Mechanisms of Action of MiRNAs and LncRNAs in Extracellular Vesicle in Atherosclerosis
- 1Department of Geriatrics, The Second Xiangya Hospital, Central South University, Changsha, China
- 2Institute of Aging and Age-related Disease Research, Central South University, Changsha, China
Atherosclerosis, a complex chronic inflammatory disease, involves multiple alterations of diverse cells, including endothelial cells (ECs), vascular smooth muscle cells (VSMCs), monocytes, macrophages, dendritic cells (DCs), platelets, and even mesenchymal stem cells (MSCs). Globally, it is a common cause of morbidity as well as mortality. It leads to myocardial infarctions, stroke and disabling peripheral artery disease. Extracellular vesicles (EVs) are a heterogeneous group of cell-derived membranous structures that secreted by multiple cell types and play a central role in cell-to-cell communication by delivering various bioactive cargos, especially microRNAs (miRNAs) and long non-coding RNAs (lncRNAs). Emerging evidence demonstrated that miRNAs and lncRNAs in EVs are tightly associated with the initiation and development of atherosclerosis. In this review, we will outline and compile the cumulative roles of miRNAs and lncRNAs encapsulated in EVs derived from diverse cells in the progression of atherosclerosis. We also discuss intercellular communications via EVs. In addition, we focused on clinical applications and evaluation of miRNAs and lncRNAs in EVs as potential diagnostic biomarkers and therapeutic targets for atherosclerosis.
Introduction
Atherosclerosis refers to a complex chronic inflammatory disease that involves endothelial dysfunction, mononuclear cell accumulation, coagulation and thrombosis, cell proliferation and migration, as well as lipid deposition (1). Numerous cells, including endothelial cells (ECs), vascular smooth muscle cells (VSMCs), monocytes, macrophages, platelets and mesenchymal stem cells (MSCs) are involved in the initiation and progression of atherosclerosis (2). Globally, atherosclerosis is the major underlying cause of cardiovascular diseases, cerebrovascular diseases and peripheral vascular diseases, and is strongly associated with high morbidity and mortality rates (3). Therefore, studies should aim at identifying novel diagnostic tools and innovative therapies to delay or curb the development of atherosclerosis and prevent adverse complications, such as stroke and myocardial infarction.
Extracellular vesicles (EVs) originate from diverse cells are a heterogeneous group of cell-derived membranous structures, with an average diameter range from 20 to 1,000 nm (4–6). Prompted by the International Society of Extracellular Vesicles (ISEV), EVs are a general term of various subtypes of membrane structures released by cells. Accordingly, EVs include exosomes (50–150 nm in diameter) (7), ectosomes (ranging from 100 to 350 nm in diameter) (8), microvesicles (100 nm to 1 μm in diameter) (9), apoptotic bodies (ranging from 500 nm to >2 μm in diameter) (10), oncosomes (1 μm to up to 10 μm in diameter) (11), and other non-defined EV subtypes (12). EVs contain various bioactive molecules, including glycoconjugates, proteins, lipids, mRNAs, and non-coding RNAs, such as miRNAs and lncRNAs (13). In addition, exosome markers, including CD9, CD63, CD81, flotillin-1 and 2, ALIX, TSG101, and syntenin-1 are not strictly specific to exosomes (14–16) (Figure 1). Throughout this review the different types of vesicles will be referred to as EVs. It has been shown that EVs emerge as pivotal modulators of gene transcriptional expression and intercellular communication (5). Notably, emerging publications indicated that EVs released by multiple cell types are implicated in the pathogenesis and progression of atherosclerosis via miRNAs and lncRNAs delivery.
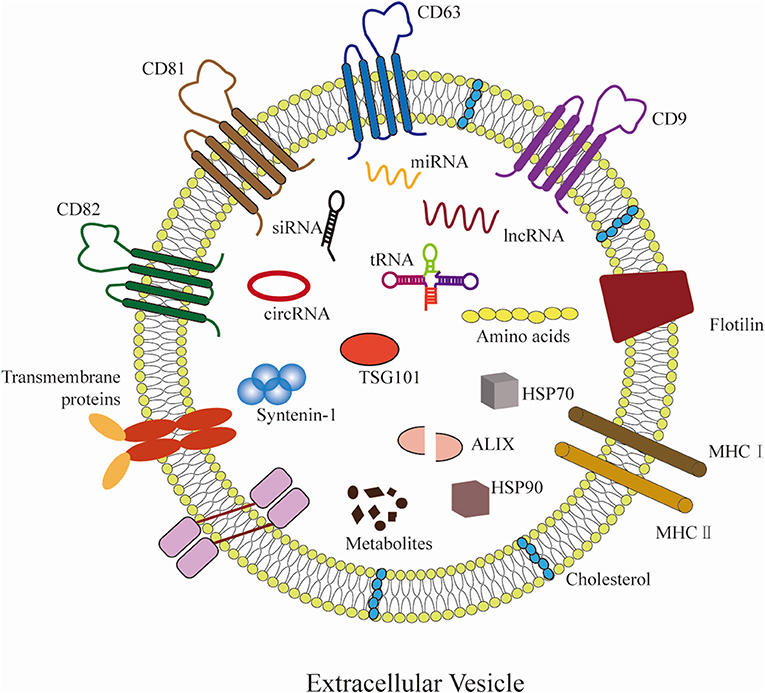
Figure 1. Hallmarks of EVs. EVs can be secreted by almost all cells. EVs carry various molecular cargos, including proteins, lipids, metabolites, and nucleic acids (mRNAs, miRNAs, lncRNAs, etc.). Proteins include tetraspanins (CD9, CD63, CD81, and CD82), ESCRT proteins (such as ALIX and TSG101), and syntenin-1 have emerged as commonly used markers for EVs. Some molecules are carried on the surface of EVs, including major histocompatibility complex (MHC) class I/II molecules, flotillin, and transmembrane proteins, etc.
MiRNAs are endogenous single-stranded RNAs, composed of 20–25 nucleotides, which are post-transcriptional modulators that exhibit a critical role in modulating gene expression and protein synthesis (17). LncRNAs are defined as a class of long non-coding RNAs with a length of more than 200 nucleotides (18). Interestingly, it has been reported that both exosomal miRNAs and lncRNAs are implicated in atherosclerosis progression by affecting the function of different cells, including ECs, VSMCs, and immune cells. Although extensive research has been carried out on the EVs containing miRNAs and lncRNAs in atherosclerosis, the latent mechanisms are still not well-clarified.
In this review, we will focus on the cumulative roles of miRNAs and lncRNAs packaged in diverse cell types-derived EVs during atherosclerosis development. We also discuss cell-to-cell communications via EVs and miRNAs and lncRNAs delivery. In addition, we provided a comprehension of clinical applications and evaluation of miRNAs and lncRNAs in EVs as potential diagnostic biomarkers and therapeutic targets for atherosclerosis, focusing on recent developments in the field.
MiRNAs and lncRNAs in EVs From Different Cells in Atherosclerosis
Endothelial dysfunction, VSMCs proliferation and migration, and immune cells infiltration are tightly associated with atherosclerosis progression. EVs can be released from almost all cell types, including ECs, VSMCs, monocytes, macrophages, DCs, platelets, and MSCs (19). A host of data supports that EVs play significant roles in the regulation of occurrence and development of atherosclerosis by delivering miRNAs and lncRNAs to recipient cells (20).
MiRNAs in EVs From Different Cells in Atherosclerosis
MiRNAs are a sort of non-coding RNAs, which mediate protein synthesis through their interactions with messenger RNAs during atherosclerosis (21). Increasing evidence demonstrates that miRNAs packaged in EVs can modulate atherosclerosis through regulating inflammation, VSMCs proliferation and migration, mononuclear macrophage adhesion, as well as platelets activation. In this part, we summarize the emerging roles of miRNAs in EVs from different cells in atherosclerosis (Table 1).
ECs
ECs form the main components of the intima and act as a barrier between blood and tissues. A growing number of studies suggest that ECs-derived EVs are important in the pathogenesis of atherosclerosis (2). In addition, EVs are implicated in altering lipid metabolism and homeostasis, both of which are important for atherosclerosis.
MiRNAs in ECs-derived EVs play essential roles in atherosclerosis. MiRNA-126 packaged in EVs from ECs transferred to recipient cells can promote ECs proliferation, migration, and reendothelialization through modulating the target protein sprouty-related, EVH1 domain-containing protein 1 (SPRED1). A study analysis of 176 patients with coronary artery disease with and without diabetes mellitus found that the expression of miRNA-126 was decreased in EVs from diabetic patients (24). ECs-derived EVs can promoted anti-inflammation by reducing the expression of ICAM-1 through the transfer of miR-222 into recipient cells. EVs from high glucose-treated ECs exhibit a downregulated level of miR-222 and reduced anti-inflammation (30). It has been reported that EVs released from ECs can attenuate atherosclerosis by inhibiting inflammation. When transferred into monocytes, miRNA-10a in EVs from ECs exert anti-inflammatory effects by down-regulating the production of pro-inflammatory genes, including IRAK4, MAP3K7, and BTRC, and inhibiting the nuclear factor-kappa B (NF-κB) signaling pathway (22). MiR-126 and miR-210 of EVs were upregulated in ECs overexpressing hypoxia-inducible factor (HIF)-1. The transfer of miR-126 and miR-210 inhibited macrophages infiltration and reduced plaque area (3). EVs derived from human umbilical vein ECs (HUVECs) under stimulus, such as shear stress, exhibit a high level of miRNA-143/145 (27). Hergenreider et al. found that ECs-derived EVs overexpressed shear-responsive transcription factor Krüppel-like factor 2 (KLF2) induces miRNA-143/145 accumulation in ECs. Subsequently, EVs from ECs transfer miRNA-143/145 to VSMCs, thereby mediating the communication between ECs and VSMCs. Moreover, they found that overexpression of KLF2 in ECs attenuates the size of atherosclerotic plaque in ApoE(-/-) mice (27). In addition, miRNA-126 was enriched in EVs secreted by ECs. It promotes G protein-coupled receptor (GPCR) signaling by suppressing the function of the regulator of G protein signaling 16, thereby enhancing the production of CXC chemokine ligand 12 (CXCL12) (25). EVs produced from ECs containing miRNA-126 repress the amounts of macrophages and apoptotic cells in plaques, and increase the amount of VSMCs, resulting in less inflammation and small lesions. Ohta et al. reported that elevated IL-6 levels suppress the expression of miRNA-126-3p in EVs derived from ECs and promote monocyte cell line THP-1 cells adhesion, eventually enhancing intercellular adhesion molecule-1 (ICAM-1) levels, leading to exacerbation and deterioration of atherosclerotic plaques. In contrast, elevated the expression level of miRNA-126-3p has the opposite effects (26). The level of miRNA-19b was upregulated in EVs from hypoxia-induced ECs. The transfer of EVs MiRNA-19b can inhibit migration and angiogenesis of ECs through decreasing the expression of transforming growth factor-β2 (TGF-β2) (23). Lombardo et al. found that IL-3 increases the secretion of EVs and transmission of miRNA-126-3P from ECs to wounds by promoting the activity of signal transduction and activator of transcription 5 (STAT5), thereby stimulating angiogenesis (72). Additionally, ECs-derived EVs modulate ECs function, vascular angiogenesis through the delivery of miRNA-214 between ECs (73). van Balkom et al. reported that miRNA-214 enriched in EVs inhibits ataxia telangiectasia mutation and promote angiogenesis. Suppressing miRNA-214 levels shows opposite effects (73). On the contrary, miRNAs encapsulated in EVs from ECs can damage the function of blood vessels and accelerate atherosclerosis progression. Atherosclerosis associated inflammation enhances inflammatory factor accumulation (74), including tumor necrosis factor-α (TNF-α), which promotes the expression of miRNA-155 in ECs-derived EVs. MiRNA-155 of EVs downregulates endothelial Nitric oxide synthase (eNOS) expression and weakens endothelium vasorelaxation. Low production of eNOS contribute to endothelial dysfunction and impair vascular homeostasis (75). Furthermore, simvastatin has been shown to reduce the expression of miRNA-155 in EVs and improve eNOS generation (28, 76). In addition, miRNA-505 in EVs from ox-LDL treated HUVECs have been shown to enhance atherosclerotic development in mice models through impairing blood vessels function. Mechanistically, EVs released from ox-LDL-induced HUVECs co-cultured with neutrophils transfer miRNA-505 to neutrophils, activate the NF-κB pathway and inhibit SIRT3 in neutrophils, resulting in reactive oxygen species (ROS) accumulation and increased formation of neutrophil extracellular traps (NETs) (31).
VSMCs
Vascular media is mainly composed of VSMCs, which are important in maintaining physiological functions and structural integrity of blood vessels. VSMCs proliferation is vital in plaque repair and might be a beneficial process in early atherosclerosis, while VSMCs death and senescence induce plaque instability and promote atherosclerosis (77).
VSMCs-derived EVs exert significant effects in regulation of inflammation, cell migration, vascular calcification and cell coagulation among others. Additionally, EVs secreted by VSMCs can be elevated through stimulation by platelet derived growth factor (PDGF) and TNF-α, leading to VSMCs calcification. VSMCs-derived EVs that are rich in the miRNA-143/145 cluster play important roles in modulating VSMCs differentiation, quiescent vs. proliferative phenotype and vascular homeostasis (78). Climent et al. reported that transforming growth factor-β (TGF-β) or vessel stress-treated VSMCs-derived EVs mediate ECs angiogenesis and vascular stability through the transfer of miR-143/145 (79). Besides, molecules such as TNF-α and PDGF-BB enhance production of miRNA-143 in EVs, resulting in cell proliferation, cell migration, and VSMCs calcification (3, 80, 81). MiRNA-143-3p rather than miRNA-143-5p is enriched in EVs from pulmonary artery smooth muscle cells (PASMCs), and regulated PASMCs migration. Deng et al. revealed that miR-143-3p packaged in EVs derived from PASMC paracrine to ECs and act as a proangiogenic regulator (80). In addition, EVs from VSMCs transfer miRNA-150 to ECs, enhancing the expression of vascular endothelial growth factor-A (VEGF-A) and promoting the VEGF-A/VEGFR/PI3K/Akt pathway, leading to ECs migration (35). Besides, miRNA-150 knockdown in VSMCs attenuate ECs migration (35). Studies have shown that miRNA-150 overexpression induced by either spliced X-box binding protein 1 (XBP1) or PDGF-BB is crucial in regulating ECs and VSMCs function as well as in maintaining vessel homeostasis (35, 82). Dysregulation of autophagy by ECs has an effect on the progression of atherosclerosis. MiRNAs in EVs bind autophagy-related genes to inhibit ECs autophagy. Human aortic SMCs co-cultured with HUVECs were shown to suppress the autophagic activity of HUVECs through upregulating the levels of miRNA-221/222 in EVs from human aortic SMCs. Phosphatase and tensin homolog deleted on chromosome 10 (PTEN) is an anti-inflammatory and antifibrotic modulator, mediating VSMCs differentiation, migration and proliferation (83). The elevated expression levels of miRNA-221/222 in EVs downregulated the expression of PTEN in HUVECs and activated the Akt pathway, resulting in LC3II, Beclin-1, ATG5 downregulation and SQSTM1/p62 upregulation (37). Furthermore, atherosclerosis is associated with downregulated miRNA-133a levels (84). Gao et al. reported that miRNA-133a levels were decreased in ApoE(-/-) atherosclerosis mice models and were associated with inhibited insulin-like growth factor-1 receptor (IGF-1R) levels as well as suppressed VSMC growth (33). In addition, overexpression of miRNA-133a targets proapoptotic genes, including Bim (Bcl2l11) and Bmf (Bcl-2 modifying factor), inhibiting cell apoptosis and promoting proliferation of cardiac progenitor cells (34). On the contrary, overexpression of KLF5 in VSMCs induces miRNA-155 production and secretion (36). KLF5 plays a role in promoting VSMCs proliferation by upregulating the generation of growth factors such as PDGF-A (85). VSMC-derived EVs transfer miRNA-155 to ECs, impairing EC barriers by weakening tight junctions and coherence, leading to increased endothelial permeability and enhanced atherosclerotic development (36). Moreover, miRNA-92a and miRNA-195 in EVs released from VSMCs shuttled into macrophages to regulating inflammation and promoting atherosclerosis through elevating the expression levels of IL-6 and CXCL1 (32).
Monocytes/Macrophages
Monocyte-endothelial cell adhesion induces numerous monocyte progression to macrophage- or DC- like phenotypes in early atheromata. Risk factors such as hyperlipidemia and hypertension can trigger vascular inflammation and further stimulate the expression of adhesion molecules such as ICAM-1, VCAM-1, and selectin E in ECs. These molecules promote monocyte-ECs adhesion and monocytes infiltration into the subcutaneous space of ECs (86). In early atheromata, the majority of monocyte progression to macrophage- or DC- like phenotypes (87). Many macrophages and dendritic-like cells have cytoplasmic membrane-bound lipid droplets (foam cells). Foam cells secrete numerous pro-inflammatory cytokines and promote monocyte proliferation and accumulation. Accumulating data suggests that macrophages regulate atherosclerosis development by destabilizing atherosclerotic plaques. Ox-LDL induces monocytes/macrophages inflammatory response emerge as a key event in atherosclerosis.
EVs released from macrophages stimulate pro-inflammatory responses by transporting miRNAs and lncRNAs into recipient cells (88). For example, M1-like-type macrophages secrete numerous pro-inflammatory EVs, with anti-angiogenic properties (45). Besides, M2-like-type macrophage-derived EVs have both pro-inflammatory and anti-inflammatory roles (89). Monocyte/macrophage-derived EVs can modulate cell proliferation and migration, inflammation, apoptosis and angiogenesis. Zhang et al. reported that miRNA-150 is transferred from THP-1 cells to ECs through EVs. Mechanistically, THP-1 cells-derived EVs were shown to have an abundance of miRNA-150, which are transferred to human microvascular ECs-1 (HMECs-1), resulting in HMECs-1 migration by downregulating c-Myb (43). Notably, c-Myb, a nuclear transcription factor, was found to be highly expressed in atherosclerotic mice models and acted as a critical regulator of the atherosclerosis process (90). In addition, miRNA-21-3p and miRNA-142a-5p of EVs are secreted by macrophages. Macrophages exposed to nicotine trigger the transfer of miRNA-21-3p to VSMCs via EVs, and induce VSMCs proliferation and migration by directly inhibiting PTEN (38). EVs containing miRNA-106a-3p can be transferred from ox-LDL-treated THP-1 macrophages to VSMCs, thereby promoting VSMCs proliferation and inhibiting cell apoptosis by combining with CASP9 to modulate the Caspase signaling pathway. Contrastingly, treatment with EVs secretion inhibitor, GW4869, suppresses EVs secretion from ox-LDL-induced THP-1 cells, decreasing VSMCs viability and increasing cell apoptosis (40). Wang et al. reported that the transfer of miRNA-222 in EVs from M1 macrophages to VSMCs elevated VSMCs proliferation and migration through the mediation of Cyclin Dependent Kinase Inhibitor 1B (CDKN1B) and CDKN1C in vitro and enhanced neointima formation after carotid artery injury in vivo, which was reversed by 2'OMe-miR-222, a miRNA-222 inhibitor (46). Human THP-1 macrophages exposed to ox-LDL were shown to exhibit elevated expression levels of miRNA-155 in a dose-dependent manner. MiRNA-155 from THP-1 cells is an atheroprotective regulator that inhibits inflammatory responses during the pathogenesis of atherosclerosis (44). Silencing miRNA-155 in THP-1 cells significantly promotes lipid intake, upregulates the secretion of scavenger receptors, leading to the accumulation of inflammatory factors such as IL-6, IL-8, and TNF-α. Furthermore, attenuation of miRNA-155 activation significantly elevates myeloid differentiation primary response gene 88 (MyD88) levels and suppresses the NF-κB pathway. M1 macrophage-derived EVs carrying miRNA-155 shuttle into ECs, playing an anti-angiogenesis role by inhibiting the activity of Sirtuin 1/protein kinase AMP-activated catalytic subunit alpha 2 (AMPKα2), endothelial nitric oxide synthase and Rac family small GTPase 1 (RAC1)/p21-activated kinase 2 (PAK2) signaling pathways (45). MiRNA-210 is a critical anoxic-related miRNA in EVs from cardiac progenitor cell. It promotes angiogenesis and suppresses myocardial cells apoptosis (91). Moreover, HIF-1α in inflammatory and lesional macrophages increases miRNA-210 expression and decreases miRNA-383 production, reduces ATP levels and elevates necroptosis and atherosclerosis through targeting 2,4-dienoyl-CoA reductase and mitochondrial ROS accumulation (92). Moreover, miR-210-3p suppresses the NF-κB pathway by inhibiting IGF2/IGF2R, resulting in amelioration of lipid congestion and inflammation (93). Furthermore, differentiation of macrophages induces EVs secretion and stimulates miRNA-233 delivery to recipient cells. Elevated miRNA-233 levels promote macrophages differentiation and apoptosis (94). It has been reported that miR-223, which is enriched in macrophage-derived EVs, acts as an inhibitor of Pknox1, thereby repressing macrophages inflammatory responses and downregulating adipose tissue inflammation (47). IL-4 induced naive bone marrow macrophages-derived EVs exhibit high expression levels of miRNA-99a/146b/378a, as well as downregulate NF-κB and TNF-α signaling pathways. Therefore, macrophages are polarized toward the M2-like-type, which ameliorates atherosclerosis lesion areas (39). On the contrary, miRNA-146a in EVs derived from macrophages can accelerate the development of atherosclerosis. EVs produced by ox-LDL exposed macrophages are rich in miRNAs, such as miRNA-146a, miR-185, miR-128, miR-503, and miR-365. It has been reported that EVs contained miRNAs transferred from donor macrophages to naive recipient macrophages are involved in the pathogenesis of atherosclerosis. MiRNAs encapsulated in EVs, especially miRNA-146a, from atherogenic macrophages, inhibit recipient macrophages migration and promote macrophages accumulation in vessel walls by suppressing the expression of insulin-like growth factor 2 mRNA-binding protein 1 (IGF2BP1) and human antigen R or ELAV-like RNA-binding protein 1 (HuR), thereby accelerating atherosclerosis progression (41). It has been accepted that IGF2BP1 is associated with cell proliferation and growth while HuR is involved in cell proliferation, differentiation, apoptosis and senescence (95, 96). Zhang et al. found that miRNA-146a was enriched in EVs produced from THP-1 macrophages treated with ox-LDL. MiRNA-146a promoted atherogenesis by targeting superoxide dismutase 2 (SOD2) and increasing the production of ROS and NETs (42).
DCs
DCs, a group of antigen-presenting cells, are implicated in the pathogenesis of atherosclerosis. During the development of atherosclerosis, DCs play roles in anti-inflammation, antigen presentation, lipid metabolism, and efferocytosis. Normal arteries have been shown to have low levels of DCs while atherosclerotic tissues have been shown to have elevated DC levels, implying that DCs play a critical role in atherosclerosis (97).
EVs secreted by DCs stimulated by other antigens seem to be atherogenic. Mature DCs-derived EVs are associated with ECs inflammation and atherosclerosis progression through the NF-κB signaling pathway. MiRNA-16 and miRNA-21 were found to be enriched in EVs from bone marrow-derived DCs treated with low-intensity pulsed ultrasonography (LIPUS). DCs-derived EVs co-cultured with HUVECs can inhibit TNFα-induced inflammation by ameliorating the expression of ICAM-1 and vascular cell adhesion molecule (VCAM)-1, subsequently inhibiting the NF-κB signaling pathway (48). In addition, it has been admitted that miRNA-155 promotes inflammation while miRNA-146a suppresses it. DCs-derived EVs exhibit elevated levels of miRNA-146a and miRNA-155. MiRNA-146a and miRNA-155 packaged in EVs from DCs, uptaken by recipient DCs, are involved in intercellular communication and modulation of inflammatory responses (50). Zhong et al. co-cultured mature DCs-derived EVs with HUVECs and found that EVs from mature DCs promoted the expression of adhesion molecules, such as VCAM-1 and E-Selectin by a quick activation of NF-κB signaling pathway. Further study indicated that mature DCs-derived EVs shuttled miRNA-146a into HUVECs, inhibiting interleukin 1 receptor associated kinase, which repressed the second stimulation of HUVECs via EVs and inhibiting ECs inflammation (49). The expression of miR-203-3p in EVs was downregulated in ox-LDL-induced bone marrow-derived macrophages (BMDMs). Lin et al. found that the transfer of miR-203-3p targeted cathepsin S in bone marrow-derived macrophages to attenuate atherosclerosis development in mice (51).
Platelets
Platelets, inflammatory cells with a well-established role in hemostasis and thrombosis, play central roles in the progression of atherosclerosis. Vascular exposure to stimuli such as hyperlipidemia and hyperglycemia initiates platelet activation as well as interactions with ECs and leukocytes, which has been implicated in atherosclerosis.
Platelet-derived EVs interact with monocytes, ECs and VSMCs, thereby promoting atherosclerosis progression. EVs secreted by thrombin-activated platelets inhibit ECs inflammation, cell proliferation and migration, angiogenesis and atherogenesis through miRNAs. MiRNA-25-3p has been shown to be enriched in EVs derived from thrombin induced platelets in atherosclerosis mice models. Peripheral blood platelet-derived EVs inhibited the expression of Adam 10 and further suppressed coronary vascular ECs inflammation and lipid deposition through reducing the levels of IL-1β, IL-6, TNF-α, α-smooth muscle actin, Collagen I a1, Collagen III a1, total cholesterol, and triglycerides through the delivery of miR-25-3p. In addition, miRNA-25-3p packaged in EVs from platelets alleviates ECs inflammation by suppressing the NF-κB signaling pathway (52). Li et al. found that thrombin-activated platelet-derived EVs from atherosclerotic animal models were rich in miRNA-21, miRNA-223, and miRNA-339. MiRNA-223 transferred to HUVECs to suppressed ICAM-1 production, which can be reversed by a miRNA-223 inhibitor. Thrombin-activated platelets-derived EVs carry miRNA-223 and inhibit ECs inflammation by modulating MAPK and NF-κB signaling pathways (56). Moreover, platelets-derived EVs contain miRNA-223, which regulates ECs apoptosis by decreasing IGF-1R levels (55). The expression levels of miRNA-21, miRNA-223, and miRNA-339 are associated with platelet activation and are enriched in platelet-derived EVs. They inhibited co-cultured VSMCs proliferation by suppressing PDGFRβ production, which is associated with VSMCs invasion and migration (57, 98). Additionally, in acute coronary syndrome patients, miRNA-126 has been shown to be significantly elevated in platelet-derived EVs. Isolated EVs, incubated with HUVECs, promote cell proliferation and migration by increasing the production of angiogenic factors, such as VEGF, basic Fibroblast Growth Factor and TGF-β1 (53). Thrombin-activated platelets-derived EVs contained a high level of miRNA-142-3p and shuttled miR-142-3p into ECs to promoted ECs proliferation through targeting Bcl-2-associated transcription factor-1(BCLAF-1) and its downstream genes (54). MiRNA Let-7a was enriched in platelet-derived EVs. The transfer of miRNA let-7a from platelet to HUVECs promoted angiogenesis through downregulating the generation of thrombospondin-1 (THBS-1), an anti-angiogenic factor (99). MiR-320b secreted by activated platelets was slightly elevated in the circulation of myocardial infarction patients. Activated platelet-derived EVs transfer miRNA-320b to human microvascular endothelial cell-1 cells to mediate inflammatory responses through downregulating the expression of ICAM-1 (58, 84).
MSCs
MSCs with the characteristics of self-renewal and multipotent differentiation as well as the capability of anti-inflammation may be promising therapeutic strategies for atherosclerosis. For example, bone marrow-derived MSCs (BMSCs) enhance the generation of anti-inflammatory cytokines, such as TGF-β1 and IL-10 and inhibit the expression of pro-inflammatory cytokines, such as TNF-α and IL-6 (100). An increasing number of publications indicate that EVs secreted by MSCs are served as atheroprotective regulators through regulating intercellular communications.
MSCs-derived EVs are implicated in regulating cell proliferation, migration, and apoptosis, and alleviating atherosclerotic plaque formation via miRNAs. Expression levels of miRNA-30b are highly elevated in MSCs-derived EVs. Co-cultures with HUVECs revealed that EVs transfer miRNAs from MSCs into HUVECs and enhance angiogenesis by suppressing delta-like 4 (DLL4) levels, a negative modulator of angiogenesis (59, 101). In addition, human adipose MSCs-derived EVs exhibit high expression of miRNA-125a that inhibit the production of DLL4, leading to ECs angiogenesis and the improvement of endothelial tip cell formation (61). MiRNA-31 was up-regulated in EVs from endothelial differentiation medium-treated adipose-derived stem cells. EVs containing miRNA-31 shuttled from SCs into HUVECs to regulate cell migration and tube formation through suppressing HIF-1 (60). Overexpression of miRNA-126 in MSCs-derived EVs were shown to promote cell proliferation and migration by enhancing the production of EGF, VEGF, bFGF, and PDGF in Hypoxia/Reoxygenation-injured ECs, as well as MSCs. Besides, miRNA-126-3p was found to be enriched in EVs from human umbilical cord MSCs. In rat models, miRNA-126-3p was shown to enhance HUVECs proliferation, migration, and tube formation through the SPRED-1/PIK3R2/AKT/ERK1/2 pathways (102). In contrast, Wang et al. reported that MSCs-derived miRNA-125b transfer to VSMCs through EVs suppresses VSMCs proliferation and migration as well as neointimal hyperplasia by ameliorating the expression of myosin 1E (Myo1e) (62). MSCs-derived EVs carrying miRNA-145 shuttled into HUVECs. The transfer of miRNA-145 inhibits cell migration and ameliorates atherogenesis plaque development in vivo by suppressing junctional adhesion molecule A (JAM-A), which plays an essential role in maintaining endothelial barrier functions (66, 103). Furthermore, MSCs-derived EVs containing miRNA-126 shuttled into ECs to attenuated ECs apoptosis by increasing the activity of PI3K/Akt/eNOS signaling pathway (65). Additionally, miRNA-342-5p in EVs from adipose-derived MSCs inhibits HUVECs apoptosis and protects ECs function against atherosclerosis by inhibiting PPP1R12B expression (69). Moreover, miRNA-125b-1-3p in EVs was highly expressed in human adipose-derived MSCs and reduced B-cell chronic lymphocytic leukemia (CLL)/lymphoma 11B (BCL11B) levels in lymphocyte, leading to lymphocyte apoptosis in atherosclerotic arterial tissues and in amelioration of atherosclerosis (63). EVs secreted by BMSCs from aorta tissues of ApoE(-/-) atherosclerosis mice models were shown to contain significantly elevated miRNA-125b-5p levels, which induced the suppression of mitogen-activated protein 4 kinase 4 (Map4k4), down-regulated MMP-9 expression, inflammation, blood lipid accumulation and plaque size, as well as increased α-SMA expression, thereby alleviating apoptosis in atherosclerosis mice (64). Furthermore, MSC-derived EVs can also attenuate atherosclerosis by modulating inflammation. ECs endocytosis of EVs with highly expressed miRNA-512-3p from MSCs was shown to suppress ox-LDL induced EC injury, Caspase-3 activation and cell apoptosis, decreased the secretion of inflammatory cytokines such as oxidative factor MDA, TNF-α, IL-1β and IL-6, increased GSH-PX and SOD levels and promoted ECs proliferation. Mechanistically, the transfer of miRNA-512-3p in EVs from MSCs inhibits Keleh-like ECH-associated protein 1 (Keap1) to alleviate ox-LDL-induced ECs dysfunction (70). In addition, EVs released from MSCs transfer miRNA-let7 into macrophages, where they promote M2 macrophage polarization, inhibit M1 macrophage polarization and alleviates atherosclerotic plaque in ApoE-/- mice models through the miR-let7/HMGA2/NF-κB signaling pathway (71). Moreover, MSCs-derived EVs suppress macrophage infiltration in the atherogenesis plaque through the miR-let7/IGF2BP1/PTEN pathway (71). Furthermore, EVs containing miRNA-301 transferred from BMSCs can protect against atherosclerosis through inhibiting myocardial autophagy (68). BMSCs-derived EVs carrying miR-223 to suppress the expression of nod-like receptor protein 3 (NLRP3) and inhibit macrophages pyroptosis, thereby inhibiting the rupture of atherosclerotic plaques (67).
LncRNAs in EVs From Different Cells in Atherosclerosis
In recent years, numerous studies have demonstrated that lncRNAs act as endogenous sponges to mediate the function and expression of miRNAs (104). Accumulating evidence suggests that EVs containing lncRNAs are delivered to recipient cells to participate in macrophage polarization and proliferation and migration of VSMCs (Table 2).
EVs contain a variety of lncRNAs were associated with inflammation. Studies have revealed that lncRNA ZEB1-AS1 was involved in ECs apoptosis and macrophage inflammation by regulating the ZEB1-AS1/miR-942/HMGB1 axis (113). Chen et al. found that ZEB1-AS1 was enriched in EVs from ox-LDL-treated HUVECs, where it induced inflammation, proliferation, and apoptosis by mediating the expression of E26 oncogene homolog 1 (ETS1). ETS1 promotes the activation of the TGF-β/Smad signing pathway, however, further research reveals that ZEB1-AS1 knockdown reverses this effect. Therefore, ameliorating the expression of ZEB1-AS1 may be a promising and potential therapeutic strategy for atherosclerosis (110). In addition, Wang et al. investigated the levels of EVs and EVs carried lncRNA HIF1A-AS1 in 35 atherosclerosis patients and 28 healthy adults. The study found that EVs and HIF1A-AS1 of EVs were highly expressed in atherosclerosis patients. Therefore, HIF1A-AS1 in EVs can serve as a potential diagnostic biomarker for atherosclerosis (114). Shan et al. established that lncRNA-RNCR3 is elevated in ECs and VSMCs of mouse and human atherosclerotic plaque. RNCR3 knockdown in ECs and VSMCs leads to the accumulation of inflammatory molecules, impairs the function of ECs and VSMCs, and worsens atherosclerosis (105). Besides, alleviating the level of RNCR3 in ECs by RNase A or proteinase K reduces the proliferation and migration ability of VSMCs. HUVECs-derived EVs containing RNCR3 has emerged as a competing endogenous RNA of miR-185-5p, leading to an increase in KLF2 (115). EVs released by ox-LDL-induced HUVECs carried a high level of LINC01005. ECs-derived EVs promoted VSMCs proliferation and migration through the transfer of LINC01005. Mechanistically, LINC01005 acted as a sponge of miR-128-3p and elevate the level of KLF4 (106). LncRNA-MALAT1 was upregulated in lipopolysaccharide (LPS)-activated macrophages. Mechanistically, MALAT1 interacts with the NF-κB signaling pathway to reduce the production of inflammatory cytokines (such as TNF-α and IL-6) through inhibiting its DNA binding activity (116). Huang et al. established that MALAT1 packaged in EVs was elevated in oxLDL-treated HUVECs. EVs carrying MALAT1 co-cultured with macrophages promoted M2 macrophage polarization, however, the loss of MALAT1 can reverse this effect (107). Rahman et al. reported that M2 macrophages play an essential role in inflammation and regression of atherosclerotic plaque (117). Moreover, EVs shed by oxLDL-induced ECs exhibited a high level of MALAT1. In ox-LDL treated HUVECs co-cultured with neutrophils, MALAT1 in EVs acts as a regulator increasing NETs formation and trigger hyperlipidemia and inflammatory responses, which in turn exacerbate atherosclerosis (109). Additionally, MALAT1 in EVs shuttles from ECs into recipient DCs and ameliorates the development of atherosclerosis. Overexpression of MALAT1 in EVs delivered to immature DCs inhibits ROS congestion and represses DCs maturation by interacting with the Nrf2 signaling pathway. Nrf2 is vital in modulating antioxidant gene expression and serves as an anti-inflammatory mediator (108, 118). Additionally, MALAT1 in EVs from HUVECs treated with ox-LDL exhibit lower expression compared with EVs from HUVECs without stimuli, an indication that the loss of MALAT1 in EVs promotes DCs maturation in atherosclerosis. Increasing evidence suggests that lncRNAs are significantly elevated in atherosclerotic plaque of patients and animal models and play an important role in atherosclerosis. LncRNA GAS5 is enriched in EVs from ox-LDL -induced THP-1 macrophages and it is more abundant in ECs, where they promote ECs apoptosis together with apoptotic factors such as caspases. GAS5 knockdown in THP-1 cells-derived EVs alleviates ECs apoptosis. In contrast, overexpression of GAS5 in THP-1 cells exposed to ox-LDL leads to THP-1 cell apoptosis, while GAS5 knockdown inhibits the apoptosis of THP-1 cells (112). Besides, Liang et al. pointed out that in patients with atherosclerosis and ECs treated with ox-LDL, the level of GAS5 was upregulated while miRNA-26a was downregulated. GAS5 can directly bind to miRNA-26a, resulting in ECs apoptosis and impaired autophagy flux (111). Notably, miRNA−26a-5p overexpression suppresses cell apoptosis by inactivating the TLR4/ NF-κB signaling pathway in human aortic ECs exposed to ox-LDL (119). Therefore, GAS5 in EVs derived from ox-LDL-induced THP-1 cells transferred into ECs causing cellular apoptosis via downregulation of miRNA-26a-5p and activation of the TLR4/ NF-κB pathways and the upregulation of apoptotic factors including Caspases.
EVs Mediate Intercellular Communication Between Different Cells in Atherosclerosis
Atherosclerosis is a complex vascular disorder involving multiple pathological processes. ECs dysfunction, macrophages migration and foam cells formation, VSMCs proliferation and migration, and platelets activation contribute to the progression of atherosclerosis (120). A wide variety of stimuli trigger endothelial dysfunction to activate the NF-κB pathway, such as the release of numerous molecules, including ICAM-1, VCAM-1, and monocyte chemoattractant protein-1 (MCP-1) which are tightly associated with ECs dysfunction in atherosclerosis (121). Moreover, ICAM-1, VCAM-1, and NETs are implicated in leukocytes adhesion and migration to ECs and accumulating leukocytes and ECs secrete numerous inflammatory factors. Meanwhile, monocytes differentiate into macrophages and ultimately form foam cells (122). Besides, numerous chemokines and growth factors stimulate VSMCs proliferation and migration. EVs secreted by multiple cell types, including ECs, VSMCs, immune cells, and MSCs mediate intercellular communication in atherosclerosis. Accumulating evidence demonstrates that EVs, as well as EVs carrying miRNAs and lncRNAs, mediate cell-to-cell communication (Figure 2).
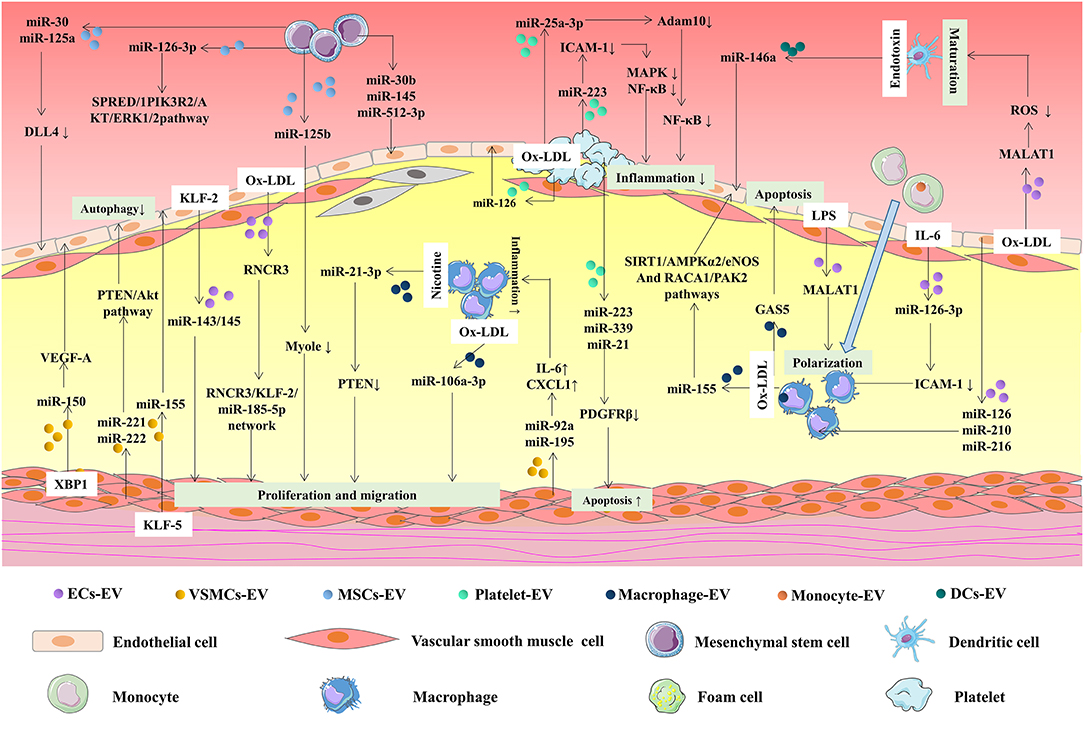
Figure 2. MiRNAs and lncRNAs in EVs mediate intercellular communication in atherosclerosis. EVs, secreted by different cells, including ECs, VSMCs, and immune cells, are implicated in atherosclerosis via transferring miRNAs and lncRNAs. KLF2-expressing ECs-derived EVs reduced atherosclerotic lesion formation in ApoE (-/-) mice via delivering miR-143/145. Ox-LDL-induced ECs secret EVs regulate VSMCs proliferation and migration through the delivery of RNCR3. On the contrary, XBP1 splicing in VSMCs can control ECs migration via EVs inclusion of miRNA-150 and miRNA-150-driven VEGF-A/VEGFR/PI3K/Akt pathway. MiR-221/222 in VSMCs-derived EVs repressed HUVECs autophagy via PTEN/Akt pathway. MiR-155 was elevated in KLF5-overexpressing VSMCs-derived EVs and enhanced atherosclerosis development via inhibiting ECs proliferation, migration, and re-endothelialization. ECs-derived EVs carrying miRNA-126, miRNA-210, miRNA-216, and MALAT1 alleviate macrophages polarization and infiltration. The transfer of miRNA-126-3P to macrophages can reduce atherosclerotic plaque area via EVs. Overexpression of miR-155 in EVs from ox-LDL-induced macrophages regulated ECs inflammation by modulating Sirt1/AMPKα2/eNOS and RAC1/PAK2 pathways. GAS5 was upregulated in THP-1 cells-derived EVs after oxLDL stimulation. GAS5 was involved in regulating ECs apoptosis and stimulating atherosclerosis. MiRNA-92a and miRNA-195 were increased in EVs from VSMCs and they could regulate inflammatory reaction through enhancing the expression of IL-6 and CXCL1 in macrophages. MiR-21-3p in EVs from nicotine-treated macrophages accelerate atherosclerosis by PTEN-mediated VSMC migration and proliferation. MiR-106a-3p was increased in EVs from ox-LDL stimulated THP-1 and it could promote cell proliferation of VSMCs. DCs-derived EVs transferred miRNA-146a into HUVECs to protect HUVECs from a second stimulation of TNF-α. Loss of MALAT1 in EVs from ox-LDL-induced VECs promoted DCs maturation in atherosclerosis development via Nrf2 pathway. MiR-25-3p was upregulated in thrombin-induced platelet-derived EVs and alleviated ox-LDL-induced ECs inflammation. Activated platelet-derived EVs promote ECs proliferation by transferring miRNA-126 and enhancing the production of VEGF, bFGF, and TGF-β1. Thrombin stimulated platelet-derived EVs repressed the production of PDGFRβ in VSMCs via the delivery of miR-223, miR-339, and miR-21, promoting cell apoptosis. MiRNA-30 and miRNA-125a in EVs released from MSCs reduced the expression of DLL4 in ECs, mediating angiogenesis. MSCs-derived EVs promote the proliferation and migration of ECs through delivering miRNA-126-3P. Besides, the transfer of miR-125b from MSCs-derived EVs to VSMCs inhibited VSMC proliferation by inhibiting Myo1e.
MiRNAs and LncRNAs in EVs Mediated ECs-VSMCs Cross-Talk
ECs dysfunction in response to various stimuli such as hyperglycemia and hypercholesterolemia are central to atherogenesis. Notably, the NF-κB signaling plays an important role in ECs dysfunction, which is critical in the development of atherosclerosis. VSMCs proliferation and migration are important in atherosclerosis progression. ECs dysfunction is closely related to the function and migration of VSMCs through the delivery of EVs and their cargos. For example, our previous studies indicated that EVs from HUVECs exposed to high glucose induce VSMCs calcification (123). The intercellular communication between ECs and VSMCs through miRNAs and lncRNAs in EVs is bidirectional.
ECs-derived EVs mediate VSMCs phenotype. ECs treated with low or oscillatory shear stress in atherogenesis areas increase the activation of activator protein 1 (AP-1) and NF-κB signaling, leading to pro-inflammation and pro-coagulation during atherosclerosis. Several studies report that the level of KLF2 in ECs plays anti-inflammatory and anti-thrombosis roles in atherosclerosis by enhancing the generation of anti-inflammatory and anti-coagulant molecules, including thrombomodulin (TM) and eNOS. Moreover, KLF2 inhibits AP-1 and NF-κB activity (124). Emerging evidence supports that ECs in KLF2-/- show a normal endothelium, but dysfunctional and disorganized in VSMCs, indicating that KLF2 is involved in the migration of VSMCs (125). EVs secreted by HUVECs exposed to KLF2 or shear stress exhibit a high expression of miRNA-143/145 which is implicated in the control of VSMCs phenotype. The transfer of miRNA-143/145 from ECs to VSMCs modulate VSMCs migration and proliferation through EVs (27). Besides, lncRNAs in EVs have an impact on the progression and development of atherosclerosis. RNCR3 is highly expressed in ECs exposed to ox-LDL and human atherosclerotic lesions which are vital to atherogenesis. RNCR3 acts as an atheroprotective regulator via the RNCR3/ KLF2/miR-185-5p network. EVs containing RNCR3 from ECs transferred to VSMCs, mediates VSMCs proliferation and migration, and regulates VSMCs function giving rise to atherogenic phenotype (105). Besides, HUVECs stimulated VSMCs phenotype switch and proliferation via the transfer of LINC01005 in EVs (106).
VSMCs-derived EVs can influence ECs angiogenesis and vessel stabilization via miRNAs transfer. VSMC-derived EVs transfer miRNA-150 to ECs and trigger ECs migration by promoting the generation of VEGF-A and activation of the VEGF-A/VEGFR/PI3K/Akt pathway (35). Besides, co-cultured human aortic SMCs with ECs represses ECs autophagy activity by transferring miRNA-221/222 from human aortic SMCs-derived EVs (37). In contrast, EVs secreted by VSMCs with high expression of KLF5 deliver miRNA-155 to ECs, suppressing the proliferation, migration, and re-endothelialization of ECs. Besides, the transfer of miRNA-155 can enhance vascular endothelial permeability by destroying the tight junctions and integrity of endothelium barriers. KLF5, a zinc-finger-containing transcription factor, is vital to VSMCs proliferation and migration. Increasing evidence suggests that overexpression of KLF5 in VSMCs is significantly associated with ECs function during the process of atheromata (36).
MiRNAs and LncRNAs in EVs Mediated ECs-Macrophages Cross-Talk
EVs mediate ECs and monocytes/macrophages communication via miRNAs and lncRNA. ECs exposed to various stimuli like ox-LDL trigger ECs injury and apoptosis, and recruit monocytes to adhere to ECs and differentiate into macrophages. Interestingly, ECs dysfunction induces inflammatory responses which are associated with monocytes/macrophages activation, eventually causing atherosclerotic plaque formation.
On the one hand, ECs-derived EVs can mediate monocyte activation and vascular inflammation. MiRNA-10a encapsulated in EVs transferred from ECs to monocytes, inhibits inflammatory responses (22). Additionally, miRNA-126-3P in EVs was decreased in IL-6-treated ECs, which increases the adhesion of THP-1 cells, while high levels of miRNA-126-3P show opposite effects (26). ECs-derived EVs inhibit macrophages accumulation and ameliorate atherosclerotic lesions through the delivery of miRNA-126 and miRNA-210 (29). Furthermore, MALAT1 was upregulated in EVs from ECs treated with ox-LDL and transferred into macrophages to induce M2 macrophage polarization (107). On the other hand, EVs derived from macrophages regulate ECs migration and angiogenesis. MiRNA-150 shed by EVs from THP-1 cells shuttled into ECs, thus promoting cell migration (43). Besides, EVs from M1 macrophages show an anti-angiogenesis effect on ECs. MiRNA-155 in EVs transferred from macrophages into ECs alleviated angiogenesis and stimulated cellular dysfunction (45). Moreover, the transfer of GAS5 in ECs-derived EVs modulates ECs and THP-1 cells apoptosis (112).
MiRNAs and LncRNAs in EVs Mediated VSMCs-Macrophages Cross-Talk
EVs contained miRNAs and lncRNAs mediate the intercellular communication between VSMCs and macrophages during atherosclerotic progression. VSMCs-derived EVs carrying miRNA-92a and miRNA-195 transfer to macrophages and promote inflammation and atherogenesis (122). Moreover, EVs secreted by macrophages transfer miRNAs to VSMCs and mediate VSMCs function and migration. Nicotine-induced macrophages-derived EVs stimulated proliferation and migration of VSMCs and led to atherosclerotic development through the delivery of miRNA-21-3p (38). Besides, miRNA-106a-3p which is highly expressed in EVs from THP-1 macrophages treated with ox-LDL and incubated with VSMCs strengthens VSMCs proliferation and inhibits VSMCs apoptosis (40). Additionally, miRNA-222 significantly increases in M1 macrophages-derived EVs and are taken up by VSMCs, promoting VSMCs proliferation and migration through the target CDKN1B and CDKN1C (46).
MiRNAs and LncRNAs in EVs Mediated DCs-ECs Cross-Talk
EVs mediate the communication between DCs and ECs, by inhibiting endothelial inflammation and preventing atherosclerotic deterioration. Existing evidence suggests that DCs-derived EVs are implicated in ECs inflammatory responses and atherogenesis through regulating NF κB signaling via TNF-α. MiRNA-16 and miRNA-21 of EVs isolated from DCs incubated with HUVECs suppress NF-κB pathway activation and repress endothelial inflammation associated with TNF-α (48). Moreover, the high level of miRNA-146a in mature DCs-derived EVs shuttled into HUVECs to protect HUVECs from a second stimulation by EVs from DCs, indicating that EVs from DCs play a negative role in mediating endothelial inflammation (49). Overexpression of MALAT1 in HUVECs-derived EVs secreted to DCs represses DCs maturation and attenuates ROS deposition. Preconditioning with ox-LDL, HUVECs exhibit low expression levels of MALAT1 promoting DCs maturation and atherosclerosis development (108).
MiRNAs and LncRNAs in EVs Mediated Platelets-ECs and VSMCs Cross-Talk
EVs mediate the interaction among platelets, ECs, and VSMCs, inhibit endothelial inflammation and VSMCs proliferation, thereby, showing a protective effect in atherogenesis. Platelet-derived EVs containing miRNAs shuttle into ECs to modulate endothelial inflammation. It has been reported that miRNA-25-3p is significantly increased in EVs derived from thrombin-induced platelet and isolated EVs co-culture with coronary vascular ECs alleviate ox-LDL stimulated endothelial inflammation, lipid deposition, and fibrosis (52). EVs from platelets transfer miRNA-126 to HUVECs to improve migration and proliferation via upregulating angiogenic factors (53). Thrombin-activated platelets-derived EVs enhance ECs proliferation through the delivery of miR-142-3p (54). Platelets-derived EVs containing miRNA Let-7a can shuttled into HUVECs to increase angiogenesis (99). Platelets secreted EVs deliver microRNA-223 to ECs, and regulates cell apoptosis by attenuating IGF-1R expression (55). MiRNA-223 packaged in EVs shuttle into HUVECs alleviates endothelial inflammation by repressing the production of ICAM-1 and inhibiting MAPK and NF-κB pathways (56). Interestingly, platelet-derived EVs rich in miRNA-223, miRNA-339, and miRNA-21 uptake by VSMCs suppress cell proliferation by ameliorating the level of PDGFRβ (57).
MiRNAs and LncRNAs in EVs Mediated MSCs-ECs and VSMCs Cross-Talk
EVs are involved in the exchange of information between MSCs and other cells, including ECs, VSMCs, and macrophages, regulating ECs angiogenesis and proliferation, VSMCs proliferation and migration, and macrophages polarization and infiltration. MiRNA-30b and miRNA-126-3P of EVs from MSCs delivered into recipient HUVECs increase cell tube-like structure formation and migration, and regulate angiogenesis (59, 102). Similarly, MSCs-derived EVs containing miRNA-125a, which enhances endothelial tip cell formation (61). Besides, SCs-derived EVs enhance ECs angiogenesis through transferring miRNA-31 (60). The delivery of miRNA-126 from MSCs to ECs promote ECs function via EVs (65). MiRNA-145 in EVs derived from MSCs alleviate HUVECs migration and reduce atherosclerotic plaque. Additionally, MSC-derived EVs containing miRNA-125b transferred to VSMCs ameliorates cell proliferation and migration by inhibiting Myo1e (62). MSCs-derived EVs shuttle into M2 macrophages, and induces cell polarization and infiltration through the transfer of miRNA-let7 (71).
Application of miRNAs and lncRNAs in EVs in Atherosclerosis
Atherosclerosis is a major cause of high morbidity and mortality worldwide. Therefore, there is a need for studies to explore various diagnostic biomarkers and therapies for atherosclerosis. For early diagnosis, the identified biomarkers must be sensitive, precise, and specific differentiate atherosclerotic patients from healthy individuals (84). MiRNAs and lncRNAs packaged in EVs are indisputably implicated in the development, diagnosis, and potential therapy of atherosclerosis (120). Considering the increasing attention of EVs containing miRNAs and lncRNAs in atherosclerosis progression, they have emerged as novel diagnostic biomarkers and potential therapeutic targets for atherosclerosis.
MiRNAs and LncRNAs in EVs as Diagnostic Biomarkers in Atherosclerosis
The roles of EVs carrying miRNAs and lncRNAs as potential biomarkers must, however, be carefully considered. For atherosclerosis diagnosis, the miRNAs include miRNA-192-5p, miRNA-16-5p, and miRNA-122-5p of EVs are reported to be reliable biomarkers for predicting intracranial atherosclerotic disease (126). Additionally, the high levels of miRNA-30e and miRNA-92a in EVs in 42 atherosclerosis patients, indicating that they may serve as promising diagnostic biomarkers for atherosclerosis (127). Evidently, miRNAs and lncRNAs in EVs are associated with atherosclerosis, including miRNA-21, miRNA-150, miRNA-155, miRNA-126, miRNA-210, miRNA-505, miRNA-146a, miR-133, miRNA-223, miRNA-125, miRNA-let7, RNCR3, MALAT1, GAS5, and HIF1A-AS1 which provide a strong basis for their application as diagnostic markers for atherosclerosis. Furthermore, the changes in expression of miRNAs and lncRNAs should be explored in the treatment of atherosclerosis, since they might provide promising prognostic biomarkers.
As for diagnosis, miRNAs and lncRNAs in EVs have important advantages of serving as non-invasive biomarkers due to EVs are easily accessible and highly stabile in body fluids, such as blood, saliva, and urine (88). In addition, miRNAs and lncRNAs encapsulated in EVs exhibit better application compared with circulating miRNAs and lncRNAs, due to the high sensitivity and specificity of EVs (128). MiRNAs of EVs are not affected by RNASE-dependent degradation. Therefore, they can stably detected in circulating plasma and serum, making them ideal biomarkers for clinical diagnostic applications (129). The level of miR-126 in EVs was positively correlated with the severity of coronary artery stenosis in acute myocardial infarction patients (130). Besides, miR-30e and miR-92a from plasma EVs could be used as novel biomarkers for atherosclerosis diagnosis (127). However, the application of miRNAs and lncRNAs carried by EVs as diagnosis biomarkers has not been clinically explored. Despite miRNAs and lncRNAs in EVs exert pivotal roles in the development, diagnosis, and potential therapy of atherosclerosis, there are still some limitations. First of all, the commonly used isolation technologies for EVs mainly included ultracentrifugation, size-based isolation techniques, and immunoaffinity capture techniques (131). Different EVs purification strategies may affect the content of miRNAs in EVs (132). Secondly, the large number of variable miRNAs carried by EVs may regulate various signaling pathways and have an overall effect on recipient cells. Therefore, it is difficult to thoroughly understand the function of miRNAs in EVs (133). Thirdly, it is difficult to identify miRNAs in a single EVs, or to measure the number of specific miRNAs carried by EVs when EVs are present in low abundance (134). Additionally, there is still no consensus on the optimal panel of EVs contained miRNAs or lncRNAs to use as diagnostic markers for atherosclerosis. The screening and development of the optimal panel require extensive and accurate studies involving substantial samples, which is time-consuming and labor-intensive. Therefore, there is a need to validate these miRNAs and lncRNAs packaged in EVs as clinical diagnostic and prognostic biomarkers in atherosclerosis using large cohort studies. More work is required in identifying the roles of miRNAs and lncRNAs in EVs as novel biomarkers in clinical management as well as therapies of atherosclerosis.
MiRNAs and LncRNAs in EVs as Therapeutic Targets in Atherosclerosis
A better understanding of the underlying mechanisms of miRNAs and lncRNAs in EVs in atherosclerosis may allow for the development of EVs-based therapies against atherosclerosis. Numerous lines of evidence supports that EVs emerge as drug delivery carriers in the treatment of many disorders, for example, miRNAs-loaded EVs can pass through the blood-brain barrier and repress BACE1 in Alzheimer's disease (135). EVs can effectively transfer Ldlr mRNA to stabilize atherosclerosis plaques in Ldlr-/- mouse models (136). To date, there are over 200 preclinical studies associated with the role of EVs as therapeutic tools in different disease models (19). EVs and contained miRNAs and lncRNAs are tightly associated with atherosclerosis, thus, they might provide safe and reliable therapeutic targets for atherosclerosis treatment by overexpressing or knocking down prospective miRNAs and lncRNAs in EVs. Paeonol modulates monocytes-derived EVs inhibit NLRP3 activation and ameliorates the accumulation of inflammatory cytokines through the transfer of microRNA-223. Therefore, high expression of miRNA-223 in EVs might be a potentially novel strategy for the treatment of atherosclerosis (137). As discussed, miRNA-143/145 can also be explored in the development of potential treatment targets through mediating ECs and VSMCs communication and abrogating atherosclerotic plaque. Besides, upregulation of lncRNAs in EVs, including RNCR3 and MALAT1 provide a strong basis for their application as potential therapeutic targets. Reducing the expression of miRNA-126-3p, miRNA-146a, and miRNA-155 support fresh therapeutic strategies against atherosclerosis (26, 28).
With respect to clinical therapy, miRNAs and lncRNAs in EVs have the potential to become therapeutic targets or drug delivery systems for atherosclerosis. On the one hand, EVs have the ability to target tissues or cells and penetrate biological barriers (138). One of the biggest advantages of EVs is that they are endogenous and can avoid causing adverse events such as immune responses. Besides, EVs, as a drug delivery medium, have essentially biocompatibility and non-immunogenic compared to other nano-delivery systems. On the other hand, there exist several obstacles. One of the major problems in implementing EV-based therapies is the low productivity of EVs (139). Besides, natural EVs are unable to effectively deliver drugs or targeted applications due to their inadequacy, such as poor stability and rapid elimination (140). Moreover, natural EVs have the handicap of poor targeting. Therefore, the EVs used for treatment should be self-derived and modified to form engineered EVs, so as to avoid the side effects of exogenous EVs and compensate for the defects of natural EVs. Additionally, EVs cannot be stored for a long time which makes it difficult for transportation and clinical therapy. Thus, more studies are required to explore novel EVs preservation techniques (131). Nevertheless, it is important to consider the various unpredictable side effects. Therefore, miRNAs and lncRNAs in EVs delivery into intended sites is a challenging but interesting endeavor that requires further exploration.
Perspectives and Conclusions
Atherosclerosis is implicated in intricate pathophysiological processes, including endothelial dysfunction, mononuclear macrophages infiltration, VSMCs proliferation and migration, and platelets activation. EVs secreted by multiple cell types have been demonstrated to participate in intercellular communication through the transfer of miRNAs and lncRNAs to recipient cells, inducing alterations in cell structure and function. Currently, numerous lines of studies have revealed the roles and mechanisms of miRNAs and lncRNAs in EVs as potential diagnostic biomarkers and therapeutic targets in atherosclerosis. In this review, we summarize the molecular and cellular mechanisms of different miRNAs and lncRNAs from diverse cells-derived EVs in the progression of atherosclerosis and discuss their potential applications in the diagnosis and treatment of atherosclerosis. Although the ability of EVs to deliver miRNAs and lncRNAs in atherosclerosis has aroused significant attention, there is a great need for more work to validate that miRNAs and lncRNAs in EVs serve as diagnostic biomarkers and therapeutic targets in atherosclerosis.
Author Contributions
HX and Y-QN collected the literature and wrote the manuscript. Y-SL conceived the idea and supervised the manuscript. All authors read and approved the final manuscript.
Funding
This work was supported by the National Natural Science Foundation of China (Nos. 82071593 and 81770833) and the Fundamental Research Funds for the Central Universities of Central South University (No. 2019zzts354).
Conflict of Interest
The authors declare that the research was conducted in the absence of any commercial or financial relationships that could be construed as a potential conflict of interest.
Publisher's Note
All claims expressed in this article are solely those of the authors and do not necessarily represent those of their affiliated organizations, or those of the publisher, the editors and the reviewers. Any product that may be evaluated in this article, or claim that may be made by its manufacturer, is not guaranteed or endorsed by the publisher.
References
1. Ross R. Atherosclerosis–an inflammatory disease. N Engl J Med. (1999) 340:115–26. doi: 10.1056/NEJM199901143400207
2. Chen YT, Yuan HX, Ou ZJ, Ou JS. Microparticles (exosomes) and atherosclerosis. Curr Atheroscler Rep. (2020) 22:23. doi: 10.1007/s11883-020-00841-z
3. Wang Y, Xie Y, Zhang A, Wang M, Fang Z, Zhang J. Exosomes: an emerging factor in atherosclerosis. Biomed Pharmacother. (2019) 115:108951. doi: 10.1016/j.biopha.2019.108951
4. Hafiane A, Daskalopoulou SS. Extracellular vesicles characteristics and emerging roles in atherosclerotic cardiovascular disease. Metabolism. (2018) 85:213–22. doi: 10.1016/j.metabol.2018.04.008
5. van Niel G, D'Angelo G, Raposo G. Shedding light on the cell biology of extracellular vesicles. Nat Rev Mol Cell Biol. (2018) 19:213–28. doi: 10.1038/nrm.2017.125
6. Paone S, Baxter AA, Hulett MD, Poon IKH. Endothelial cell apoptosis and the role of endothelial cell-derived extracellular vesicles in the progression of atherosclerosis. Cell Mol Life Sci. (2019) 76:1093–106. doi: 10.1007/s00018-018-2983-9
7. Kowal J, Arras G, Colombo M, Jouve M, Morath JP, Primdal-Bengtson B, et al. Proteomic comparison defines novel markers to characterize heterogeneous populations of extracellular vesicle subtypes. Proc Natl Acad Sci USA. (2016) 113:E968–977. doi: 10.1073/pnas.1521230113
8. Cocucci E, Meldolesi J. Ectosomes and exosomes: shedding the confusion between extracellular vesicles. Trends Cell Biol. (2015) 25:364–72. doi: 10.1016/j.tcb.2015.01.004
9. Inal JM, Kosgodage U, Azam S, Stratton D, Antwi-Baffour S, Lange S. Blood/plasma secretome and microvesicles. Biochim Biophys Acta. (2013) 1834:2317–25. doi: 10.1016/j.bbapap.2013.04.005
10. Akers JC, Gonda D, Kim R, Carter BS, Chen CC. Biogenesis of extracellular vesicles (EV): exosomes, microvesicles, retrovirus-like vesicles, and apoptotic bodies. J Neurooncol. (2013) 113:1–11. doi: 10.1007/s11060-013-1084-8
11. Di Vizio D, Morello M, Dudley AC, Schow PW, Adam RM, Morley S, et al. Large oncosomes in human prostate cancer tissues and in the circulation of mice with metastatic disease. Am J Pathol. (2012) 181:1573–84. doi: 10.1016/j.ajpath.2012.07.030
12. Thery C, Witwer KW, Aikawa E, Alcaraz MJ, Anderson JD, Andriantsitohaina R, et al. Minimal information for studies of extracellular vesicles 2018 (MISEV2018): a position statement of the International Society for Extracellular Vesicles and update of the MISEV2014 guidelines. J Extracell Vesicles. (2018) 7:1535750. doi: 10.1080/20013078.2018.1535750
13. Deng W, Tang T, Hou Y, Zeng Q, Wang Y, Fan W, et al. Extracellular vesicles in atherosclerosis. Clin Chim Acta. (2019) 495:109–17. doi: 10.1016/j.cca.2019.04.051
14. Escola JM, Kleijmeer MJ, Stoorvogel W, Griffith JM, Yoshie O, Geuze HJ. Selective enrichment of tetraspan proteins on the internal vesicles of multivesicular endosomes and on exosomes secreted by human B-lymphocytes. J Biol Chem. (1998) 273:20121–7. doi: 10.1074/jbc.273.32.20121
15. Hemler ME. Tetraspanin proteins mediate cellular penetration, invasion, and fusion events and define a novel type of membrane microdomain. Annu Rev Cell Dev Biol. (2003) 19:397–422. doi: 10.1146/annurev.cellbio.19.111301.153609
16. Kugeratski FG, Hodge K, Lilla S, McAndrews KM, Zhou X, Hwang RF, et al. Quantitative proteomics identifies the core proteome of exosomes with syntenin-1 as the highest abundant protein and a putative universal biomarker. Nat Cell Biol. (2021) 23:631–41. doi: 10.1038/s41556-021-00693-y
17. Lu TX, Rothenberg ME. MicroRNA. J Allergy Clin Immunol. (2018) 141:1202–7. doi: 10.1016/j.jaci.2017.08.034
18. St Laurent G, Wahlestedt C, Kapranov P. The landscape of long noncoding RNA classification. Trends Genet. (2015) 31:239–51. doi: 10.1016/j.tig.2015.03.007
19. Elahi FM, Farwell DG, Nolta JA, Anderson JD. Preclinical translation of exosomes derived from mesenchymal stem/stromal cells. Stem Cells. (2020) 38:15–21. doi: 10.1002/stem.3061
20. Reiss AB, Vernice NA, Siegart NM, De Leon J, Kasselman LJ. Exosomes in cholesterol metabolism and atherosclerosis. Cardiovasc Hematol Disord Drug Targets. (2017) 17:185–94. doi: 10.2174/1871529X18666180103124443
21. Thum T, Mayr M. Review focus on the role of microRNA in cardiovascular biology and disease. Cardiovasc Res. (2012) 93:543–4. doi: 10.1093/cvr/cvs085
22. Njock MS, Cheng HS, Dang LT, Nazari-Jahantigh M, Lau AC, Boudreau E, et al. Endothelial cells suppress monocyte activation through secretion of extracellular vesicles containing antiinflammatory microRNAs. Blood. (2015) 125:3202–12. doi: 10.1182/blood-2014-11-611046
23. Liang HZ, Li SF, Zhang F, Wu MY, Li CL, Song JX, et al. Effect of endothelial microparticles induced by hypoxia on migration and angiogenesis of human umbilical vein endothelial cells by delivering MicroRNA-19b. Chin Med J. (2018) 131:2726–33. doi: 10.4103/0366-6999.245271
24. Jansen F, Yang X, Hoelscher M, Cattelan A, Schmitz T, Proebsting S, et al. Endothelial microparticle-mediated transfer of MicroRNA-126 promotes vascular endothelial cell repair via SPRED1 and is abrogated in glucose-damaged endothelial microparticles. Circulation. (2013) 128:2026–38. doi: 10.1161/CIRCULATIONAHA.113.001720
25. Zernecke A, Bidzhekov K, Noels H, Shagdarsuren E, Gan L, Denecke B, et al. Delivery of microRNA-126 by apoptotic bodies induces CXCL12-dependent vascular protection. Sci Signal. (2009) 2:ra81. doi: 10.1126/scisignal.2000610
26. Ohta M, Kihara T, Toriuchi K, Aoki H, Iwaki S, Kakita H, et al. IL-6 promotes cell adhesion in human endothelial cells via microRNA-126-3p suppression. Exp Cell Res. (2020) 393:112094. doi: 10.1016/j.yexcr.2020.112094
27. Hergenreider E, Heydt S, Tréguer K, Boettger T, Horrevoets AJ, Zeiher AM, et al. Atheroprotective communication between endothelial cells and smooth muscle cells through miRNAs. Nat Cell Biol. (2012) 14:249–56. doi: 10.1038/ncb2441
28. Sun HX, Zeng DY, Li RT, Pang RP, Yang H, Hu YL, et al. Essential role of microRNA-155 in regulating endothelium-dependent vasorelaxation by targeting endothelial nitric oxide synthase. Hypertension. (2012) 60:1407–14. doi: 10.1161/HYPERTENSIONAHA.112.197301
29. Ong SG, Lee WH, Huang M, Dey D, Kodo K, Sanchez-Freire V, et al. Cross talk of combined gene and cell therapy in ischemic heart disease: role of exosomal microRNA transfer. Circulation. (2014) 130(11Suppl.1):S60–9. doi: 10.1161/CIRCULATIONAHA.113.007917
30. Jansen F, Yang X, Baumann K, Przybilla D, Schmitz T, Flender A, et al. Endothelial microparticles reduce ICAM-1 expression in a microRNA-222-dependent mechanism. J Cell Mol Med. (2015) 19:2202–14. doi: 10.1111/jcmm.12607
31. Chen L, Hu L, Li Q, Ma J, Li H. Exosome-encapsulated miR-505 from ox-LDL-treated vascular endothelial cells aggravates atherosclerosis by inducing NET formation. Acta Biochim Biophys Sin. (2019) 51:1233–41. doi: 10.1093/abbs/gmz123
32. Santulli G, Sardu C, Shu J, Matarese A, Wang X. Abstract 802: cardiomyocyte-derived exosomal MicroRNAs regulates post-infarction inflammation and myofibroblast phenoconversion. Circul Res. (2019) 125(Suppl.1):802. doi: 10.1161/res.125.suppl_1.802
33. Gao S, Wassler M, Zhang L, Li Y, Wang J, Zhang Y, et al. MicroRNA-133a regulates insulin-like growth factor-1 receptor expression and vascular smooth muscle cell proliferation in murine atherosclerosis. Atherosclerosis. (2014) 232:171–9. doi: 10.1016/j.atherosclerosis.2013.11.029
34. Izarra A, Moscoso I, Levent E, Cañón S, Cerrada I, Díez-Juan A, et al. miR-133a enhances the protective capacity of cardiac progenitors cells after myocardial infarction. Stem Cell Reports. (2014) 3:1029–42. doi: 10.1016/j.stemcr.2014.10.010
35. Zhao Y, Li Y, Luo P, Gao Y, Yang J, Lao KH, et al. XBP1 splicing triggers miR-150 transfer from smooth muscle cells to endothelial cells via extracellular vesicles. Sci Rep. (2016) 6:28627. doi: 10.1038/srep28627
36. Zheng B, Yin WN, Suzuki T, Zhang XH, Zhang Y, Song LL, et al. Exosome-mediated miR-155 transfer from smooth muscle cells to endothelial cells induces endothelial injury and promotes atherosclerosis. Mol Ther. (2017) 25:1279–94. doi: 10.1016/j.ymthe.2017.03.031
37. Li L, Wang Z, Hu X, Wan T, Wu H, Jiang W, et al. Human aortic smooth muscle cell-derived exosomal miR-221/222 inhibits autophagy via a PTEN/Akt signaling pathway in human umbilical vein endothelial cells. Biochem Biophys Res Commun. (2016) 479:343–50. doi: 10.1016/j.bbrc.2016.09.078
38. Zhu J, Liu B, Wang Z, Wang D, Ni H, Zhang L, et al. Exosomes from nicotine-stimulated macrophages accelerate atherosclerosis through miR-21-3p/PTEN-mediated VSMC migration and proliferation. Theranostics. (2019) 9:6901–19. doi: 10.7150/thno.37357
39. Bouchareychas L, Duong P, Covarrubias S, Alsop E, Phu TA, Chung A, et al. Macrophage exosomes resolve atherosclerosis by regulating hematopoiesis and inflammation via MicroRNA cargo. Cell Rep. (2020) 32:107881. doi: 10.1016/j.celrep.2020.107881
40. Liu Y, Zhang WL, Gu JJ, Sun YQ, Cui HZ, Bu JQ, et al. Exosome-mediated miR-106a-3p derived from ox-LDL exposed macrophages accelerated cell proliferation and repressed cell apoptosis of human vascular smooth muscle cells. Eur Rev Med Pharmacol Sci. (2020) 24:7039–50.
41. Nguyen MA, Karunakaran D, Geoffrion M, Cheng HS, Tandoc K, Perisic Matic L, et al. Extracellular vesicles secreted by atherogenic macrophages transfer MicroRNA to inhibit cell migration. Arterioscler Thromb Vasc Biol. (2018) 38:49–63. doi: 10.1161/ATVBAHA.117.309795
42. Zhang YG, Song Y, Guo XL, Miao RY, Fu YQ, Miao CF, et al. Exosomes derived from oxLDL-stimulated macrophages induce neutrophil extracellular traps to drive atherosclerosis. Cell Cycle. (2019) 18:2674–84. doi: 10.1080/15384101.2019.1654797
43. Zhang Y, Liu D, Chen X, Li J, Li L, Bian Z, et al. Secreted monocytic miR-150 enhances targeted endothelial cell migration. Mol Cell. (2010) 39:133–44. doi: 10.1016/j.molcel.2010.06.010
44. Huang RS, Hu GQ, Lin B, Lin ZY, Sun CC. MicroRNA-155 silencing enhances inflammatory response and lipid uptake in oxidized low-density lipoprotein-stimulated human THP-1 macrophages. J Investig Med. (2010) 58:961–7. doi: 10.2310/JIM.0b013e3181ff46d7
45. Liu S, Chen J, Shi J, Zhou W, Wang L, Fang W, et al. M1-like macrophage-derived exosomes suppress angiogenesis and exacerbate cardiac dysfunction in a myocardial infarction microenvironment. Basic Res Cardiol. (2020) 115:22. doi: 10.1007/s00395-020-0781-7
46. Wang Z, Zhu H, Shi H, Zhao H, Gao R, Weng X, et al. Exosomes derived from M1 macrophages aggravate neointimal hyperplasia following carotid artery injuries in mice through miR-222/CDKN1B/CDKN1C pathway. Cell Death Dis. (2019) 10:422. doi: 10.1038/s41419-019-1667-1
47. Zhuang G, Meng C, Guo X, Cheruku PS, Shi L, Xu H, et al. A novel regulator of macrophage activation: miR-223 in obesity-associated adipose tissue inflammation. Circulation. (2012) 125:2892–903. doi: 10.1161/CIRCULATIONAHA.111.087817
48. Li X, Li X, Lin J, Sun X, Ding Q. Exosomes derived from low-intensity pulsed ultrasound-treated dendritic cells suppress tumor necrosis factor-induced endothelial inflammation. J Ultrasound Med. (2019) 38:2081–91. doi: 10.1002/jum.14898
49. Zhong X, Gao W, Wu R, Liu H, Ge J. Dendritic cell exosome-shuttled miRNA146a regulates exosome-induced endothelial cell inflammation by inhibiting IRAK-1: a feedback control mechanism. Mol Med Rep. (2019) 20:5315–23. doi: 10.3892/mmr.2019.10749
50. Alexander M, Hu R, Runtsch MC, Kagele DA, Mosbruger TL, Tolmachova T, et al. Exosome-delivered microRNAs modulate the inflammatory response to endotoxin. Nat Commun. (2015) 6:7321. doi: 10.1038/ncomms8321
51. Lin B, Xie W, Zeng C, Wu X, Chen A, Li H, et al. Transfer of exosomal microRNA-203-3p from dendritic cells to bone marrow-derived macrophages reduces development of atherosclerosis by downregulating Ctss in mice. Aging (Albany NY). (2021) 13:15638–58. doi: 10.18632/aging.103842
52. Yao Y, Sun W, Sun Q, Jing B, Liu S, Liu X, et al. Platelet-derived exosomal MicroRNA-25-3p inhibits coronary vascular endothelial cell inflammation through adam10 via the NF-κB signaling pathway in ApoE(-/-) mice. Front Immunol. (2019) 10:2205. doi: 10.3389/fimmu.2019.02205
53. Sun Y, Liu XL, Zhang D, Liu F, Cheng YJ, Ma Y, et al. Platelet-derived exosomes affect the proliferation and migration of human umbilical vein endothelial cells via miR-126. Curr Vasc Pharmacol. (2019) 17:379–87. doi: 10.2174/1570161116666180313142139
54. Bao H, Chen YX, Huang K, Zhuang F, Bao M, Han Y, et al. Platelet-derived microparticles promote endothelial cell proliferation in hypertension via miR-142-3p. FASEB J. (2018) 32:3912–23. doi: 10.1096/fj.201701073R
55. Pan Y, Liang H, Liu H, Li D, Chen X, Li L, et al. Platelet-secreted microRNA-223 promotes endothelial cell apoptosis induced by advanced glycation end products via targeting the insulin-like growth factor 1 receptor. J Immunol. (2014) 192:437–46. doi: 10.4049/jimmunol.1301790
56. Li J, Tan M, Xiang Q, Zhou Z, Yan H. Thrombin-activated platelet-derived exosomes regulate endothelial cell expression of ICAM-1 via microRNA-223 during the thrombosis-inflammation response. Thromb Res. (2017) 154:96–105. doi: 10.1016/j.thromres.2017.04.016
57. Tan M, Yan HB, Li JN, Li WK, Fu YY, Chen W, et al. Thrombin stimulated platelet-derived exosomes inhibit platelet-derived growth factor receptor-beta expression in vascular smooth muscle cells. Cell Physiol Biochem. (2016) 38:2348–65. doi: 10.1159/000445588
58. Gidlöf O, van der Brug M, Ohman J, Gilje P, Olde B, Wahlestedt C, et al. Platelets activated during myocardial infarction release functional miRNA, which can be taken up by endothelial cells and regulate ICAM1 expression. Blood. (2013) 121:3908–17. doi: 10.1182/blood-2012-10-461798
59. Gong M, Yu B, Wang J, Wang Y, Liu M, Paul C, et al. Mesenchymal stem cells release exosomes that transfer miRNAs to endothelial cells and promote angiogenesis. Oncotarget. (2017) 8:45200–12. doi: 10.18632/oncotarget.16778
60. Kang T, Jones TM, Naddell C, Bacanamwo M, Calvert JW, Thompson WE, et al. Adipose-derived stem cells induce angiogenesis via microvesicle transport of miRNA-31. Stem Cells Transl Med. (2016) 5:440–50. doi: 10.5966/sctm.2015-0177
61. Liang X, Zhang L, Wang S, Han Q, Zhao RC. Exosomes secreted by mesenchymal stem cells promote endothelial cell angiogenesis by transferring miR-125a. J Cell Sci. (2016) 129:2182–9. doi: 10.1242/jcs.170373
62. Wang D, Gao B, Yue J, Liu F, Liu Y, Fu W, et al. Exosomes from mesenchymal stem cells expressing miR-125b inhibit neointimal hyperplasia via myosin IE. J Cell Mol Med. (2019) 23:1528–40. doi: 10.1111/jcmm.14060
63. Yu C, Tang W, Lu R, Tao Y, Ren T, Gao Y. Human adipose-derived mesenchymal stem cells promote lymphocyte apoptosis and alleviate atherosclerosis via miR-125b-1-3p/BCL11B signal axis. Ann Palliat Med. (2021) 10:2123–33. doi: 10.21037/apm-21-49
64. Lin F, Zhang S, Liu X, Wu M. Mouse bone marrow derived mesenchymal stem cells-secreted exosomal microRNA-125b-5p suppresses atherosclerotic plaque formation via inhibiting Map4k4. Life Sci. (2021) 274:119249. doi: 10.1016/j.lfs.2021.119249
65. Pan Q, Wang Y, Lan Q, Wu W, Li Z, Ma X, et al. Exosomes derived from mesenchymal stem cells ameliorate hypoxia/reoxygenation-injured ECs via transferring MicroRNA-126. Stem Cells Int. (2019) 2019:2831756. doi: 10.1155/2019/2831756
66. Yang W, Yin R, Zhu X, Yang S, Wang J, Zhou Z, et al. Mesenchymal stem-cell-derived exosomal miR-145 inhibits atherosclerosis by targeting JAM-A. Mol Ther Nucleic Acids. (2021) 23:119–31. doi: 10.1016/j.omtn.2020.10.037
67. Lin Y, Liu M, Chen E, Jiang W, Shi W, Wang Z. Bone marrow-derived mesenchymal stem cells microvesicles stabilize atherosclerotic plaques by inhibiting NLRP3-mediated macrophage pyroptosis. Cell Biol Int. (2021) 45:820–30. doi: 10.1002/cbin.11526
68. Li Y, Yang R, Guo B, Zhang H, Zhang H, Liu S, et al. Exosomal miR-301 derived from mesenchymal stem cells protects myocardial infarction by inhibiting myocardial autophagy. Biochem Biophys Res Commun. (2019) 514:323–8. doi: 10.1016/j.bbrc.2019.04.138
69. Xing X, Li Z, Yang X, Li M, Liu C, Pang Y, et al. Adipose-derived mesenchymal stem cells-derived exosome-mediated microRNA-342-5p protects endothelial cells against atherosclerosis. Aging. (2020) 12:3880–98. doi: 10.18632/aging.102857
70. Chen S, Zhou H, Zhang B, Hu Q. Exosomal miR-512-3p derived from mesenchymal stem cells inhibits oxidized low-density lipoprotein-induced vascular endothelial cells dysfunction via regulating Keap1. J Biochem Mol Toxicol. (2021) 35:1–11. doi: 10.1002/jbt.22767
71. Li J, Xue H, Li T, Chu X, Xin D, Xiong Y, et al. Exosomes derived from mesenchymal stem cells attenuate the progression of atherosclerosis in ApoE(-/-) mice via miR-let7 mediated infiltration and polarization of M2 macrophage. Biochem Biophys Res Commun. (2019) 510:565–72. doi: 10.1016/j.bbrc.2019.02.005
72. Lombardo G, Dentelli P, Togliatto G, Rosso A, Gili M, Gallo S, et al. Activated Stat5 trafficking via endothelial cell-derived extracellular vesicles controls IL-3 pro-angiogenic paracrine action. Sci Rep. (2016) 6:25689. doi: 10.1038/srep25689
73. van Balkom BW, de Jong OG, Smits M, Brummelman J, den Ouden K, de Bree PM, et al. Endothelial cells require miR-214 to secrete exosomes that suppress senescence and induce angiogenesis in human and mouse endothelial cells. Blood. (2013) 121:3997–4006. doi: 10.1182/blood-2013-02-478925
74. Zhu Y, Xian X, Wang Z, Bi Y, Chen Q, Han X, et al. Research progress on the relationship between atherosclerosis and inflammation. Biomolecules. (2018) 8:30080. doi: 10.3390/biom8030080
75. Yang X, Chang Y, Wei W. Endothelial dysfunction and inflammation: immunity in rheumatoid arthritis. Mediators Inflamm. (2016) 2016:6813016. doi: 10.1155/2016/6813016
76. Zhang Z, Wang M, Xue SJ, Liu DH, Tang YB. Simvastatin ameliorates angiotensin II-induced endothelial dysfunction through restoration of Rho-BH4-eNOS-NO pathway. Cardiovasc Drugs Ther. (2012) 26:31–40. doi: 10.1007/s10557-011-6351-3
77. Bennett MR, Sinha S, Owens GK. Vascular smooth muscle cells in atherosclerosis. Circ Res. (2016) 118:692–702. doi: 10.1161/CIRCRESAHA.115.306361
78. Cordes KR, Sheehy NT, White MP, Berry EC, Morton SU, Muth AN, et al. miR-145 and miR-143 regulate smooth muscle cell fate and plasticity. Nature. (2009) 460:705–10. doi: 10.1038/nature08195
79. Climent M, Quintavalle M, Miragoli M, Chen J, Condorelli G, Elia L. TGFβ triggers miR-143/145 transfer from smooth muscle cells to endothelial cells, thereby modulating vessel stabilization. Circ Res. (2015) 116:1753–64. doi: 10.1161/CIRCRESAHA.116.305178
80. Deng L, Blanco FJ, Stevens H, Lu R, Caudrillier A, McBride M, et al. MicroRNA-143 activation regulates smooth muscle and endothelial cell crosstalk in pulmonary arterial hypertension. Circ Res. (2015) 117:870–83. doi: 10.1161/CIRCRESAHA.115.306806
81. Kapustin AN, Chatrou ML, Drozdov I, Zheng Y, Davidson SM, Soong D, et al. Vascular smooth muscle cell calcification is mediated by regulated exosome secretion. Circ Res. (2015) 116:1312–23. doi: 10.1161/CIRCRESAHA.116.305012
82. Zhao G, Fu Y, Cai Z, Yu F, Gong Z, Dai R, et al. Unspliced XBP1 confers VSMC homeostasis and prevents aortic aneurysm formation via FoxO4 interaction. Circ Res. (2017) 121:1331–45. doi: 10.1161/CIRCRESAHA.117.311450
83. Moulton KS, Li M, Strand K, Burgett S, McClatchey P, Tucker R, et al. PTEN deficiency promotes pathological vascular remodeling of human coronary arteries. JCI Insight. (2018) 3. doi: 10.1172/jci.insight.97228
84. Lu M, Yuan S, Li S, Li L, Liu M, Wan S. The exosome-derived biomarker in atherosclerosis and its clinical application. J Cardiovasc Transl Res. (2019) 12:68–74. doi: 10.1007/s12265-018-9796-y
85. Suzuki T, Sawaki D, Aizawa K, Munemasa Y, Matsumura T, Ishida J, et al. Kruppel-like factor 5 shows proliferation-specific roles in vascular remodeling, direct stimulation of cell growth, and inhibition of apoptosis. J Biol Chem. (2009) 284:9549–57. doi: 10.1074/jbc.M806230200
86. Hansson GK, Libby P. The immune response in atherosclerosis: a double-edged sword. Nat Rev Immunol. (2006) 6:508–19. doi: 10.1038/nri1882
87. Moore KJ, Tabas I. Macrophages in the pathogenesis of atherosclerosis. Cell. (2011) 145:341–55. doi: 10.1016/j.cell.2011.04.005
88. Wang C, Li Z, Liu Y, Yuan L. Exosomes in atherosclerosis: performers, bystanders, biomarkers, and therapeutic targets. Theranostics. (2021) 11:3996–4010. doi: 10.7150/thno.56035
89. Wu G, Zhang J, Zhao Q, Zhuang W, Ding J, Zhang C, et al. Molecularly engineered macrophage-derived exosomes with inflammation tropism and intrinsic heme biosynthesis for atherosclerosis treatment. Angew Chem Int Ed Engl. (2020) 59:4068–74. doi: 10.1002/anie.201913700
90. Shikatani EA, Besla R, Ensan S, Upadhye A, Khyzha N, Li A, et al. c-Myb exacerbates atherosclerosis through regulation of protective IgM-producing antibody-secreting cells. Cell Rep. (2019) 27:2304–12.e2306. doi: 10.1016/j.celrep.2019.04.090
91. Wang L, Jia Q, Xinnong C, Xie Y, Yang Y, Zhang A, et al. Role of cardiac progenitor cell-derived exosome-mediated microRNA-210 in cardiovascular disease. J Cell Mol Med. (2019) 23:7124–31. doi: 10.1111/jcmm.14562
92. Karshovska E, Wei Y, Subramanian P, Mohibullah R, Geißler C, Baatsch I, et al. HIF-1α (Hypoxia-Inducible Factor-1α) Promotes Macrophage Necroptosis by Regulating miR-210 and miR-383. Arterioscler Thromb Vasc Biol. (2020) 40:583–96. doi: 10.1161/ATVBAHA.119.313290
93. Qiao XR, Wang L, Liu M, Tian Y, Chen T. MiR-210-3p attenuates lipid accumulation and inflammation in atherosclerosis by repressing IGF2. Biosci Biotechnol Biochem. (2020) 84:321–9. doi: 10.1080/09168451.2019.1685370
94. Ismail N, Wang Y, Dakhlallah D, Moldovan L, Agarwal K, Batte K, et al. Macrophage microvesicles induce macrophage differentiation and miR-223 transfer. Blood. (2013) 121:984–95. doi: 10.1182/blood-2011-08-374793
95. Grammatikakis I, Abdelmohsen K, Gorospe M. Posttranslational control of HuR function. Wiley Interdiscip Rev RNA. (2017) 8. doi: 10.1002/wrna.1372
96. Huang X, Zhang H, Guo X, Zhu Z, Cai H, Kong X. Insulin-like growth factor 2 mRNA-binding protein 1 (IGF2BP1) in cancer. J Hematol Oncol. (2018) 11:88. doi: 10.1186/s13045-018-0628-y
97. Koltsova EK, Ley K. How dendritic cells shape atherosclerosis. Trends Immunol. (2011) 32:540–7. doi: 10.1016/j.it.2011.07.001
98. Palumbo R, Gaetano C, Melillo G, Toschi E, Remuzzi A, Capogrossi MC. Shear stress downregulation of platelet-derived growth factor receptor-beta and matrix metalloprotease-2 is associated with inhibition of smooth muscle cell invasion and migration. Circulation. (2000) 102:225–30. doi: 10.1161/01.CIR.102.2.225
99. Anene C, Graham AM, Boyne J, Roberts W. Platelet microparticle delivered microRNA-Let-7a promotes the angiogenic switch. Biochim Biophys Acta Mol Basis Dis. (2018) 1864:2633–43. doi: 10.1016/j.bbadis.2018.04.013
100. Li F, Guo X, Chen SY. Function and therapeutic potential of mesenchymal stem cells in atherosclerosis. Front Cardiovasc Med. (2017) 4:32. doi: 10.3389/fcvm.2017.00032
101. Lobov IB, Renard RA, Papadopoulos N, Gale NW, Thurston G, Yancopoulos GD, et al. Delta-like ligand 4 (Dll4) is induced by VEGF as a negative regulator of angiogenic sprouting. Proc Natl Acad Sci USA. (2007) 104:3219–24. doi: 10.1073/pnas.0611206104
102. Qu Q, Wang L, Bing W, Bi Y, Zhang C, Jing X, et al. miRNA-126-3p carried by human umbilical cord mesenchymal stem cell enhances endothelial function through exosome-mediated mechanisms in vitro and attenuates vein graft neointimal formation in vivo. Stem Cell Res Ther. (2020) 11:464. doi: 10.1186/s13287-020-01978-z
103. Kakogiannos N, Ferrari L, Giampietro C, Scalise AA, Maderna C, Ravà M, et al. JAM-A acts via C/EBP-α to promote claudin-5 expression and enhance endothelial barrier function. Circ Res. (2020) 127:1056–73. doi: 10.1161/CIRCRESAHA.120.316742
104. Zhao Z, Sun W, Guo Z, Zhang J, Yu H, Liu B. Mechanisms of lncRNA/microRNA interactions in angiogenesis. Life Sci. (2020) 254:116900. doi: 10.1016/j.lfs.2019.116900
105. Shan K, Jiang Q, Wang XQ, Wang YN, Yang H, Yao MD, et al. Role of long non-coding RNA-RNCR3 in atherosclerosis-related vascular dysfunction. Cell Death Dis. (2016) 7:e2248. doi: 10.1038/cddis.2016.145
106. Zhang Z, Yi D, Zhou J, Zheng Y, Gao Z, Hu X, et al. Exosomal LINC01005 derived from oxidized low-density lipoprotein-treated endothelial cells regulates vascular smooth muscle cell phenotypic switch. Biofactors. (2020) 46:743–53. doi: 10.1002/biof.1665
107. Huang C, Han J, Wu Y, Li S, Wang Q, Lin W, et al. Exosomal MALAT1 derived from oxidized low-density lipoprotein-treated endothelial cells promotes M2 macrophage polarization. Mol Med Rep. (2018) 18:509–15. doi: 10.3892/mmr.2018.8982
108. Li H, Zhu X, Hu L, Li Q, Ma J, Yan J. Loss of exosomal MALAT1 from ox-LDL-treated vascular endothelial cells induces maturation of dendritic cells in atherosclerosis development. Cell Cycle. (2019) 18:2255–67. doi: 10.1080/15384101.2019.1642068
109. Gao H, Wang X, Lin C, An Z, Yu J, Cao H, et al. Exosomal MALAT1 derived from ox-LDL-treated endothelial cells induce neutrophil extracellular traps to aggravate atherosclerosis. Biol Chem. (2020) 401:367–76. doi: 10.1515/hsz-2019-0219
110. Chen D, Wang K, Zheng Y, Wang G, Jiang M. Exosomes-mediated LncRNA ZEB1-AS1 facilitates cell injuries by miR-590-5p/ETS1 axis through the TGF-β/Smad pathway in oxidized low-density lipoprotein-induced human umbilical vein endothelial cells. J Cardiovasc Pharmacol. (2021) 77:480–90. doi: 10.1097/FJC.0000000000000974
111. Liang W, Fan T, Liu L, Zhang L. Knockdown of growth-arrest specific transcript 5 restores oxidized low-density lipoprotein-induced impaired autophagy flux via upregulating miR-26a in human endothelial cells. Eur J Pharmacol. (2019) 843:154–61. doi: 10.1016/j.ejphar.2018.11.005
112. Chen L, Yang W, Guo Y, Chen W, Zheng P, Zeng J, et al. Exosomal lncRNA GAS5 regulates the apoptosis of macrophages and vascular endothelial cells in atherosclerosis. PLoS ONE. (2017) 12:e0185406. doi: 10.1371/journal.pone.0185406
113. Hua Z, Ma K, Liu S, Yue Y, Cao H, Li Z. LncRNA ZEB1-AS1 facilitates ox-LDL-induced damage of HCtAEC cells and the oxidative stress and inflammatory events of THP-1 cells via miR-942/HMGB1 signaling. Life Sci. (2020) 247:117334. doi: 10.1016/j.lfs.2020.117334
114. Wang Y, Liang J, Xu J, Wang X, Zhang X, Wang W, et al. Circulating exosomes and exosomal lncRNA HIF1A-AS1 in atherosclerosis. Int J Clin Exp Pathol. (2017) 10:8383–8.
115. Dekker RJ, Boon RA, Rondaij MG, Kragt A, Volger OL, Elderkamp YW, et al. KLF2 provokes a gene expression pattern that establishes functional quiescent differentiation of the endothelium. Blood. (2006) 107:4354–63. doi: 10.1182/blood-2005-08-3465
116. Zhao G, Su Z, Song D, Mao Y, Mao X. The long noncoding RNA MALAT1 regulates the lipopolysaccharide-induced inflammatory response through its interaction with NF-κB. FEBS Lett. (2016) 590:2884–95. doi: 10.1002/1873-3468.12315
117. Rahman K, Vengrenyuk Y, Ramsey SA, Vila NR, Girgis NM, Liu J, et al. Inflammatory Ly6Chi monocytes and their conversion to M2 macrophages drive atherosclerosis regression. J Clin Invest. (2017) 127:2904–15. doi: 10.1172/JCI75005
118. Saha S, Buttari B, Panieri E, Profumo E, Saso L. An overview of Nrf2 signaling pathway and its role in inflammation. Molecules. (2020) 25. doi: 10.3390/molecules25225474
119. Zhong X, Zhang L, Li Y, Li P, Li J, Cheng G. Kaempferol alleviates ox-LDL-induced apoptosis by up-regulation of miR-26a-5p via inhibiting TLR4/NF-κB pathway in human endothelial cells. Biomed Pharmacother. (2018) 108:1783–9. doi: 10.1016/j.biopha.2018.09.175
120. Wang H, Xie Y, Salvador AM, Zhang Z, Chen K, Li G, et al. Exosomes: multifaceted messengers in atherosclerosis. Curr Atheroscler Rep. (2020) 22:57. doi: 10.1007/s11883-020-00871-7
121. Gimbrone MAJr, Garcia-Cardena G. Endothelial cell dysfunction and the pathobiology of atherosclerosis. Circ Res. (2016) 118:620–36. doi: 10.1161/CIRCRESAHA.115.306301
122. Ling H, Guo Z, Tan L, Cao Q, Song C. Stem cell-derived exosomes: role in the pathogenesis and treatment of atherosclerosis. Int J Biochem Cell Biol. (2021) 130:105884. doi: 10.1016/j.biocel.2020.105884
123. Li S, Zhan JK, Wang YJ, Lin X, Zhong JY, Wang Y, et al. Exosomes from hyperglycemia-stimulated vascular endothelial cells contain versican that regulate calcification/senescence in vascular smooth muscle cells. Cell Biosci. (2019) 9:1. doi: 10.1186/s13578-018-0263-x
124. Boon RA, Horrevoets AJ. Key transcriptional regulators of the vasoprotective effects of shear stress. Hamostaseologie. (2009) 29:39–40. doi: 10.1055/s-0037-1616937
125. Wu J, Bohanan CS, Neumann JC, Lingrel JB. KLF2 transcription factor modulates blood vessel maturation through smooth muscle cell migration. J Biol Chem. (2008) 283:3942–50. doi: 10.1074/jbc.M707882200
126. Jiang H, Toscano JF, Song SS, Schlick KH, Dumitrascu OM, Pan J, et al. Differential expression of circulating exosomal microRNAs in refractory intracranial atherosclerosis associated with antiangiogenesis. Sci Rep. (2019) 9:19429. doi: 10.1038/s41598-019-54542-y
127. Wang Z, Zhang J, Zhang S, Yan S, Wang Z, Wang C, et al. MiR-30e and miR-92a are related to atherosclerosis by targeting ABCA1. Mol Med Rep. (2019) 19:3298–304. doi: 10.3892/mmr.2019.9983
128. Raju S, Fish JE, Howe KL. MicroRNAs as sentinels and protagonists of carotid artery thromboembolism. Clin Sci. (2020) 134:169–92. doi: 10.1042/CS20190651
129. Lin J, Li J, Huang B, Liu J, Chen X, Chen XM, et al. Exosomes: novel biomarkers for clinical diagnosis. ScientificWorldJournal. (2015) 2015:657086. doi: 10.1155/2015/657086
130. Ling H, Guo Z, Shi Y, Zhang L, Song C. Serum exosomal MicroRNA-21, MicroRNA-126, and PTEN are novel biomarkers for diagnosis of acute coronary syndrome. Front Physiol. (2020) 11:654. doi: 10.3389/fphys.2020.00654
131. Zhang Y, Bi J, Huang J, Tang Y, Du S, Li P. Exosome: a review of its classification, isolation techniques, storage, diagnostic and targeted therapy applications. Int J Nanomedicine. (2020) 15:6917–34. doi: 10.2147/IJN.S264498
132. Rekker K, Saare M, Roost AM, Kubo AL, Zarovni N, Chiesi A, et al. Comparison of serum exosome isolation methods for microRNA profiling. Clin Biochem. (2014) 47:135–8. doi: 10.1016/j.clinbiochem.2013.10.020
133. Zhang J, Li S, Li L, Li M, Guo C, Yao J, et al. Exosome and exosomal microRNA: trafficking, sorting, and function. Genom Proteom Bioinformat. (2015) 13:17–24. doi: 10.1016/j.gpb.2015.02.001
134. Chevillet JR, Kang Q, Ruf IK, Briggs HA, Vojtech LN, Hughes SM, et al. Quantitative and stoichiometric analysis of the microRNA content of exosomes. Proc Natl Acad Sci USA. (2014) 111:14888–93. doi: 10.1073/pnas.1408301111
135. Alvarez-Erviti L, Seow Y, Yin H, Betts C, Lakhal S, Wood MJ. Delivery of siRNA to the mouse brain by systemic injection of targeted exosomes. Nat Biotechnol. (2011) 29:341–5. doi: 10.1038/nbt.1807
136. Li Z, Zhao P, Zhang Y, Wang J, Wang C, Liu Y, et al. Exosome-based Ldlr gene therapy for familial hypercholesterolemia in a mouse model. Theranostics. (2021) 11:2953–65. doi: 10.7150/thno.49874
137. Shi X, Xie X, Sun Y, He H, Huang H, Liu Y, et al. Paeonol inhibits NLRP3 mediated inflammation in rat endothelial cells by elevating hyperlipidemic rats plasma exosomal miRNA-223. Eur J Pharmacol. (2020) 885:173473. doi: 10.1016/j.ejphar.2020.173473
138. Saint-Pol J, Gosselet F, Duban-Deweer S, Pottiez G, Karamanos Y. Targeting and crossing the blood-brain barrier with extracellular vesicles. Cells. (2020) 9:40851. doi: 10.3390/cells9040851
139. Yamashita T, Takahashi Y, Takakura Y. Possibility of exosome-based therapeutics and challenges in production of exosomes eligible for therapeutic application. Biol Pharm Bull. (2018) 41:835–42. doi: 10.1248/bpb.b18-00133
Keywords: extracellular vesicles, microRNA, long non-coding RNA, endothelial cells, vascular smooth muscle cells, atherosclerosis
Citation: Xu H, Ni Y-Q and Liu Y-S (2021) Mechanisms of Action of MiRNAs and LncRNAs in Extracellular Vesicle in Atherosclerosis. Front. Cardiovasc. Med. 8:733985. doi: 10.3389/fcvm.2021.733985
Received: 06 July 2021; Accepted: 14 September 2021;
Published: 08 October 2021.
Edited by:
Tohru Fukai, Augusta University, United StatesReviewed by:
Alexander N. Kapustin, AstraZeneca, United KingdomPushpankur Ghoshal, Augusta University, United States
Copyright © 2021 Xu, Ni and Liu. This is an open-access article distributed under the terms of the Creative Commons Attribution License (CC BY). The use, distribution or reproduction in other forums is permitted, provided the original author(s) and the copyright owner(s) are credited and that the original publication in this journal is cited, in accordance with accepted academic practice. No use, distribution or reproduction is permitted which does not comply with these terms.
*Correspondence: You-Shuo Liu, liuyoushuo@csu.edu.cn
†These authors have contributed equally to this work