydrogel enabled meniscal repair in an orthotopic rat model. Biomaterials 132, 59–71. doi: 10.1016/j.biomaterials.2017.04.004
Yuan, Z., Liu, S., Hao, C., Guo, W., Gao, S., Wang, M., et al. (2016). AMECM/DCB scaffold prompts successful total meniscus reconstruction in a rabbit total meniscectomy model. Biomaterials 111, 13–26. doi: 10.1016/j.biomaterials.2016.09.017
Zamboni, F., Vieira, S., Reis, R. L., Oliveira, J. M., and Collins, M. N. (2018). The potential of hyaluronic acid in immunoprotection and immunomodulation: chemistry, processing and function. Progr. Mater. Sci. 97, 97–122. doi: 10.1016/j.pmatsci.2018.04.003
Zarif, M.-E. (2018). A review of chitosan-, alginate-, and gelatin-based biocomposites for bone tissue engineering. Biomater. Tissue Eng. Bull. 5, 97–109. doi: 10.33263/bteb534.097109
Zarrintaj, P., Manouchehri, S., Ahmadi, Z., Saeb, M. R., Urbanska, A. M., Kaplan, D. L., et al. (2018). Agarose-based biomaterials for tissue engineering. Carbohydr. Polym. 187, 66–84. doi: 10.1016/j.carbpol.2018.01.060
Zellner, J., Mueller, M., Xin, Y., Krutsch, W., Brandl, A., Kujat, R., et al. (2015). Dynamic hydrostatic pressure enhances differentially the chondrogenesis of meniscal cells from the inner and outer zone. J. Biomech. 48, 1479–1484. doi: 10.1016/j.jbiomech.2015.02.003
Zellner, J., Pattappa, G., Koch, M., Lang, S., Weber, J., Pfeifer, C. G., et al. (2017). Autologous mesenchymal stem cells or meniscal cells: what is the best cell source for regenerative meniscus treatment in an early osteoarthritis situation? Stem Cell Res. Ther. 8, 1–12.
Zhang, F., and King, M. W. (2020). Biodegradable polymers as the pivotal player in the design of tissue engineering scaffolds. Adv. Healthcare Mater. 9:1901358. doi: 10.1002/adhm.201901358
Zhang, H., Zhang, K., Zhang, X., Zhu, Z., Yan, S., Sun, T., et al. (2014). Comparison of two hyaluronic acid formulations for safety and efficacy (chase) study of knee osteoarthritis: a multicenter, randomized, double-blind, 26 week non-inferiority trial comparing durolane® to artz®. Osteoarthr. Cartilage 22:S397.
Zhang, W., Chen, J., Tao, J., Jiang, Y., Hu, C., Huang, L., et al. (2013). The use of type 1 collagen scaffold containing stromal cell-derived factor-1 to create a matrix environment conducive to partial-thickness cartilage defects repair. Biomaterials 34, 713–723. doi: 10.1016/j.biomaterials.2012.10.027
Zhang, Z.-Z., Chen, Y.-R., Wang, S.-J., Zhao, F., Wang, X.-G., Yang, F., et al. (2019). Orchestrated biomechanical, structural, and biochemical stimuli for engineering anisotropic meniscus. Sci. Transl. Med. 11:eaao0750. doi: 10.1126/scitranslmed.aao0750
Zhang, Z.-Z., Jiang, D., Ding, J.-X., Wang, S.-J., Zhang, L., Zhang, J.-Y., et al. (2016). Role of scaffold mean pore size in meniscus regeneration. Acta Biomater. 43, 314–326. doi: 10.1016/j.actbio.2016.07.050
Zhao, D., Zhu, T., Li, J., Cui, L., Zhang, Z., Zhuang, X., et al. (2021). Poly(lactic-co-glycolic acid)-based composite bone-substitute materials. Bioact. Mater. 6, 346–360. doi: 10.1016/j.bioactmat.2020.08.016
Zhou, H., and Lee, J. (2011). Nanoscale hydroxyapatite particles for bone tissue engineering. Acta Biomater. 7, 2769–2781. doi: 10.1016/j.actbio.2011.03.019
Zhou, H., Lawrence, J. G., and Bhaduri, S. B. (2012). Fabrication aspects of PLA-CaP/PLGA-CaP composites for orthopedic applications: a review. Acta Biomater. 8, 1999–2016. doi: 10.1016/j.actbio.2012.01.031
Zhou, X., Tenaglio, S., Esworthy, T., Hann, S. Y., Cui, H., Webster, T. J., et al. (2020). Three-dimensional printing biologically inspired DNA-based gradient scaffolds for cartilage tissue regeneration. ACS Appl. Mater. Interfaces 12, 33219–33228. doi: 10.1021/acsami.0c07918
Keywords: polymeric scaffold, natural polymer, synthetic polymer, meniscal tissue engineering, meniscal regeneration
Citation: Li H, Li P, Yang Z, Gao C, Fu L, Liao Z, Zhao T, Cao F, Chen W, Peng Y, Yuan Z, Sui X, Liu S and Guo Q (2021) Meniscal Regenerative Scaffolds Based on Biopolymers and Polymers: Recent Status and Applications. Front. Cell Dev. Biol. 9:661802. doi: 10.3389/fcell.2021.661802
Received: 31 January 2021; Accepted: 15 June 2021;
Published: 13 July 2021.
Edited by:
Stevo J. Najman, University of Niš, SerbiaReviewed by:
Hongmin Qin, Texas A&M University, United StatesDong Jiang, Peking University Third Hospital, China
Jianxun Ding, Changchun Institute of Applied Chemistry, Chinese Academy of Sciences, China
Copyright © 2021 Li, Li, Yang, Gao, Fu, Liao, Zhao, Cao, Chen, Peng, Yuan, Sui, Liu and Guo. This is an open-access article distributed under the terms of the Creative Commons Attribution License (CC BY). The use, distribution or reproduction in other forums is permitted, provided the original author(s) and the copyright owner(s) are credited and that the original publication in this journal is cited, in accordance with accepted academic practice. No use, distribution or reproduction is permitted which does not comply with these terms.
*Correspondence: Shuyun Liu, clear_ann@163.com; Quanyi Guo, doctorguo_301@163.com
†These authors have contributed equally to this work and share first authorship