Histone Arginine Methyltransferase CARM1-Mediated H3R26me2 Is Essential for Morula-to-Blastocyst Transition in Pigs
- 1Anhui Province Key Laboratory of Local Livestock and Poultry, Genetical Resource Conservation and Breeding, College of Animal Science and Technology, Anhui Agricultural University, Hefei, China
- 2Reproductive Medicine Center, The 901st Hospital, Hefei, China
- 3Reproductive and Genetic Branch, The First Affiliated Hospital of University of Science and Technology of China, Hefei, China
Coactivator-associated arginine methyltransferase 1 (CARM1) is involved in both establishment of first pluripotent lineage and pluripotency maintenance of embryonic stem cells (ESCs) in mice. However, the histone substrates and role of CARM1 in early embryonic development remain largely unknown. Here, we show that CARM1 specifically catalyzes H3R26me2 to promote porcine blastocyst formation. The putative histone substrates of CARM1, including H3R2me2, H3R17me2, and H3R26me2, are present in pig early embryos. The changes of CARM1 mRNA during early embryogenesis parallel that of H3R26me2. Functional studies using a combinational approach of chemical inhibition and RNA interference (RNAi) showed that catalytic activity inhibition of CARM1 protein or knockdown (KD) of CARM1 mRNA did not alter the levels of both H3R2me2 and H3R17me2, but significantly reduced H3R26me2 levels in porcine embryos. Furthermore, CARM1 inhibition or KD did not affect embryo development to the 2-cell, 4-cell, 8-cell, and morula stages, but severely compromised blastocyst development. CARM1 knocked down embryos that developed to the blastocyst stage had fewer total cells, inner cell mass (ICM), and trophectoderm (TE) cells. Mechanistically, single embryo RNA-sequencing analysis revealed that CARM1 KD altered the transcriptome characterized by downregulation of key genes associated with Hippo and PI3K-AKT signaling pathways. Taken together, these results demonstrate that CARM1 specifically catalyzes H3R26me2 in porcine embryos and participates in blastocyst development.
Introduction
The blastocyst formation is an important cellular event during preimplantation embryonic development. Concomitantly, the first cell segregation generates pluripotent and differentiating lineages, leading to the formation of ICM and TE in blastocysts (Chazaud and Yamanaka, 2016; White and Plachta, 2020). The first lineage specification is tightly regulated by the restricted expression of master transcription factors (Rossant, 2018) and signaling pathways (Menchero et al., 2017). Epigenetic regulation on the chromatin is also critical for establishing lineage-specific gene expression during early embryogenesis. Accumulating studies showed that methylation on the DNA (Nakanishi et al., 2012) and histone lysine residues (Gao et al., 2010; Liu et al., 2016), and accessible chromatin landscape (Yang et al., 2020) present asymmetrical distribution between ICM and TE in blastocysts and play essential roles in the lineage specification. However, epigenetic regulation of the first lineage specification with histone arginine methylation remains poorly understood.
CARM1 (also known as PRMT4) is recognized as a transcriptional activator and belongs to the family of protein arginine methyltransferase. CARM1 usually mediates asymmetric arginine dimethylation on both histone tails and non-histone proteins (Shishkova et al., 2017; Jarrold and Davies, 2019). It was reported that CARM1 mainly catalyzes the dimethylation at histone H3 arginine 2 (H3R2), 17 (H3R17), and 26 (H3R26) (Schurter et al., 2001). It is worth noting that CARM1 preferentially methylates H3R17 over H3R26 in a cell type specific manner (Jacques et al., 2016). In addition, CARM1 can catalyze H3R17me2 in mouse early embryos (Torres-Padilla et al., 2007), but not in mouse embryonic fibroblasts (Cheng et al., 2020). This implies that CARM1’s option for histone arginine substrates has context-dependent effects. Considering the unique consequences of each methylargine isoform, it is necessary to determine CARM1’s histone substrate under a specific species or cellular context.
At the molecular level, CARM1-mediated histone dimethylation has been implicated in RNA transcription, pre-mRNA splicing, mRNA translation, and genome stability (Lorton and Shechter, 2019). CARM1 is also involved in several cellular processes, including pluripotency maintenance of ESCs (Wu et al., 2009), differentiation of epithelial cells (O’Brien et al., 2010), and spermatogenesis (Bao et al., 2018). In mouse blastocysts, ICM cells express a higher level of CARM1 protein relative to TE cells (Sun et al., 2020). Maternal and zygotic CARM1-deficient embryos could develop into blastocysts that contained fewer ICM cells (White et al., 2016; Xia et al., 2018). Correspondingly, CARM1 overexpression-induced H3R26me2 is sufficient to contribute blastomere progeny to the ICM lineage in blastocysts (Torres-Padilla et al., 2007). Endogenous CARM1 in mouse embryos suppresses the expression of trophectodermal genes to ensure ICM lineage specification (Parfitt and Zernicka-Goetz, 2010). Intriguingly, a recent study by base editing in mouse embryos showed that CARM1-catalyzed H3R17me2 is required for blastocyst formation (Yang et al., 2019). However, the role of CARM1-mediated H3R26me2 in TE and blastocyst development remains largely unclear.
Given the similarity of reproductive physiology, embryonic developmental timing in pigs to humans (Mordhorst and Prather, 2017; Alberio, 2020), studies in the pig may be informative for understanding the development of human embryos. In the present study, we investigated the role and potential mechanisms of CARM1 in porcine early embryonic development. We found that CARM1 specifically recognizes H3K26me2 in porcine early embryos. Functional studies using RNAi reveal that CARM1-mediated H3R26me2 is indispensable for both lineage specification and blastocyst formation. Single-embryo RNA sequencing analysis demonstrate that CARM1 regulates the expression of key genes required for lineage specification. Our findings provide new insights into the role of CARM1-catalyzed H3R26me2 in porcine blastocyst development.
Materials and Methods
All reagents used were purchased from Sigma (Sigma-Aldrich, St Louis, MO) unless otherwise stated. Animal experiments were conducted in accordance with the Institutional Animal Care and Use Committee (IACUC) guidelines under current approved protocols at Anhui Agricultural University.
Preparation of CARM1 Inhibitor
CARM1 inhibitor (Millipore, 217531) was dissolved in DMSO (Sigma, D2650) and stored at −20°C. Embryo culture medium was used to dilute the stock solution to obtain the desired working solution. The same volume of DMSO was added into the medium as a control when the chemical was used.
Oocyte in vitro Maturation
Ovaries were collected from a local slaughterhouse. Follicular fluid was aspirated from antral follicles at 3–6 mm in diameter. Cumulus-oocyte complexes (COCs) were selected under a stereomicroscope. Subsequently, COCs were cultured in one well of 4-well plate containing 400 μL in vitro maturation medium for 44 h at 38.5°C, 5% CO2 and saturated humidity. Cumulus cells surrounding oocytes was removed using 1 mg/mL hyaluronidase following maturation.
Parthenogenetic Activation (PA)
Oocytes at metaphase II stage were stimulated using two pulses of direct current (1.56 kV/cm for 80 ms) in activation medium. Subsequently, the activated oocytes were washed three times in the porcine zygote medium (PZM-3) medium and were incubated in the chemically assisted activation medium for 4 h. Then, embryos were cultured in PZM-3 droplets at 38.5°C, 5% CO2 and 95% air with saturated humidity.
In vitro Fertilization (IVF)
Oocytes at the metaphase II were washed in the modified Tris-buffered medium (mTBM) containing 2 mg/mL BSA and 2 mM caffeine. The oocytes were incubated in mTBM for 4 h at 38.5°C in 5% CO2 in air. Semen of two boars was mixed and centrifuged at 1,900 g for 4 min in DPBS supplemented with 1 mg/mL BSA. Following the removal of supernatant, the sperm concentration was adjusted with mTBM to 1 × 106 sperms/mL. The semen was then added to the mTBM droplets containing oocytes. After co-incubation of oocyte and sperm for 6 h, sperms surrounding oocytes were washed out and presumptive zygotes were cultured in PZM-3 at 38.5 C in 5% CO2 in air.
In vitro Transcription
CARM1-mCherry mRNA used for microinjection was synthesized in vitro. pIVT- CARM1-mCherry plasmid containing T7 promoter was linearized by digestion with BspQI. Linearized DNA templates were purified using a DNA clean and concentrator Kit (ZYMO RESEARCH, D4003, Tustin, CA, United States). According to the manufacture’s manual, in vitro transcription of CARM1-mCherry mRNA was performed through using mMESSAGE MACHINE TM T7 kit (Ambion, AM1344, shanghai, China) and Poly (A) Tailing Kit (Ambion, AM1350, Shanghai, China). Then, mRNA was treated with TURBO DNase to remove the DNA templates and was further purified using MEGAclear Kit (Ambion, AM1908, Shanghai, China). After mRNA was dissolved in RNase-free water, mRNA concentration was determined by a NanoDrop instrument (Thermo Fisher Scientific, Shanghai, China) and was then aliquoted and stored at −80°C.
Real-Time Quantitative Polymerase Chain Reaction (qPCR)
Total RNA was extracted from oocytes and embryos using RNeasy Mini Kit (Qiagen, 74104). RNA was transcriptionally reversed into cDNA using QuantiTect Reverse Transcription Kit (Qiagen, 205311). The primers used in this study were listed in Supplementary Table 1. The assembly of polymerase chain reaction was prepared in FastStart SYBR Green Master (Roche, 04673514001) and was run on StepOne Plus (Applied Biosystems, Foster, United States). The samples were collected three times and three biological replicates were conducted for each gene. EF1A1 was used as the internal reference gene. The Cq values were obtained and analyzed using the 2–ΔΔCt method.
Immunofluorescence Staining
Embryos were fixed in 4% paraformaldehyde solution for 15 min, permeabilized with 1% Triton X-100 for 30 min at room temperature (RT) and then blocked with 2% BSA at RT for 1 h. Samples were incubated in solution containing primary antibodies overnight at 4°C. Following washing, samples were incubated for 1 h in solution containing secondary antibodies in the dark at 37°C. Following washing, samples were counterstained using 4, 6-diamidino-2-phenylindole dihydrochloride or propidium iodide for 10 min and were then loaded onto glass slides. Finally, samples were imaged using laser scanning confocal microscopy (Olympus, Japan). Information regarding primary and secondary antibodies used was provided in Supplementary Table 2.
Microinjection
siRNA species was designed to target three different sites of the porcine CARM1 coding region (GenePharma, Shanghai, China). Microinjection was performed in a T2 (TCM199 with 2% FBS) medium containing 7.5 μg/mL Cytochalasin B on an inverted microscope (Olympus, Japan). Approximately 10 pl of siRNA solution (50 μM) was microinjected into the cytoplasm of MII oocytes. Embryos were cultured in PZM-3 medium for 7 days.
Single-Embryo RNA Sequencing
Single embryo at day 5 (non-injected and CARM1-siRNA injected embryos) was collected for RNA-seq analysis. RNA was extracted using the RNeasy Mini Kit (Qiagen, 74104). Pre-amplified cDNA was fragmented using Fragmentase (NEB, M0348S) via the incubation at 37°C for 20 min. cDNA libraries were constructed by TruSeq Nano DNA LT Library Preparation Kit (FC-121-4001). Then, the libraries were sequenced on the Illumina HiSeq 2500 instrument (LC-Sciences, Hangzhou, China). The reads were mapped to the pig reference genome. Differential gene expression between non-injectedand CARM1 siRNA injected embryos was determined using Cufflinks. The threshold for significance was a false discovery rate ≤0.05 and a fold expression change ≥2. GO analysis was performed using DAVID Bioinformatics Resources 6.8. RNA-seq data are presented in Supplementary Tables 3–5.
Statistical Analysis
Statistical analyses were performed using one-way ANOVA or Student’s t-test (SPSS 17.0). All experiments were carried out at least three times and were presented as mean ± standard error of mean (mean ± S.E.M). P < 0.05 was considered to be statistically significant.
Results
Characterization of CARM1 mRNA and Potential Histone Substrates in Early Embryos
To quantify the relative abundance of CARM1 mRNA in early embryos, qPCR was performed to determine the expression of CARM1 mRNA. The results revealed that CARM1 is expressed in oocytes and embryos, but the expression levels of CARM1 mRNA are significantly higher in embryos at the 4-cell and 8-cell stages compared to oocytes, morulae, and blastocysts (Figure 1A) (P < 0.05). Furthermore, immunofluorescence staining was performed to determine the localization of CARM1’s putative histone substrates including H3R2me2, H3R17me2, and H3R26me2 in porcine embryos. The specificity of the arginine dimethylation antibodies in porcine blastocysts had been verified before undergoing immunostaining experiment (Supplementary Figure 1A). Immunofluorescence analysis showed that the dimethylation modifications of three histone arginine residues simultaneously localize at the cytoplasm and nuclei of both GV and MII oocytes whereas they are present in nuclei throughout early embryonic development (Figures 1B–D). Additionally, the dimethylation modifications of three histone arginine residues are also present in both the ICM and TE cells of blastocysts (Figures 1B–D). Therefore, these results indicate that CARM1 mRNA and its putative dimethylation modifications of histone arginine residues are dynamically present in early embryonic development.
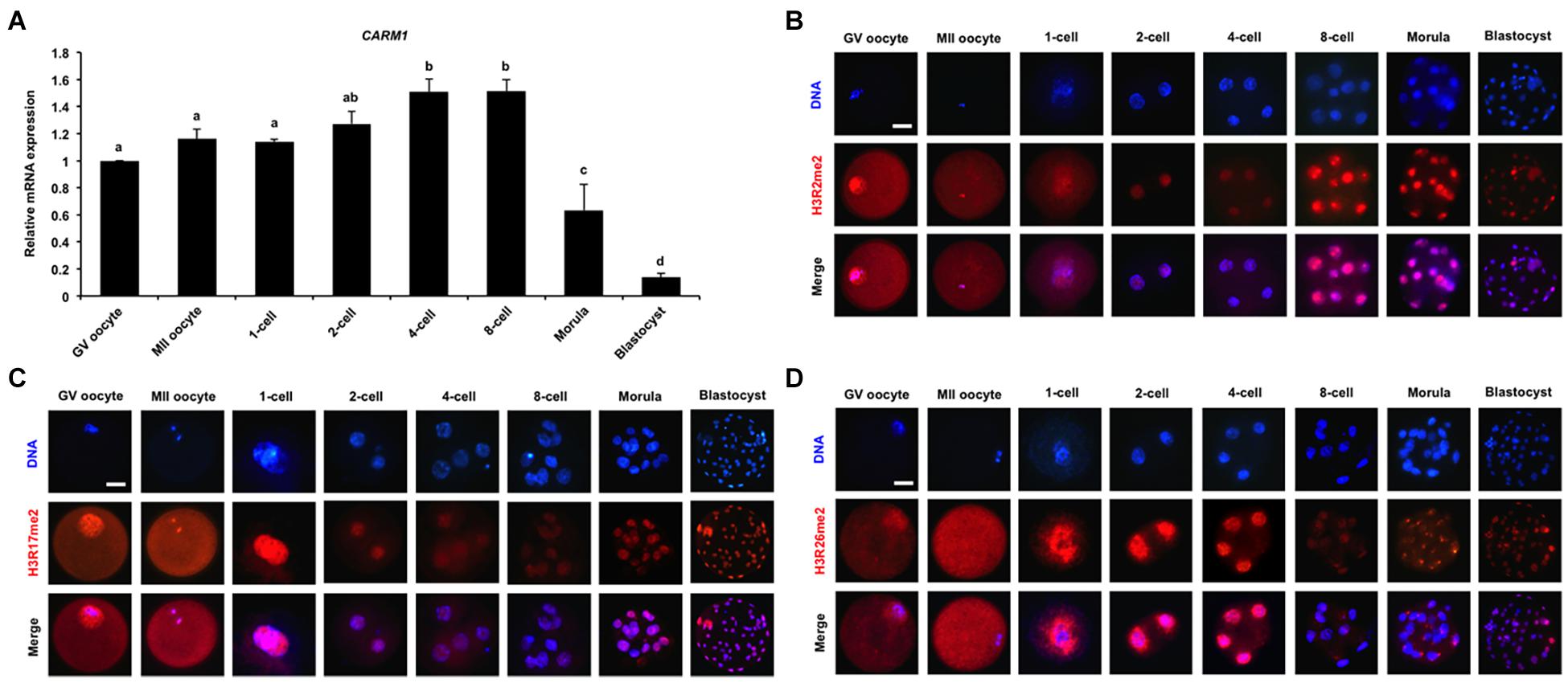
Figure 1. Dynamics of CARM1 mRNA and putative histone substrates in early embryos. (A) Expression of CARM1 mRNA in porcine early embryos. Relative abundance of CARM1 mRNA was determined by qPCR. Data were normalized to the reference gene (EF1α1) and the data from GV oocytes were set as 1. GV, germinal vesicle; MII, metaphase II. Data are shown as mean ± S.E.M and different letters on the bars indicate significant differences (P < 0.05). Changes of H3R2me2 (B), H3R17me2 (C), H3R26me2 (D) levels in early embryonic development. Embryos were stained for H3R2me2, H3R17me2, H3R26me2 (red), and DNA (blue). Representative images obtained by confocal microscopy are shown. The experiment was independently repeated three times with at least 30 embryos per group. Scale bar: 50 μm.
Chemical Inhibition of CARM1 Activity Blocks Blastocyst Formation and Reduces H3R26me2 Levels in Early Embryos
To determine the specific histone arginine substrates of CARM1 in porcine embryos, a chemical inhibitor with different concentrations (9, 18, and 27 μM) was used to treat embryos. The developmental results revealed that treatment of 9 μM CARM1 inhibitor did not affect the developmental rates of early embryos (Figures 2A,B and Supplementary Figures 2A,B). Treatment of 18 μM CARM1 inhibitor partially hindered the development of 2-cell to morula whereas it significantly reduced the blastocyst rates (Figures 2A,B and Supplementary Figures 2A,B) (P < 0.05). Treatment of 27 μM CARM1 inhibitor significantly blocked the development of 2-cell to blastocyst stages (Figures 2A,B and Supplementary Figures 2A,B) (P < 0.05). Considering the developmental phenotypes of embryos exposed to different concentrations of CARM1 inhibitor, the dosage of 27 μM was used in the subsequent experiments. To further determine the levels of H3R2me2, H3R17me2, and H3R26me2, immunofluorescence staining was performed in embryos at the 2-cell, 4-cell, and blastocyst stages. The results showed that CARM1 inhibition did not affect H3R2me2 and H3R17me2 levels, but significantly reduced H3R26me2 levels in embryos at the indicated stages (Figures 2C–E and Supplementary Figures 2C,D) (P < 0.05). Therefore, these data demonstrate that CARM1 activity is required for blastocyst formation and the generation of H3R26me2 modification in porcine embryos.
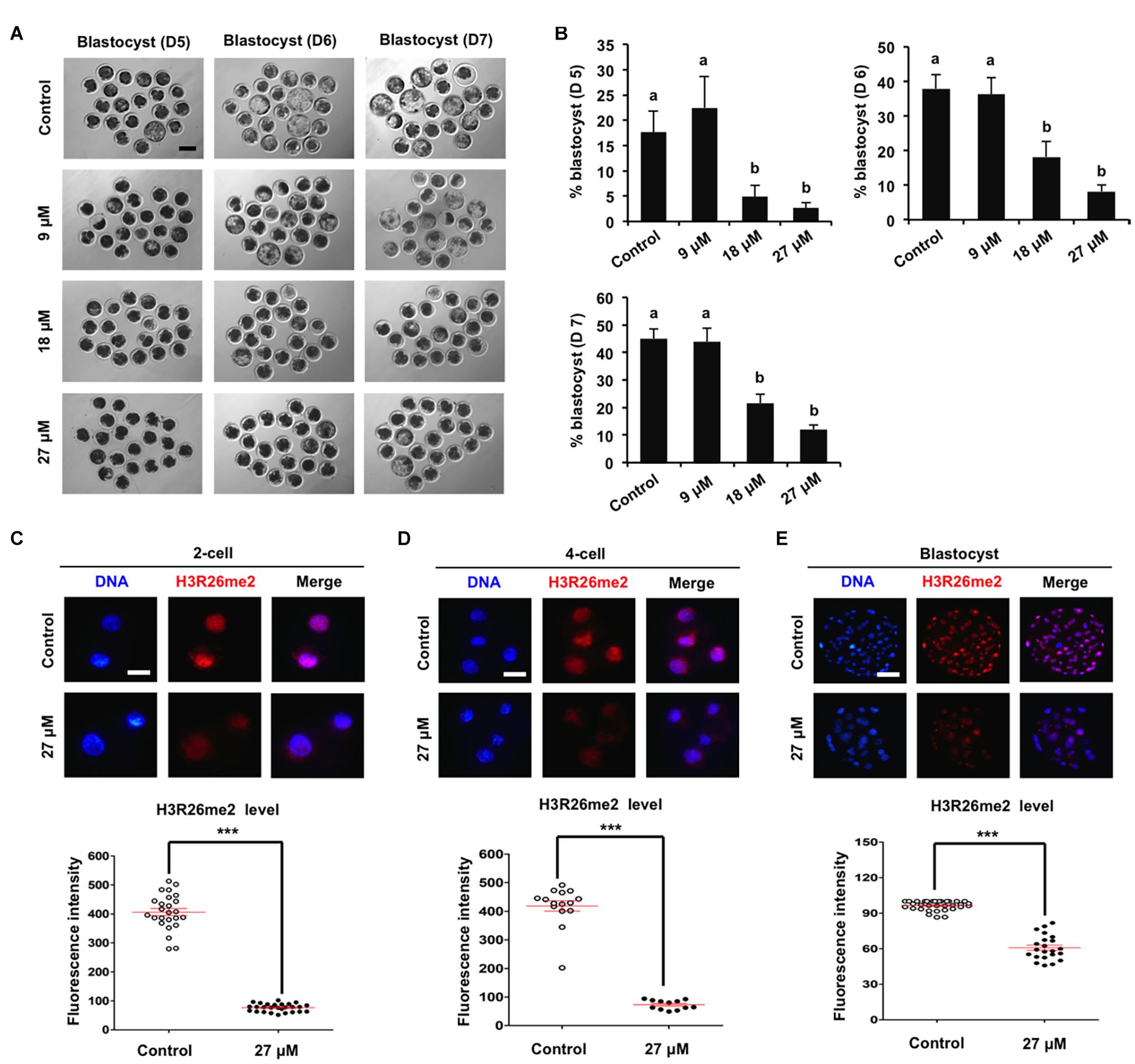
Figure 2. Effect of CARM1 inhibition on blastocyst development and H3R26me2 levels in embryos. (A) Representative images of blastocysts at days 5, 6, and 7 from control and treatment groups. Scale bar: 100 μm. (B) Effect of treatment of CARM1 inhibitor with different concentrations on blastocyst rates. Effect of CARM1 inhibition on H3R26me2 levels in 2-cell (C), 4-cell (D), and blastocysts (E). Embryos at different stages were stained for H3R26me2 (red) and DNA (blue). The experiment was independently repeated three times with at least 12 embryos per group. Scale bar: 50 μm. All data are shown as mean ± S.E.M and different letters on the bars or asterisks indicate significant differences (P < 0.05).
siRNA Injection Efficiently Attenuates Expression of CARM1 mRNA and Protein and Specifically Decreases H3R26me2 Levels in Early Embryos
To further confirm whether CARM1 protein specifically recognizes the H3R26 residue in porcine embryos, RNAi approach was used to delete the CARM1 protein. MII oocytes were microinjected with CARM1 siRNA or negative control (NC) siRNA. Uninjected oocytes served as control groups. A subset of embryos at the 4-cell and 8-cell stage was subject to qPCR to detect the expression levels of CARM1 mRNA. The results revealed that siRNA injection significantly reduced the levels of CARM1 mRNA in embryos at the 4-cell (Figure 3A) and 8-cell (Figure 3B) stage compared to the control groups (P < 0.05). No differences in expression levels of CARM1 mRNA were observed between NC group and uninjected group (Figures 3A,B). There are not available for porcine specific CARM1 antibodies so that we could not directly evaluate the levels of CARM1 protein in embryos. Instead, we constructed the fusion expression vector of CARM1-mCherry and synthesized in vitro the fusion mRNA. Both CARM1-mCherry mRNA and siRNA was injected into oocytes and served as the experimental group, uninjected, mCherry mRNA or CARM1-mCherrymRNA injection served as the control groups. Fluorescence intensity analysis showed that CARM1 siRNA injection significantly reduced the levels of CARM1-mCherry protein at the 4-cell stage compared to the control groups (Figure 3C) (P < 0.05). Next, immunofluorescence staining was performed to evaluate whether CARM1 KD affected the levels of H3R2me2, H3R17me2, and H3R26me2 in embryos. As shown in Supplementary Figures 3A,B, CARM1 KD did not alter the levels of both H3R2me2 and H3R17me2 in embryos at the 2-cell, 4-cell, and blastocyst stage. However, CARM1 KD significantly decreased H3R26me2 levels in embryos at the 2-cell (Figure 3D), 4-cell (Figure 3E), and blastocyst (Figure 3F) stage compared to the control groups (P < 0.05). Collectively, these results document that CARM1 protein specifically catalyzes H3R26me2 in porcine early embryos.
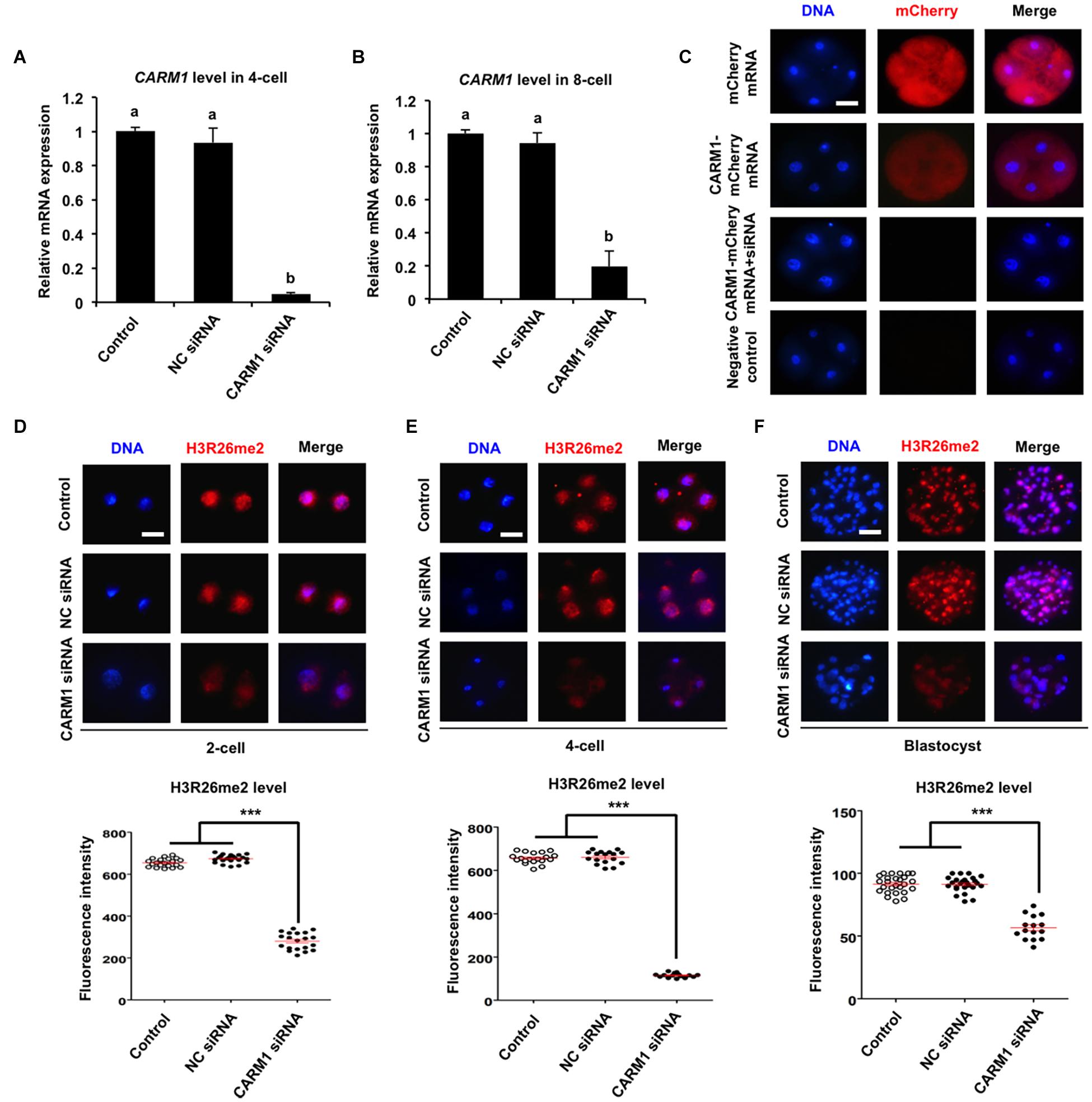
Figure 3. Effect of siRNA injection on CARM1 expression and H3R26me2 levels in embryos. Expression levels of CARM1 mRNA in 4-cell (A) and 8-cell (B) from control, NC siRNA injection, and CARM1 siRNA injection were determined by qPCR. NC, negative control. (C) Expression of CARM1 protein in 4-cell embryos. Embryos from the indicated groups were imaged for mCherry (red) and DNA (blue) by confocal microscopy and the representative images are shown. The experiment was independently repeated three times with at least 20 embryos per stage. Scale bar: 50 μm. Effect of siRNA injection on H3R26me2 levels in 2-cell (D), 4-cell (E), and blastocysts (F). Embryos at different stages were stained for H3R26me2 (red) and DNA (blue). The experiment was independently repeated three times with at least 15 embryos per group. Scale bar: 50 μm. All data are shown as mean ± S.E.M and different letters on the bars or asterisks indicate significant differences (P < 0.05).
CARM1 Knockdown Impedes Blastocyst Formation and Disrupts Normal Lineage Allocation
To elucidate whether CARM1 KD affected embryo development, the developmental rates of PA embryos at each stage were subject to statistical analysis. We found that CARM1 KD had no effect on embryo development to 2-cell, 4-cell, 8-cell, and morula stage (Supplementary Figures 3C,D), but reduced the blastocyst rates (Days 5–7) compared to the control groups (Figures 4A,B) (P < 0.05). Additionally, we did not observe differences in the rates of embryo development between NC siRNA injected and uninjected control groups (Figures 4A,B and Supplementary Figures 3C,D). To confirm the specificity of CARM1 KD, rescue experiments were performed by coinjection of CARM1-mCherry mRNA and CARM1 siRNA in oocytes. The results revealed that coinjection of CARM1-mCherry mRNA and CARM1 siRNA could restore blastocyst formation of CARM1 KD embryos (Figures 4C,D), demonstrating a specific role of CARM1 in early embryonic development. To determine whether CARM1 KD impaired lineage allocation in blastocysts, embryos were stained with a CDX2 antibody to determine the TE cell number (Figure 4E). The number of ICM cells was indirectly determined by subtracting the TE number from the total cell number. The results showed that CARM1 KD resulted in a significant reduction in the number of total cells, ICM and TE cells (Figure 4F) (P < 0.05). However, the ratio of ICM cells to TE cells in the CARM1 KD blastocysts was similar to that in the control groups (Figure 4F). Similarly, CARM1 KD did not impair the development of IVF embryos before the morula stage (data not shown), while CARM1 KD led to a significant reduction in both embryos that developed to blastocyst stage (days 5–7) (Supplementary Figures 4A,B) and lineage cells of IVF blastocysts (Supplementary Figures 4C,D) (P < 0.05). Hence, these results indicate that CARM1 is indispensable for blastocyst formation and normal lineage allocation.
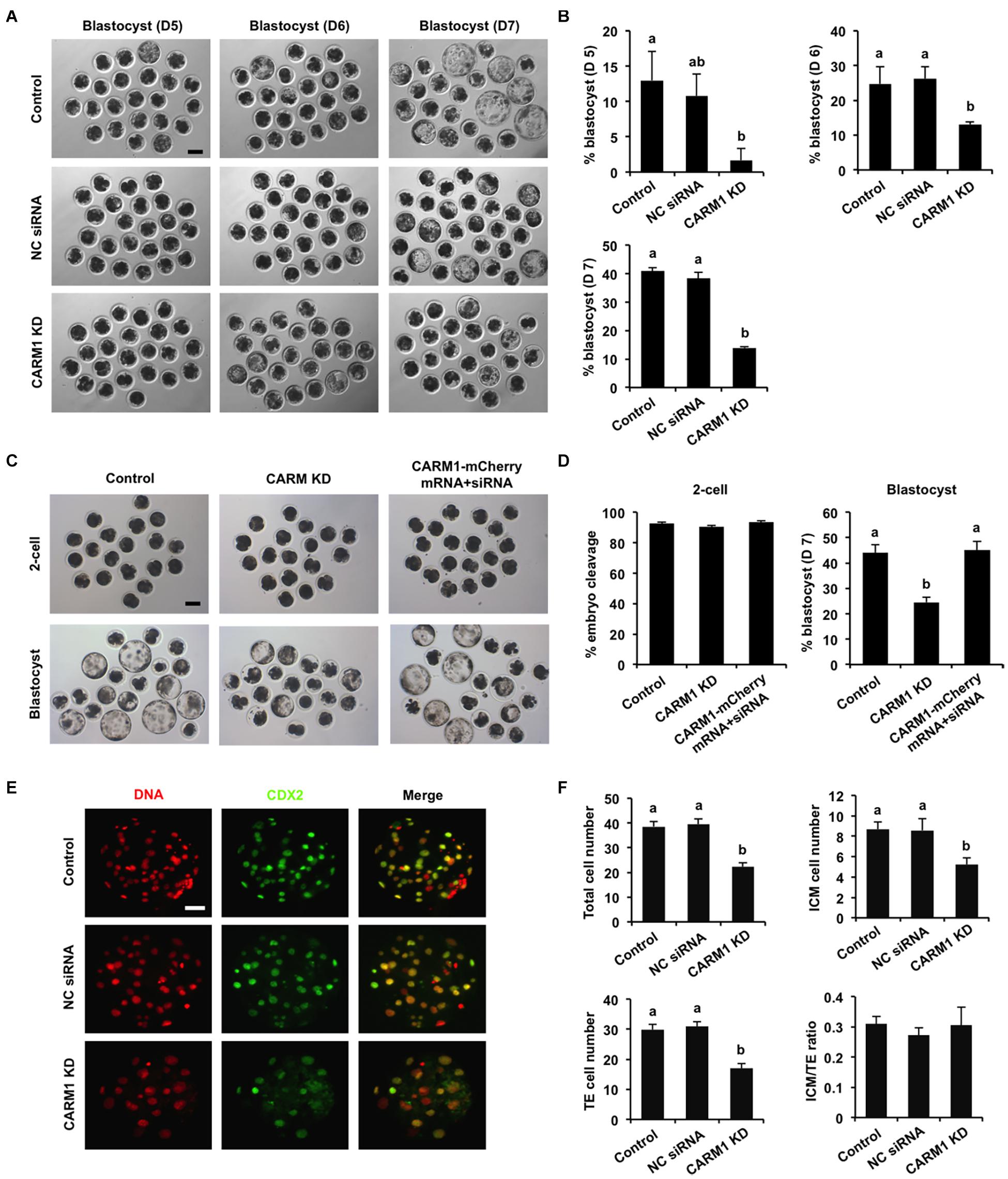
Figure 4. Effect of CARM1 knockdown on blastocyst development and lineage allocation. (A) Representative images of blastocysts from different stages at days 5, 6, and 7. Scale bar: 100 μm. (B) Analysis of blastocyst rates at days 5, 6, and 7. (C) Representative images of 2-cell embryos and blastocysts. Scale bar: 100 μm. (D) Analysis of cleavage and blastocyst rates. (E) Representative fluorescence images of blastocysts. Embryos at different groups were stained for CDX2 (green) and DNA (red). The experiment was independently repeated three times with at least 20 blastocysts per group. Scale bar: 50 μm. (F) Analysis of lineage allocation in blastocysts. The numbers of total cells, ICM cells, TE cells, and the ratio of ICM cells to TE cells were recorded and subject to statistical analysis. ICM: inner cell mass; TE: trophectoderm. All data are shown as mean ± S.E.M and different letters on the bars indicate significant differences (P < 0.05).
CARM1 Knockdown Alters the Global Transcriptome in Early Embryos
To decipher the molecular mechanisms of CARM1 regulating embryo deve0lopment, single-embryo RNA sequencing was utilized to characterize tanscriptomic changes in CARM1 KD embryos. To validate the single-embryo RNA sequencing data, the expression levels of 6 differentially expressed genes (DEGs) (3 downregulated and 3 upregulated genes), namely ID2, CCND2, CARM1, PLK5, PPP2R2C, and CCNA1, were analyzed by qPCR. The expression patterns of these selected genes are highly consistent with the treads obtained from RNA sequencing data (Supplementary Figure 5), suggesting the robustness of the results obtained by RNA sequencing. We identified a total of 154 DEGs between CARM1 KD and uninjected control groups, of which there were 89 down-regulated genes and 65 up-regulated genes, respectively (Figure 5A and Supplementary Table 3). Furthermore, GO analysis was performed to annotate the potential function of the DEGs in embryo development. The DEGs were classified into three main categories (biological process, cellular component, and molecular function) according to the GO database. The top-ranking 10 biological processes, 10 cellular components, and 10 molecular functions involved in each GO term were provided (Figure 5B and Supplementary Table 4) (P < 0.05), such as positive regulation of cell population proliferation (10 genes, e.g., AREG, CCNA1, FGF19, CARM1, CCND2), negative regulation of epithelial cell proliferation (4 genes, e.g., WNT10B, GPC3), cell adhesion (4 genes, e.g., ADAM8, TGFBI). Lastly, KEGG analysis revealed that the DEGs were mainly enriched in 10 significant pathways (Figure 5C and Supplementary Table 5) (P < 0.05), such as Hippo signaling pathway (6 genes, e.g., WNT10B, ID2, PPP2R2C, AREG, ITGB2, CCND2), PI3K-Akt signaling pathway (6 genes, e.g., AREG, FGF19, EFNA4, CCND2, CCNA1). Altogether, these data demonstrate that CARM1 regulates the expression of key genes important for blastocyst formation.
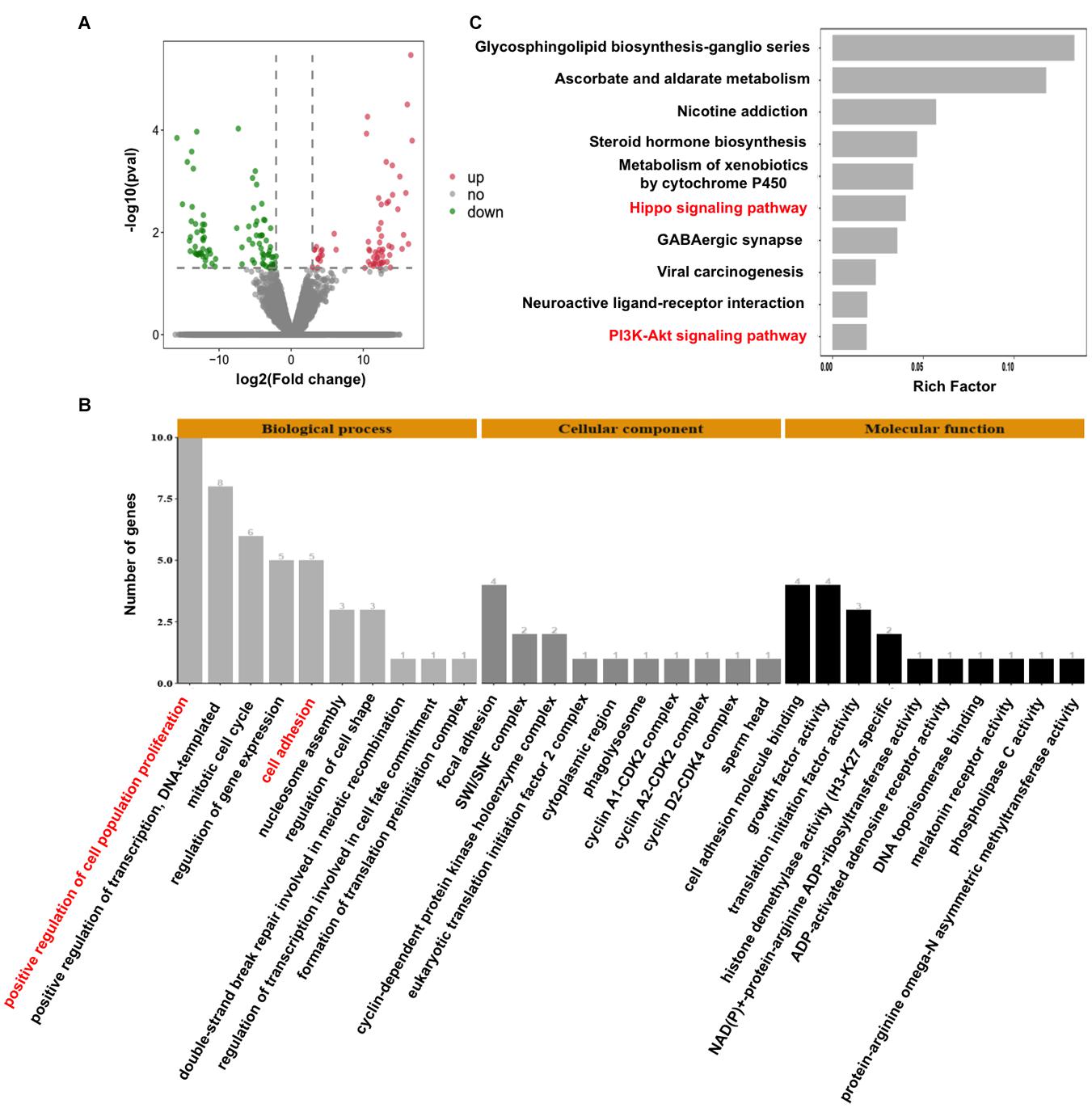
Figure 5. Effect of CARM1 knockdown on the transcriptome in embryos. (A) Volcano plot displaying differentially expressed genes in embryos from control and CARM1 KD groups. Green spots denote the number of downregulated genes; red spots indicate the number of upregulated genes. (B) GO analysis showing the most enriched functional categories enriched by the differentially expressed genes. (C) KEGG analysis showing the top 10 signaling pathways enriched by the differentially expressed genes.
Discussion
Although regulatory mechanisms of CARM1-mediated H3R26me2 underlying the ICM lineage specification have been well studied (Parfitt and Zernicka-Goetz, 2010; White et al., 2016; Xia et al., 2018), the role of CARM1-catalyzed histone arginine dimethylation in the TE lineage specification and blastocyst development remains largely unknown. Here, we show that CARM1 specifically catalyzes H3R26me2, but not H3R2me2 and H3R17me2 in porcine early embryos. CARM1-mediated H3R26me2 facilitates lineage specification and blastocyst formation. Mechanistically, CARM1 regulates the expression of multiple genes required for cell proliferation and lineage specification. Therefore, our data demonstrate that CARM1-mediated H3R26me2 participates in porcine blastocyst formation.
Histone arginine methylation encompasses diverse isoforms at different residues and each methylation isoform presents unique function in a specific biological process (Kirmizis et al., 2007; Torres-Padilla et al., 2007). It is well recognized that CARM1 can catalyze H3R2me2, H3R17me2, and H3R26me2 in a specific cell type (Schurter et al., 2001), but the option of CARM1 for histone substrates frequently has context-dependent effects (Price and Hevel, 2020). Studies showed that CARM1 can simultaneously catalyze the dimethylation at R2, R17, and R26 in mouse early embryos (Torres-Padilla et al., 2007), but it does not recognize the H3R17me2 substrate in mouse embryonic fibroblasts (Cheng et al., 2020). In this study, we found that CARM1 specifically catalyzes H3R26me2, but not H3R2me2 and H3R17me2 in porcine early embryos. The discrepancy in the recognition of CARM1 substrates in early embryos could be due to species differences. The development of early embryos exhibits a unique feature between mice and pigs (Alberio, 2020), which might cause a different requirement for CARM1’s histone substrates. Since CARM1 also catalyzes non-histone proteins in some cell types (Shishkova et al., 2017), we could not rule out the possibility that CARM1 targets other arginine substrates in porcine early embryos.
Accumulating evidences indicated that epigenetic regulation plays a critical role in the lineage specification of mammalian blastocyst (Paul and Knott, 2014; Fu et al., 2020). The results in this study showed that CARM1 KD led to a significant reduction in the number of ICM and TE cells, suggesting that CARM1 regulates lineage allocation in porcine blastocysts. In mouse preimplantation embryos, CARM1-mediated H3R26me2 limits trophectodermal features to bias blastomeres toward ICM lineage (Torres-Padilla et al., 2007). Similarly, our transcriptomic analysis revealed that CARM1 regulates Hippo signaling pathway in porcine embryos. Disruption of Hippo signaling leads to the misspecification of the ICM lineage in several species (Nishioka et al., 2009; Negron-Perez and Hansen, 2018; Cao et al., 2019). Thus, we speculate that CARM1 may activate Hippo signaling to facilitate ICM lineage specification in pigs. On the other hand, CARM1 is required for differentiation of epithelial cells (O’Brien et al., 2010) and enforced expression of CARM1 promoted CDX2 transcription and increased TE cell number in murine cloned blastocysts (Bang et al., 2018). It is thus likely that CARM1 elevates the expression of trophectoderm genes to promote TE lineage specification. Altogether, these results lead us to reason that CARM1 may exert differential mechanisms to allow the first lineage specification in porcine blastocysts.
Whether CARM1 is essential for blastocyst formation in mice has not reached consistent conclusions (White et al., 2016; Yang et al., 2019), but our data document that CARM1 is indispensable for porcine blastocyst development. A number of signaling pathways have been shown to be essential for blastocyst formation in mammals (Maekawa et al., 2005; Nakasato et al., 2006; Jiao et al., 2020). In this study, single-embryo transcriptomic analysis revealed that CARM1 is implicated in both Hippo and PI3K-AKT signaling pathways, in which amphiregulin (AREG) was downregulated in CARM1 KD embryos. Previous studies established that AREG peptide supplementation elevated blastocyst rates in mice (Richani et al., 2013) and pigs (Prochazka et al., 2011). In addition, inhibition of Akt activity blocked murine blastocyst formation (Riley et al., 2005), and inactivation of PI3K signaling also prevented porcine blastocyst development (Jiao et al., 2020). Together, these data demonstrate that CARM1 in porcine embryos facilitates blastocyst formation via modulating the expression of key genes involved in signaling pathways. Future chromatin immunoprecipitation (ChIP) studies in pig embryos are warranted to confirm whether CARM1 regulation on these genes depends on H3R26me2.
Conclusion
In conclusion, our findings demonstrate that CARM1-mediated H3R26me2 is required for blastocyst development in pigs. Our results may provide new insights into the regulatory mechanism of CARM1-catalyzed H3R26me2 in porcine blastocyst development. These findings will be instrumental in developing novel strategies to improve developmental competence of porcine in vitro production embryos.
Data Availability Statement
The data presented in the study are deposited in the GEO repository, accession number (GSE173965).
Ethics Statement
Animal experiments were conducted in accordance with the Institutional Animal Care and Use Committee (IACUC) guidelines under current approved protocols at Anhui Agricultural University.
Author Contributions
ZC and YZ designed the research and wrote the manuscript. XT, HY, NZ, XZ, MZ, XW, QL, and YY performed the experiments. XT, HY, NZ, and XZ analyzed the data. YM, TY, and YL revised the manuscript. All authors read and approved the final version of manuscript.
Funding
This work was supported by the grants from the Anhui Provincial Natural Science Foundation (1908085MC97 and 2008085MC85), the National Natural Science Foundation of China (31802059 and 31902226), the Hefei Innovation and Entrepreneurship Support Plan for Returnee Scholar (03082009), and the Anhui Provincial Innovation and Entrepreneurship Support Plan for Returnee Scholar (2020LCX015).
Conflict of Interest
The authors declare that the research was conducted in the absence of any commercial or financial relationships that could be construed as a potential conflict of interest.
Acknowledgments
We thank MS. Dandan Zhang, Mengjuan Sun, Luyan Shentu and Mr. Tenglong Guo, Xianshu Yang, Shang Ren for their help in technical assistance.
Supplementary Material
The Supplementary Material for this article can be found online at: https://www.frontiersin.org/articles/10.3389/fcell.2021.678282/full#supplementary-material
Supplementary Figure 1 | Verification of the specificity of H3R2me2, H3R17me2, and H3R26me2 antibody. H3R2me2, H3R17me2, and H3R26me2 antibody was tested on porcine blastocysts. The secondary antibody was replaced with blocking buffer to serve as a negative control. Representative images obtained by confocal microscopy are shown. Scale bar: 50 μm.
Supplementary Figure 2 | Effect of CARM1 inhibition on cleavage-stage embryonic development, H3R2me2, and H3R17me2 levels in embryos. (A) Representative images of embryos at different stages. Scale bar: 100 μm. (B) Developmental rates of 2-cell, 4-cell, 8-cell, and morula in the control and the CARM1 inhibition groups. Effect of CARM1 inhibition on H3R2me2 (C) and H3R17me2 (D) levels in 2-cell, 4-cell, and blastocysts. Embryos at different stages were stained for H3R2me2, H3R17me2 (red), and DNA (blue). The experiment was independently repeated three times with at least 15 embryos per group. Scale bar: 50 μm. All data are shown as mean ± S.E.M and different letters on the bars indicate significant differences (P < 0.05).
Supplementary Figure 3 | Effect of CARM1 knockdown on H3R2me2 and H3R17me2 levels in embryos, and cleavage-stage embryonic development. Effect of CARM1 KD on H3R2me2 (A) and H3R17me2 (B) levels in 2-cell, 4-cell, and blastocysts. Embryos at different stages were stained for H3R2me2, H3R17me2 (red), and DNA (blue). The experiment was independently repeated three times with at least 11 embryos per group. Scale bar: 50 μm. (C) Representative images of embryos at different stages. Scale bar: 100 μm. (D) Developmental rates of 2-cell, 4-cell, 8-cell, and morula in the control and the CARM1 KD groups. All data are expressed as mean ± S.E.M and different letters on the bars indicate significant differences (P < 0.05).
Supplementary Figure 4 | Effect of CARM1 knockdown on blastocyst formation and lineage allocation of in vitro fertilized embryos. (A) Representative images of IVF blastocysts from different stages at days 5, 6, and 7. Scale bar: 100 μm. (B) Percentage of blastocyst rates at days 5, 6, and 7. (C) Representative fluorescence images of IVF blastocysts. Embryos at different groups were stained for CDX2 (green) and DNA (blue). The experiment was independently repeated three times with at least 20 embryos per group. Scale bar: 50 μm. (D) Analysis of lineage allocation in IVF blastocysts. The numbers of total cells, ICM cells, TE cells, and the ratio of ICM cells to TE cells were recorded and subject to statistical analysis. ICM: inner cell mass; TE: trophectoderm. All data are expressed as mean ± S.E.M and different letters on the bars indicate significant differences (P < 0.05).
Supplementary Figure 5 | Validation of single-embryo RNA sequencing data by qPCR. Relative abundance of six differentially expressed genes was determined by qPCR. The experiment was independently repeated three times. Data were normalized to the housekeeping gene (EF1α1) and the data from control group were set as 1. Data are shown as mean ± S.E.M and different letters on the bars indicate significant differences (P < 0.05).
References
Alberio, R. (2020). Regulation of Cell Fate Decisions in Early Mammalian Embryos. Annu. Rev. Anim. Biosci. 8, 377–393. doi: 10.1146/annurev-animal-021419-083841
Bang, J. I., Lee, E. H., Lee, A. R., Il Lee, J., Choi, S. H., Seol, D. W., et al. (2018). The effect of cell penetrating peptide-conjugated coactivator-associated arginine methyltransferase 1 (CPP-CARM1) on the cloned mouse embryonic development. Sci. Rep. 8:16721. doi: 10.1038/s41598-018-35077-0
Bao, J., Rousseaux, S., Shen, J., Lin, K., Lu, Y., and Bedford, M. T. (2018). The arginine methyltransferase CARM1 represses p300∗ACT∗CREMtau activity and is required for spermiogenesis. Nucleic Acids Res. 46, 4327–4343. doi: 10.1093/nar/gky240
Cao, Z., Xu, T., Tong, X., Wang, Y., Zhang, D., Gao, D., et al. (2019). Maternal Yes-Associated Protein Participates in Porcine Blastocyst Development via Modulation of Trophectoderm Epithelium Barrier Function. Cells 8:1606. doi: 10.3390/cells8121606
Chazaud, C., and Yamanaka, Y. (2016). Lineage specification in the mouse preimplantation embryo. Development 143, 1063–1074. doi: 10.1242/dev.128314
Cheng, D., Gao, G., Di Lorenzo, A., Jayne, S., Hottiger, M. O., Richard, S., et al. (2020). Genetic evidence for partial redundancy between the arginine methyltransferases CARM1 and PRMT6. J. Biol. Chem. 295, 17060–17070. doi: 10.1074/jbc.RA120.014704
Fu, X., Zhang, C., and Zhang, Y. (2020). Epigenetic regulation of mouse preimplantation embryo development. Curr. Opin. Genet Dev. 64, 13–20. doi: 10.1016/j.gde.2020.05.015
Gao, Y., Hyttel, P., and Hall, V. J. (2010). Regulation of H3K27me3 and H3K4me3 during early porcine embryonic development. Mol. Reprod Dev. 77, 540–549. doi: 10.1002/mrd.21180
Jacques, S. L., Aquino, K. P., Gureasko, J., Boriack-Sjodin, P. A., Porter Scott, M., Copeland, R. A., et al. (2016). CARM1 Preferentially Methylates H3R17 over H3R26 through a Random Kinetic Mechanism. Biochemistry 55, 1635–1644. doi: 10.1021/acs.biochem.5b01071
Jarrold, J., and Davies, C. C. (2019). PRMTs and Arginine Methylation: Cancer’s Best-Kept Secret? Trends Mol. Med. 25, 993–1009. doi: 10.1016/j.molmed.2019.05.007
Jiao, Y., Li, J., Zhu, S., Ahmed, J. Z., Li, M., Shi, D., et al. (2020). PI3K inhibitor reduces in vitro maturation and developmental competence of porcine oocytes. Theriogenology 157, 432–439. doi: 10.1016/j.theriogenology.2020.08.019
Kirmizis, A., Santos-Rosa, H., Penkett, C. J., Singer, M. A., Vermeulen, M., Mann, M., et al. (2007). Arginine methylation at histone H3R2 controls deposition of H3K4 trimethylation. Nature 449, 928–932. doi: 10.1038/nature06160
Liu, X., Wang, C., Liu, W., Li, J., Li, C., Kou, X., et al. (2016). Distinct features of H3K4me3 and H3K27me3 chromatin domains in pre-implantation embryos. Nature 537, 558–562. doi: 10.1038/nature19362
Lorton, B. M., and Shechter, D. (2019). Cellular consequences of arginine methylation. Cell. Mol. Life Sci. 76, 2933–2956. doi: 10.1007/s00018-019-03140-2
Maekawa, M., Yamamoto, T., Tanoue, T., Yuasa, Y., Chisaka, O., and Nishida, E. (2005). Requirement of the MAP kinase signaling pathways for mouse preimplantation development. Development 132, 1773–1783. doi: 10.1242/dev.01729
Menchero, S., Rayon, T., Andreu, M. J., and Manzanares, M. (2017). Signaling pathways in mammalian preimplantation development: Linking cellular phenotypes to lineage decisions. Dev. Dyn. 246, 245–261. doi: 10.1002/dvdy.24471
Mordhorst, B. R., and Prather, R. S. (2017). Pig Models of Reproductionds. Anim. Models Hum. Reproduct. 2017, 213–234.
Nakanishi, M. O., Hayakawa, K., Nakabayashi, K., Hata, K., Shiota, K., and Tanaka, S. (2012). Trophoblast-specific DNA methylation occurs after the segregation of the trophectoderm and inner cell mass in the mouse periimplantation embryo. Epigenetics 7, 173–182. doi: 10.4161/epi.7.2.18962
Nakasato, M., Shirakura, Y., Ooga, M., Iwatsuki, M., Ito, M., Kageyama, S., et al. (2006). Involvement of the STAT5 signaling pathway in the regulation of mouse preimplantation development. Biol. Reprod 75, 508–517. doi: 10.1095/biolreprod.105.047860
Negron-Perez, V. M., and Hansen, P. J. (2018). Role of yes-associated protein 1, angiomotin, and mitogen-activated kinase kinase 1/2 in development of the bovine blastocyst. Biol. Reprod 98, 170–183. doi: 10.1093/biolre/iox172
Nishioka, N., Inoue, K., Adachi, K., Kiyonari, H., Ota, M., Ralston, A., et al. (2009). The Hippo signaling pathway components Lats and Yap pattern Tead4 activity to distinguish mouse trophectoderm from inner cell mass. Dev. Cell 16, 398–410. doi: 10.1016/j.devcel.2009.02.003
O’Brien, K. B., Alberich-Jorda, M., Yadav, N., Kocher, O., Diruscio, A., Ebralidze, A., et al. (2010). CARM1 is required for proper control of proliferation and differentiation of pulmonary epithelial cells. Development 137, 2147–2156. doi: 10.1242/dev.037150
Parfitt, D. E., and Zernicka-Goetz, M. (2010). Epigenetic modification affecting expression of cell polarity and cell fate genes to regulate lineage specification in the early mouse embryo. Mol. Biol. Cell. 21, 2649–2660. doi: 10.1091/mbc.E10-01-0053
Paul, S., and Knott, J. G. (2014). Epigenetic control of cell fate in mouse blastocysts: the role of covalent histone modifications and chromatin remodeling. Mol. Reprod. Dev. 81, 171–182. doi: 10.1002/mrd.22219
Price, O. M., and Hevel, J. M. (2020). Toward Understanding Molecular Recognition between PRMTs and their Substrates. Curr. Protein Pept. Sci. 21, 713–724. doi: 10.2174/1389203721666200124143145
Prochazka, R., Petlach, M., Nagyova, E., and Nemcova, L. (2011). Effect of epidermal growth factor-like peptides on pig cumulus cell expansion, oocyte maturation, and acquisition of developmental competence in vitro: comparison with gonadotropins. Reproduction 141, 425–435. doi: 10.1530/REP-10-0418
Richani, D., Ritter, L. J., Thompson, J. G., and Gilchrist, R. B. (2013). Mode of oocyte maturation affects EGF-like peptide function and oocyte competence. Mol. Hum. Reprod. 19, 500–509. doi: 10.1093/molehr/gat028
Riley, J. K., Carayannopoulos, M. O., Wyman, A. H., Chi, M., Ratajczak, C. K., and Moley, K. H. (2005). The PI3K/Akt pathway is present and functional in the preimplantation mouse embryo. Dev. Biol. 284, 377–386. doi: 10.1016/j.ydbio.2005.05.033
Rossant, J. (2018). Genetic Control of Early Cell Lineages in the Mammalian Embryo. Annu. Rev. Genet. 52, 185–201. doi: 10.1146/annurev-genet-120116-024544
Schurter, B. T., Koh, S. S., Chen, D., Bunick, G. J., Harp, J. M., Hanson, B. L., et al. (2001). Methylation of histone H3 by coactivator-associated arginine methyltransferase 1. Biochemistry 40, 5747–5756. doi: 10.1021/bi002631b
Shishkova, E., Zeng, H., Liu, F., Kwiecien, N. W., Hebert, A. S., Coon, J. J., et al. (2017). Global mapping of CARM1 substrates defines enzyme specificity and substrate recognition. Nat. Commun. 8:15571. doi: 10.1038/ncomms15571
Sun, H., Su, J., Wu, T., Wang, F., Kang, J., Zhang, J., et al. (2020). CARM1 is heterogeneous in mouse four-cell embryo and important to blastocyst development. Reproduction 159, 91–104. doi: 10.1530/REP-19-0405
Torres-Padilla, M. E., Parfitt, D. E., Kouzarides, T., and Zernicka-Goetz, M. (2007). Histone arginine methylation regulates pluripotency in the early mouse embryo. Nature 445, 214–218. doi: 10.1038/nature05458
White, M. D., Angiolini, J. F., Alvarez, Y. D., Kaur, G., Zhao, Z. W., Mocskos, E., et al. (2016). Long-Lived Binding of Sox2 to DNA Predicts Cell Fate in the Four-Cell Mouse Embryo. Cell 165, 75–87. doi: 10.1016/j.cell.2016.02.032
White, M. D., and Plachta, N. (2020). Specification of the First Mammalian Cell Lineages In Vivo and In Vitro. Cold Spring Harb. Perspect. Biol. 12: a035634. doi: 10.1101/cshperspect.a035634
Wu, Q., Bruce, A. W., Jedrusik, A., Ellis, P. D., Andrews, R. M., Langford, C. F., et al. (2009). CARM1 is required in embryonic stem cells to maintain pluripotency and resist differentiation. Stem Cells 27, 2637–2645. doi: 10.1002/stem.131
Xia, P., Wang, S., Ye, B., Du, Y., Li, C., Xiong, Z., et al. (2018). A Circular RNA Protects Dormant Hematopoietic Stem Cells from DNA Sensor cGAS-Mediated Exhaustion. Immunity 48:e687. doi: 10.1016/j.immuni.2018.03.016
Yang, G., Zhou, C., Wang, R., Huang, S., Wei, Y., Yang, X., et al. (2019). Base-Editing-Mediated R17H Substitution in Histone H3 Reveals Methylation-Dependent Regulation of Yap Signaling and Early Mouse Embryo Development. Cell. Rep. 26:e304. doi: 10.1016/j.celrep.2018.12.046
Keywords: CARM1, H3R26me2, blastocyst, pig, lineage specification
Citation: Cao Z, Tong X, Yin H, Zhou N, Zhang X, Zhang M, Wang X, Liu Q, Yan Y, Ma Y, Yu T, Li Y and Zhang Y (2021) Histone Arginine Methyltransferase CARM1-Mediated H3R26me2 Is Essential for Morula-to-Blastocyst Transition in Pigs. Front. Cell Dev. Biol. 9:678282. doi: 10.3389/fcell.2021.678282
Received: 09 March 2021; Accepted: 10 May 2021;
Published: 02 June 2021.
Edited by:
Ian R. Adams, University of Edinburgh, United KingdomReviewed by:
Ramiro Alberio, University of Nottingham, United KingdomChih-Jen Lin, University of Edinburgh, United Kingdom
Copyright © 2021 Cao, Tong, Yin, Zhou, Zhang, Zhang, Wang, Liu, Yan, Ma, Yu, Li and Zhang. This is an open-access article distributed under the terms of the Creative Commons Attribution License (CC BY). The use, distribution or reproduction in other forums is permitted, provided the original author(s) and the copyright owner(s) are credited and that the original publication in this journal is cited, in accordance with accepted academic practice. No use, distribution or reproduction is permitted which does not comply with these terms.
*Correspondence: Yunhai Zhang, yunhaizhang@ahau.edu.cn
†These authors have contributed equally to this work