- 1Diretoria de Ensino e Pesquisa, Fundação Hospitalar de Hematologia e Hemoterapia do Amazonas (HEMOAM), Manaus, Brazil
- 2Programa de Pós-Graduação em Imunologia Básica e Aplicada, Instituto de Ciências Biológicas, Universidade Federal do Amazonas (UFAM), Manaus, Brazil
- 3Programa de Pós-Graduação em Ciências da Saúde, Instituto René Rachou - Fundação Oswaldo Cruz (FIOCRUZ) Minas, Belo Horizonte, Brazil
- 4Programa de Pós-Graduação em Ciências Aplicadas à Hematologia, Universidade do Estado do Amazonas (UEA), Manaus, Brazil
- 5Programa de Pós-Graduação em Medicina Tropical, UEA, Manaus, Brazil
- 6Instituto de Pesquisa Clínica Carlos Borborema, Fundação de Medicina Tropical Doutor Heitor Vieira Dourado (FMT-HVD), Manaus, Brazil
- 7Escola de Enfermagem de Manaus, UFAM, Manaus, Brazil
Recently, many discoveries have elucidated the cellular and molecular diversity in the leukemic microenvironment and improved our knowledge regarding their complex nature. This has allowed the development of new therapeutic strategies against leukemia. Advances in biotechnology and the current understanding of T cell-engineering have led to new approaches in this fight, thus improving cell-mediated immune response against cancer. However, most of the investigations focus only on conventional cytotoxic cells, while ignoring the potential of unconventional T cells that until now have been little studied. γδ T cells are a unique lymphocyte subpopulation that has an extensive repertoire of tumor sensing and may have new immunotherapeutic applications in a wide range of tumors. The ability to respond regardless of human leukocyte antigen (HLA) expression, the secretion of antitumor mediators and high functional plasticity are hallmarks of γδ T cells, and are ones that make them a promising alternative in the field of cell therapy. Despite this situation, in particular cases, the leukemic microenvironment can adopt strategies to circumvent the antitumor response of these lymphocytes, causing their exhaustion or polarization to a tumor-promoting phenotype. Intervening in this crosstalk can improve their capabilities and clinical applications and can make them key components in new therapeutic antileukemic approaches. In this review, we highlight several characteristics of γδ T cells and their interactions in leukemia. Furthermore, we explore strategies for maximizing their antitumor functions, aiming to illustrate the findings destined for a better mobilization of γδ T cells against the tumor. Finally, we outline our perspectives on their therapeutic applicability and indicate outstanding issues for future basic and clinical leukemia research, in the hope of contributing to the advancement of studies on γδ T cells in cancer immunotherapy.
Introduction
The leukemic microenvironment is composed of a complex and distinct network of factors that strongly support the growth and clonal dissemination of leukemic cells (LCs), thus impacting the patient’s clinical outcome (1–4). In this context, whereas conventional T cells (CD4+ or CD8+) and natural killer cells (NK) have been reported as “cytotoxicity mediators” capable of inducing tumor regression in vivo and controlling leukemic proliferation, several reports pointed to the fact that other T cells considered “unconventional” also have a high potential for coordinating the immune system and play complex and promising roles in cancer immunity (5–9). These antitumor responses are generally mediated by individual molecules with high or low diversity, such as the alpha-beta (αβ) or gamma-delta (γδ) T cell receptor (TCR) (10, 11).
In contrast to the αβ TCR, which is highly reactive to polymorphic molecules of the major histocompatibility complex (MHC), γδ TCR-expressing T cells perform their functions through recognition of antigens (Ags) presented by several monomorphic molecules, which in turn, promote a strong, rapid and effective response (12–14). In addition to being evolutionarily conserved, γδ T cells are important effectors, since they link innate and adaptive immune responses (11, 15, 16), and are highlighted as promising targets in cancer immunotherapy, especially for leukemias. These hematological malignancies are highly heterogeneous and are defined based on blast count, maturation stage and flow cytometry immunophenotyping, which allows them to be generally classified in acute lymphoblastic leukemia (ALL), chronic lymphocytic leukemia (CLL), acute myeloid leukemia (AML) and chronic myeloid leukemia (CML) (4, 17–23).
A potential therapy against these malignancies may depend on the mobilization and targeting of effector immune cells capable of producing antitumor factors and effectively killing LCs in different compartments with the absence of toxicity or alloreactivity. In this context, γδ T cells have unique attributes that support the promising development of an off-the-shelf cell therapy, as these lymphocytes provide a lasting and efficient response through mechanisms that include a higher cytotoxicity, functional plasticity, the production of several soluble molecules and responsiveness independent of MHC/HLA expression (24, 25). Although the tumor microenvironment (TME) and the adjacent LCs may develop several strategies to escape from γδ T cell-mediated immune surveillance, ex vivo or in vivo activation, the expansion and the genetic modification of these lymphocytes may increase their antileukemic reactivity and overcome suppression and resistance established by the TME (1, 26).
There is emerging evidence that γδ T cells exhibit persistent antitumor responses in different compartments in patients with leukemia and preserve healthy tissues; however, the adjacent mechanisms are still poorly understood (27–31). Therefore, γδ T cells are being translated into several clinical and therapeutic strategies targeting these hematological malignancies. Herein, we integrate the current knowledge regarding the diversity of γδ T cells and their associated potential in leukemia immune surveillance. Several approaches to improve their antitumor functions allow effective targeting against LCs and, therefore, will be discussed here. Finally, we emphasize open questions about γδ T cells and their subtypes, and also highlight their therapeutic applicability against leukemia. A better understanding of the functional relevance of γδ T cells in these malignancies has important implications, as we may be close to the unprecedented ascension of T cell-based therapies and their positioning as key-components for improving immunotherapy against cancer.
Untangling the Riddle of γδ T Cell Diversity
γδ T cells make up a lymphoid lineage that has relevant functions in tissues and blood circulation. Their development is regulated in the thymus, where they undergo maturation in different stages of thymic ontogeny (32–34). In this process, genetic rearrangements define the compromise and differentiation of double-negative thymocytes (CD4- and CD8-) for the T cell lineage-expressing γδ TCR (35–38). Subsequently, these cells migrate to peripheral blood (PB) and mucosal tissues, where they play key roles in the host’s immunity as primary effectors in the response against infections and cancer (15, 39, 40), preceding the responses of the αβ T cell lineage (41).
Currently, four major subtypes of human γδ T cells have been documented, which are defined by the TCR δ chain (i.e., Vδ1, Vδ2, Vδ3 and Vδ5) according to the Lefranc & Rabbits’s system nomenclature (42). Vδ1 and Vδ2 subtypes are the most predominant (11, 43–45). Vδ3 cells make up the majority of Vδ1-/Vδ2- subtypes and are rarely found in PB, although they are found in large numbers in the liver (46). Vδ5 cells also can be found in PB or tissues, but their functions are not entirely clear (43, 47–50). Here, we will focus on Vδ1, Vγ9Vδ2 and Vδ3 cells that are primarily thought to be involved in antileukemic responses.
Overall, γδ T cells constitute up to 10% of circulating CD3+ cells, though predominate among all tissue-resident T cells (51–54). Vδ1 and Vγ9Vδ2 subtypes represent ~10% and 90% of blood γδ T cells, respectively (51, 55, 56). While polyclonal Vδ1 cells are distributed throughout tissues, and exhibit adaptive-like behavior after detection of metabolic Ags and stress-induced molecules, Vδ2 cells predominate in blood and exhibit innate-like behavior after detecting molecules named phosphoantigens (pAgs) and other non-peptide antigens (11, 39, 57–59). A minor subtype of Vδ3 cells makes up ~0.2% of total circulating T cells and recognize CD1d and annexin-A2 (ANX2) (49, 60). In addition, little-known subtypes include Vδ5 cells, which detect the endothelial protein C receptor (EPCR), and other distinct clonal populations such as Vδ4, Vδ6, Vδ7 and Vδ8 (43, 61–63). Nonetheless, the enigma of the combinatorial and functional diversity of γδ TCRs has been partly revealed only for the Vδ1 and Vγ9Vδ2 subtypes (Table 1).
γδ T Cells and Leukemia: The Leukemic Microenvironment Matters
Basic scientific discoveries regarding leukemia have revealed that LCs adopt numerous mechanisms for evading immune surveillance (1, 76, 77). This cancer cell hallmark involves a heterogeneous group of components i.e., stromal and/or immune cells, specific receptors and soluble molecules that are present in the leukemic microenvironment, and which reprogram the hematopoietic niche and promote the clonal expansion of LCs in the bone marrow (BM). The subsequent tumor overload in this compartment results in the release of LCs into the blood, constituting two important sites of high leukemic clonal proliferation (1, 4, 26, 78). This is because LCs can bypass antitumor responses and, consequently, develop a high potential for making the environment extremely tolerogenic (79–81). For this, they adopt intrinsic and extrinsic strategies that impair the immune response of T cells and NK cells (26, 77, 82). Among these strategies, the negative regulation of HLA expression, high expression of inhibitory ligands for programmed cell death 1 (PD1), cytotoxic T lymphocyte antigen 4 (CTLA4) or lymphocyte activation gene 3 (LAG3) and the production of regulatory factors (i.e., cytokines, chemokines and inhibitory enzymes) are important changes that contribute to the inhibition of antitumor cells and the recruitment of suppressor cells that support the survival of LCs (83–99).
These established modifications in the leukemic microenvironment have great capacity for modifying cellular functions and for suppressing antileukemic responses – a consequence of the increase in components, such as regulatory T (Treg) cells, immunosuppressive myeloid cells (IMC), mesenchymal stromal cells (MSC) and inhibitory proteins (e.g., PD1 and CTLA4), which have a high regulatory influence (1, 26). γδ T cells are not exempt within this context, since they are susceptible to the effects of several molecules such as interleukin (IL)-4, IL-6, IL-13, IL-17, IL-23 and transforming growth factor beta (TGF-β) (100–105). These factors can play synergistic or pleiotropic roles, and can induce γδ T cell exhaustion or their polarization into a tumor-promoting phenotype (Figure 1), thus contributing to malignant progression (24, 106–109).
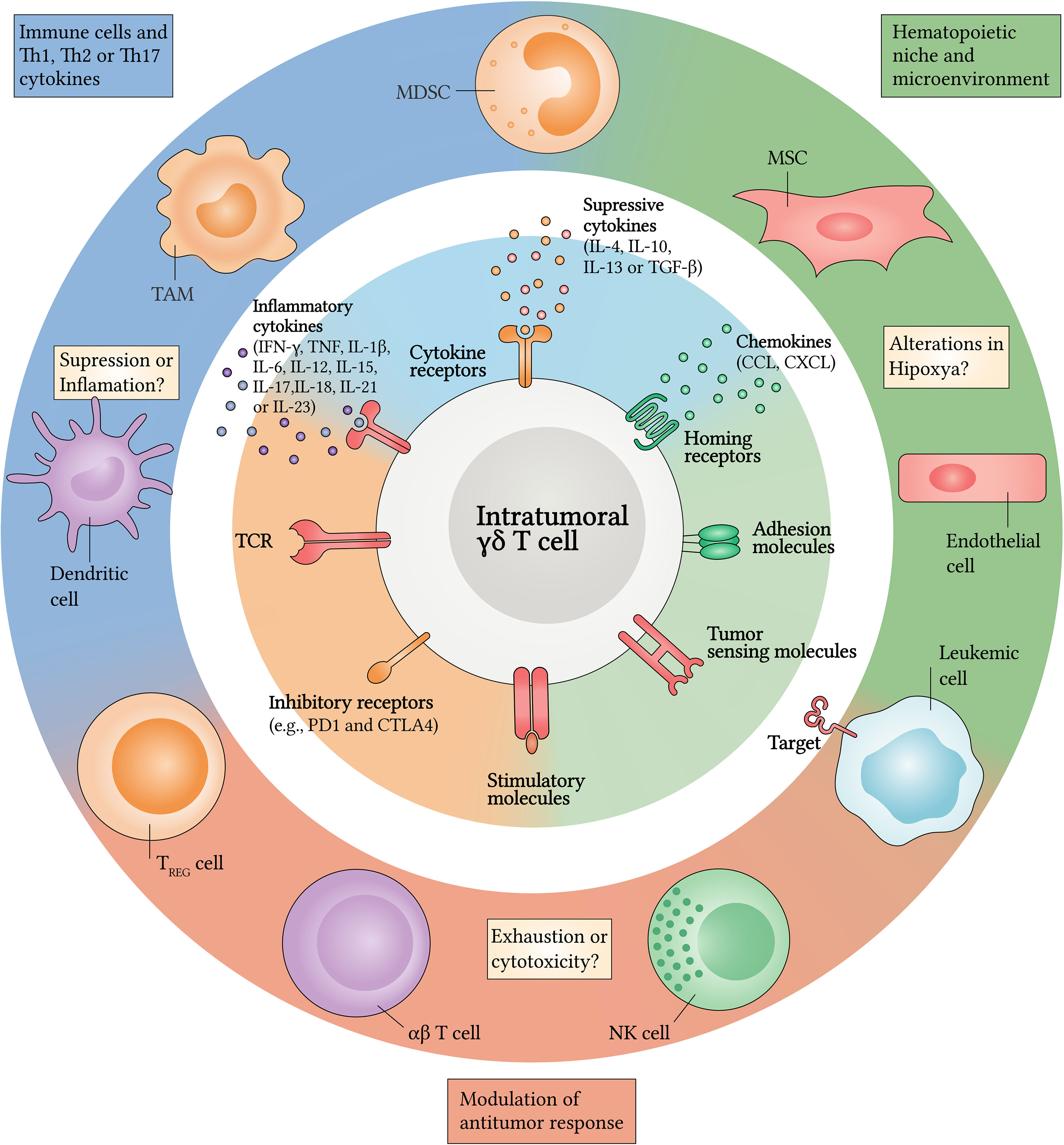
Figure 1 Crosstalk between γδ T cells and the leukemic microenvironment. Upon infiltrating the TME, γδ T cells are exposed to several persistent inflammatory and/or suppressive signals. Pathways implicated in crosstalk with the leukemic microenvironment can be classified into three general categories (center and inner circle): (i) cell-to-cell signals including antigen recognition by γδ T cell receptor (TCR), stimulatory or inhibitory molecules and/or tumor-sensing molecules; (ii) soluble factors such as cytokines and chemokines that will drive changes in expression levels of (iii) homing receptors and adhesion molecules. Several stromal and/or immune cells could be the source of many of these changes (outer circle). Among these, tumor-associated macrophages (TAM), myeloid-derived suppressor cells (MDSC), regulatory T (Treg) cells and dendritic cells (DC) retain their reprogramming potential into the TME by regulating inflammation or suppression through Th1, Th2 and Th17 cytokines. In addition, the hematopoietic niche can regulate hypoxia, responsible for supporting leukemic cells (LC) survival. Mesenchymal stromal cells (MSC) and endothelial cells can also express many factors that attract antitumor cells, such as γδ T cells, αβ T cells and NK cells which can exert cytotoxicity or undergo cell exhaustion after infiltrating the leukemic microenvironment. CCL, CC-chemokine ligand; CTLA4, cytotoxic T lymphocyte antigen 4; CXCL, CXC-chemokine ligand; PD1, programmed cell death protein 1.
Although LCs can escape the immune surveillance of αβ T cells and NK cells, they have several molecular targets that can be detected by γδ T cells; however, the crosstalk between these lymphocytes and the leukemic microenvironment is still poorly understood (Figure 1). Initially, γδ T cell responsiveness does not depend on MHC expression by LCs, whereas conventional αβ T cells require the MHC-Ag axis for activation to occur. The restricted specificity of conventional αβ TCR is also an important factor to be considered, as it is restricted to the detection of peptide antigens. In contrast, γδ TCR can identify stress-induced molecules, pAgs, lipid Ags and many other non-peptide molecules (110). In the context of leukemias, these attributes may offer an unconventional response pathway against these hematological malignancies.
Mobilization and Recruitment of γδ T Cells Into the TME
The pattern of γδ T cell migration and recruitment has not yet been fully characterized in the context of cancer and, therefore, represents an important question to be investigated. In humans, Vδ1 cells up-regulate the expression of CC-chemokine receptor 2 (CCR2) and CXC-chemokine receptor 3 (CXCR3) and infiltrate the TME. They are also activated by CC-chemokine ligand 12 (CCL2) and CXC-chemokine ligand 10 (CXCL10) and exhibit higher IFN-γ production (111, 112). Furthermore, Vδ1 cells express CXCR1 strongly and CCR5 weakly, whereas their Vγ9Vδ2 counterpart only exhibit strong expression of CCR5 (113). Interestingly, the CCR4/CCR8–CCL17/CCL22 pathway has also been shown to be an additional axis of chemoattractant signaling that recruits Vδ1 cells to the TME (114). It is important to note that Vγ9Vδ2 cells, besides retaining a high expression of CCR5, also express CCR3 and CXCR3, and can trigger antitumor responses in peripheral tissues during metastasis (115, 116).
A more accurate analysis of the profile of homing receptors expressed by γδ T cells would reveal how these cells migrate to the bone marrow microenvironment, for example. It is known that the mobilization of immune cells in this compartment is mediated mainly by the CXCR4-CXCL12 pathway, and it has been shown that CXCR4+ γδ T cells (preferably Vδ1 cells) respond to CXCL2 in vitro, but their intramedullary homing abilities have not yet been evaluated in the in vivo context of leukemia (117–119).
Despite this, many in vitro studies have shown that γδ T cells recognize and destroy leukemia blasts, but the complex network of interactions with the tumor environment in vivo remains poorly elucidated (120–122). A comparative analysis suggested that Vδ1 TCR-expressing γδ T cells were the most frequent subtype in the BM of pediatric patients with ALL (123). Subsequently, a low circulating γδ T cell frequency was detected in patients with AML before chemotherapy. Patients who regressed to minimal residual disease exhibited higher γδ T cell frequencies, whereas patients with a high leukemic burden exhibited decreased counts (27).
Transcriptomic analyses revealed an abundance of tumor-infiltrating Vγ9Vδ2 cells in cohorts of patients with leukemia (124). This high frequency was positively correlated with the survival of these patients. Although these results are encouraging, the method used to determine the relative proportions of these cells has failed to differentiate them correctly from αβ T cells and NK cells. As a result, this may have contributed to a higher γδ T cell count.
Vδ1 cells have been reported to have increased percentages in patients with CLL (28–31). A high frequency of these cells has been shown to be directly proportional to leukemic progression, that is, patients in more severe states exhibited higher Vδ1 cell counts when compared to healthy patients. This allows these lymphocytes to constitute the major γδ T cell subtype in the PB of these patients, where Vγ9Vδ2 cells generally predominate. This finding was also accompanied by cytotoxic Vδ1 cells with high granzyme (Gzm) B expression (28). Taken together, these data suggest that leukemia affects the γδ T cell frequency and that these cells have some influence during disease regression or progression.
On the other hand, a higher Vγ9Vδ2 cell frequency was associated with a poor prognosis in patients with untreated CLL (125). These lymphocytes showed a dysfunctional phenotype with reduced expression of NKG2D, although the derived LCs showed a high pAgs synthesis. This suggests that Vγ9Vδ2 cells expand in patients with leukemia and may exhibit functional exhaustion, apparently after long-term exposure to pAgs produced by LCs. Based on these reports, it becomes clear that the precise frequency of these cells and their clinical significance during the progression of leukemia is still controversial. In addition, the few studies carried out again suggest that the microenvironment of these malignancies has a strong influence on γδ T cells.
The Leukemic Cell–γδ T Cell Interactome
The sensing of LCs and γδ T cell activation are attributed to antigen recognition by γδ TCR and/or NK cell receptors (NKR), which include the natural killer group 2 member D (NKG2D) receptor, for example (Figure 2). Several reports have shown that LCs express several NKG2D ligands, which include stress-induced molecules, such as MHC class I chain-related protein A (MIC-A), MHC class I chain-related protein B (MIC-B) and UL16-binding proteins (ULBP) (71, 126, 127), while the lack of expression of these ligands is high related to immune evasion of LCs (128, 129). Besides this, some γδ T cell subtypes have a well-documented role in promoting NKG2D-mediated antileukemic responses.
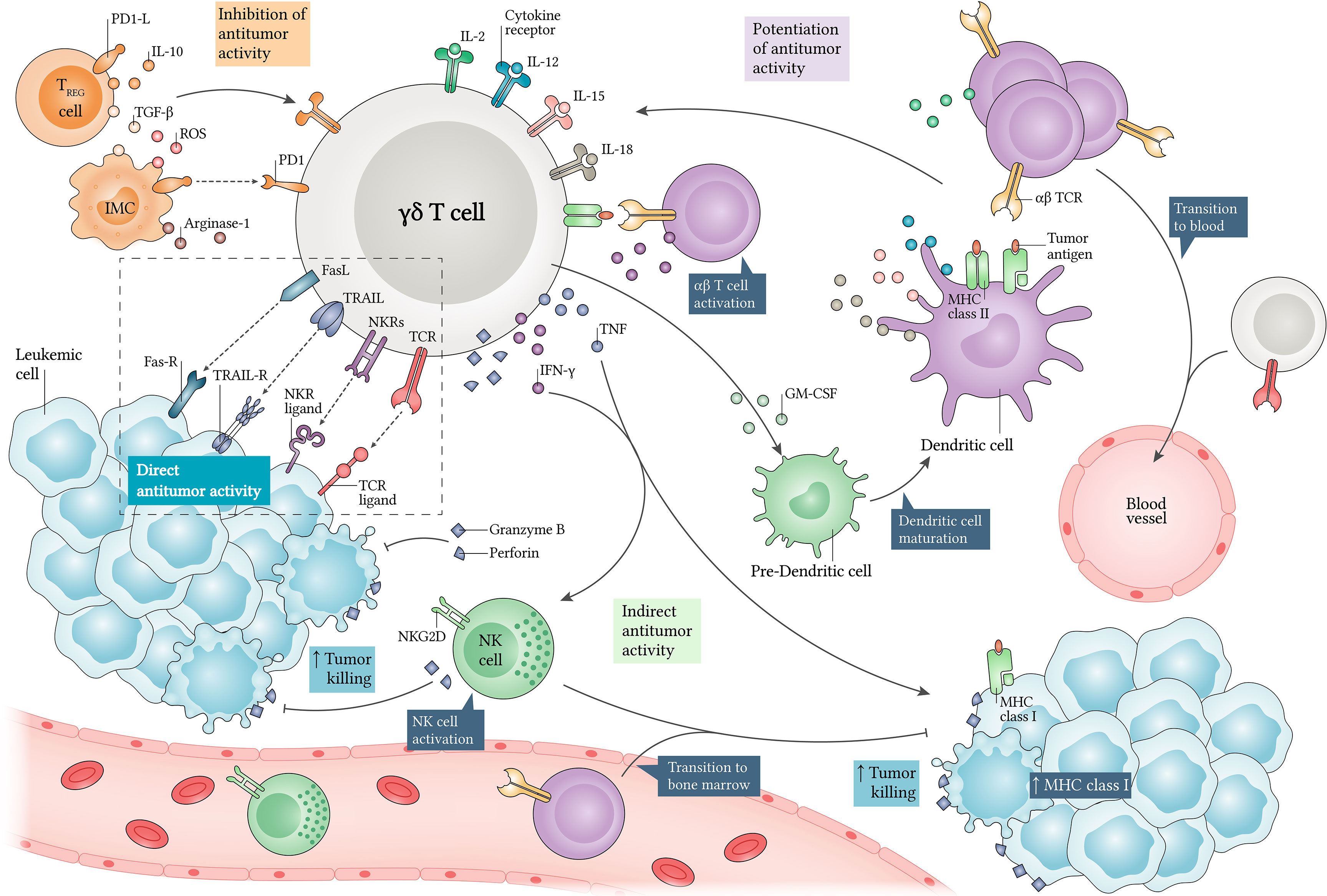
Figure 2 Antileukemic roles of γδ T cells and their regulation. γδ T cells kill leukemic cells (LC) via direct and indirect mechanisms. When identifying LCs through γδ TCR and co-receptors such as natural killer cell receptors (NKR), they secrete high levels of perforins and granzymes, mediating direct target killing. Additionally, γδ T cells produce interferon (IFN)-γ and tumor necrosis factor (TNF), which can increase MHC class I expression in LCs, and enhance αβ T cell-mediated cytotoxicity. IFN-γ release also allows NK cell activation, which can enhance tumor killing via NKG2D. Alternatively, γδ T cell-derived granulocyte-macrophage colony-stimulating factor (GM-CSF) can induce dendritic cell (DC) maturation, which in turn potentiates antitumor responses via interleukin (IL)-2, IL-12, IL-15 and IL-18. Thus, αβ or γδ T cells and NK cells can be recruited for exerting cytotoxicity in many compartments. Moreover, γδ T cells display APC functions and support αβ T cell and NK cell polarization towards an antitumor phenotype. In contrast, their cytotoxicity can be decreased by regulatory T (Treg) cells and immunosuppressive myeloid cells (IMC), since they produce several inhibitory factors such as IL-10, transforming growth factor β (TGF-β), reactive oxygen species (ROS) and Arginase-1. Finally, PD1-PD1L axis expression can regulate γδ T cell antitumor activities. APC, antigen-presenting cell; FasL, Fas ligand; Fas-R, Fas receptor; PD1, programmed cell death protein 1; PD1-L, PD1 ligand; TRAIL, tumor necrosis factor-related apoptosis-inducing ligand; TRAIL-R, TRAIL receptor.
Vδ1 cells recognize and destroy ULBP3+ MIC-A+ LCs and produce higher concentrations of interferon (IFN)-γ and tumor necrosis factor (TNF) in response to the tumor (29). In parallel, Vδ2 cells detect high regulated ULBP1 in LCs and this is indicative of tumor susceptibility to the cytotoxicity of these lymphocytes (130–132). It has also been established that Vδ1 and Vδ2 cells can destroy ULBP2+ LCs (133). Although an almost undetectable ULBP4 expression has been reported in leukemias (129, 134), remarkably, it has been shown that Vδ2 cells detect this molecule in LCs and respond with potent cytotoxicity (135). Therefore, the NKG2D receptor plays a key-role in γδ T cell-mediated immune surveillance in leukemia.
In addition to the expression of stress-induced molecules, an uncontrolled synthesis of metabolic molecules by cancer cells has emerged as a target that can be detected exclusively by reactive γδ T cells, such as the pAgs identified by Vγ9Vδ2 TCR. The pAgs detection mechanism involves butyrophilin (BTN) molecules, which are proteins related to the B7 family of co-stimulatory molecules. BTNs are essential prerequisites in γδ T cell activation, as they perform the intracellular capture of pAgs, undergo spatial and conformational changes in the membrane surface of target cells and consequently bind to the Vγ9 and Vδ2 TCR chains, sending strong stimulatory signals (72, 73). Thus, BTN3A2 has been shown to mediate the recognition of leukemic blasts even though it does not have the B30.2 intracellular domain, important in the internal pAgs uptake (136, 137). This suggests that BTN3A2 can recruit other isoforms, such as BTN3A1 or BTN3A3, and send activation signals through their intracellular domains (138). It is important to highlight that the presentation of pAgs by BTN proteins is highly regulated in LCs, whereas in normal cells the opposite occurs (139).
γδ T cells can also identify specific Ags in the context of monomorphic MHC class I molecules, such as the CD1 protein family (64). These proteins can mediate endogenous or exogenous lipid Ags recognition by γδ TCR and can be detected without loading with lipid Ags (140–142). Two major subtypes of CD1-reactive γδ T cells have been identified, namely Vδ1 and Vδ3 cells (60, 143). It is well established that these molecules are expressed in LCs and exhibit different expression patterns that are related to the leukemia subtype (144). In this context, γδ T cells may play important roles against LCs through the recognition of CD1 proteins and their isoforms.
In fact, CD1 proteins have established themselves as mediators of γδ T cell antitumor responses (145). It is important to note that the Vδ1 subtype represents a large proportion of these reactive cells (143), therefore it is suspected that Vδ1 cells can contribute to antitumor immunity through a CD1-dependent pathway. Recently, it was discovered that these cells with Vδ1 TCR, specifically Vγ4Vδ1 cells, detected CD1b in transfected LCs while they producing IFN-γ after recognition (146). These cells also recognized BTN-like (BTNL) proteins, such as BTNL3 and BTNL8, which suggests that CD1b-reactive γδ T cells may respond through the engagement and bispecific combination of CD1b and BTNLs (13).
CD1c recognition has also been investigated and although it does not yet have a well-defined description, it has been shown that this isoform can be recognized by γδ T cells (147). Their involvement in detection of LCs has not yet been reported, although it is clear whether CD1c is positively regulated in LCs (144), thus hypothesizing a possible role for CD1c in γδ T cell activation. In contrast, CD1d has been extensively investigated and the molecular insights about its recognition by γδ T cells have helped us significantly to understand its participation in immune surveillance (148). Interestingly, a high expression of CD1d has been associated with a poor prognosis in leukemia (149–152), but it should be noted that Vδ3 cells can expand and respond against CD1d+ target cells through a CD1d-restricted reactivity and with a potent secretion of effector molecules, such as IFN-γ (60, 153). Although initial studies suggest a CD1 protein-mediated cytotoxicity, questions regarding γδ T cell subtypes and their reactivity to these ligands, in the context of leukemia, still remain.
Monomorphic MHC class I-related protein (MR1) has gained prominence after many discoveries about its regulatory role in mucosal-associated invariant T (MAIT) cell biology and its expression in cancer. This protein can mediate the recognition of folate and riboflavin derived small metabolites (154, 155). In addition, recent reports support that MR1 can present not yet defined specific tumor Ags for MR1-restricted T cells (156, 157). As expected, it was also recently established that γδ TCR recognizes this molecule (65), although direct evidence for MR1+ LCs detection has not yet emerged. The identification of this protein by MR1-reactive T cells may mean a new therapeutic target for cancer immunotherapy and clearly places γδ T cells on the map as a promising and important T cell population.
As discussed above, detection of LCs appears to involve many Ags and stimulatory receptors and is not driven solely by the binding of γδ TCRs to their cognate ligands, but optionally requires the involvement of additional co-receptors and targets. Other NKRs, such as DNAX accessory molecule-1 (DNAM-1), can identify their ligands, such as the polyoma virus receptor (PVR) and nectin-2 molecules, in LCs (74, 158). Although a negative role has been reported for DNAM-1 expression in leukemia (159), this co-receptor is involved in the activation of γδ T cell cytotoxicity after interaction with their ligands in leukemic blasts. This is evidenced when Vγ9Vδ2 cells kill LCs in a TCR and DNAM-1 dependent fashion, with robust secretion of perforins and granzymes (74).
Notably, Vδ1 cells can lyse LCs via NKp30 and NKp44, which are highly regulated via the synergistic signal of cytokines and TCR (66). The expression of these natural cytotoxicity receptors (NCR) is related to higher granzyme production and cytotoxicity (66). It is important to highlight that NKp30 has been proven to be crucial for Vδ1 cell-mediated antitumor response. However, NKp30 and NKp44 are bound to an as yet undetermined target (66), ignoring their classic ligands, such as B7-H6 and MLL5 that bind to NKp30 and NKp44, respectively (67), suggesting an as yet unknown additional ligand. In addition, NKp46-expressing Vδ1 cells showed higher cytotoxic activity against LCs and IFN-γ and Gzm B production, while NKp46- γδ T cells showed reduced antileukemic activity (68). Despite this, the target ligand recognized by NKp46+ γδ T cells in LCs has not yet been demonstrated, although it is well established that cancer cells express ligands for this protein (69, 70).
Harnessing γδ T Cells Against Leukemia: From Marrow to Blood
γδ T cells are loaded with effector weapons of great potential for cancer immunotherapy (160). Findings in recent years point to important roles for these cells, highlighting them as potential predictive biomarkers, which justifies the current focus of studies on the nature of these cells and the TME (14, 25, 161). It is important to remember that several characteristics discussed here make γδ T cells potential candidates for innovative therapies against tumors and include: (i) activation in a TCR-independent manner; (ii) the ability to recognize Ags regardless of MHC/HLA expression; (iii) effector molecules production and direct and indirect cytotoxicity potentiation against cancer cells; and (iv) their role as antigen-presenting cells (APC) that induce the proliferation of antitumor cells (Figure 2).
Given the high responsiveness against LCs and the absence of toxicity or alloreactivity against the host (162, 163), the application of γδ T cells in leukemia treatment may mean a new advance in cancer immunity and immunotherapy. To make this possible, several strategies for γδ T cell handling have been developed and tested and have presented interesting data (Figure 3). The following subsections will focus on clinical trials and findings, as well as the activity of these cells in response to applied methods. Afterwards, we will discuss potential therapies that may specifically target γδ T cells and their subtypes, while summarizing the main approaches that are being explored to reach their clinical potential.
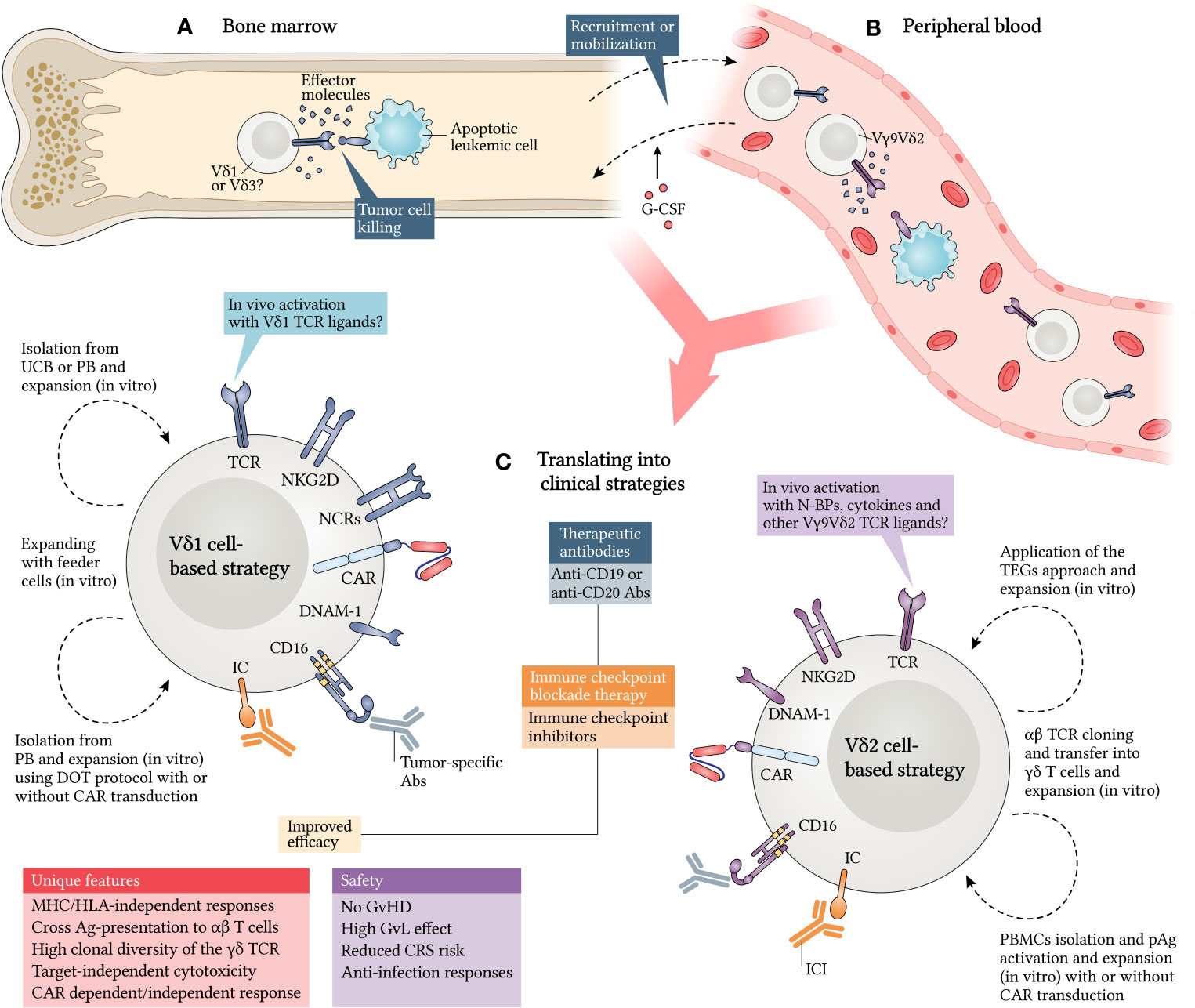
Figure 3 Translating γδ T cells into clinical strategies against leukemia. γδ T cells exert antitumor responses in different compartments and expressing distinct TCR patterns. Vδ1 and Vδ3 subtypes have been implicated as cytotoxic mediators in bone marrow (A), while Vγ9Vδ2 cells have been shown to respond mainly in peripheral blood (B). However, strategies are directed towards Vδ1 and Vδ2 subtypes, as they are the best known (C). Granulocyte colony-stimulating factor (G-CSF) was shown to be a potential adjuvant to mobilize γδ T cells for peripheral blood and enrich the graft. Additionally, Vδ1 cells can be isolated from UCB or PB and expanded in vitro using some approaches, such as the DOT protocol, already reviewed here. In vivo stimulation with Vδ1 TCR ligands may be a good alternative, but it remains poorly investigated. In parallel, Vδ2 cells can be isolated from PB and activated and/or expanded in vitro using pAgs. A new therapeutic concept consists in the cloning and transfer of γδ TCRs into αβ T cells (TEGs) and can enhance antileukemic responses. The fact is that many of these strategies give rise to γδ T cells that express several recognition receptors and have a higher capacity to target leukemic cells (LC), which can be further improved with chimeric antigen receptor (CAR) transduction. Moreover, the use of therapeutic antibodies (Abs), such as immune checkpoint inhibitors (ICI), anti-CD19 and anti-CD20 Abs, can also provide improved efficiency in potential approaches, since γδ T cells have unique features and an attractive degree of safety for their translation into clinical trials. CRS, cytokine release syndrome; DOT, Delta One T; PB, peripheral blood; IC, immune checkpoint; TEGs, T cells engineered to express a defined γδTCRs; UCB, umbilical cord blood.
Expanding γδ T Cells With pAgs, Drugs, Cytokines and Feeder Cells
Intrinsic synthesis of pAgs in cancer cells can be manipulated through pharmacological blockade mediated by aminobiphosphonates (N-BP), such as zoledronate (ZOL) and pamidronate (PAM), which interfere metabolically in the mevalonate pathway (164). The mechanism involved causes these compounds to block the enzymatic activity of farnesyl pyrophosphate synthase, which is present in this metabolic pathway. N-BP–induced interruption results in the intracellular accumulation of pAgs in cancer cells or APCs with subsequent recognition by γδ T cells and activation after cell-cell interaction (165–168). Cancer cell sensitizing with these compounds increases the tumor’s susceptibility to γδ T cell cytotoxicity, and this also applies in leukemia (132).
Some experimental evaluations took advantage of the γδ T cell recognition mechanism (directed to pAgs) to obtain a better in vitro or in vivo expansion of these lymphocytes and test their therapeutic efficacy. To date, these approaches have focused on ZOL, (E)-4-Hydroxy-3-methyl-but-2-enyl pyrophosphate (HMB-PP) and synthetic pAgs, such as bromohydrin pyrophosphate (BrHPP) (169, 170). These compounds are generally administered in combination with low cytokine doses such as IFNs, IL-2, IL-12, IL-15, IL-18 and IL-21. In addition, these approaches can induce an antitumor phenotype and the pronounced expression of associated receptors (171–176).
Vγ9Vδ2 cell expansion has become more accessible because, in addition to being the most prevalent subtype in PB (55, 56), it can also recognize a diversity of relatively well-defined target molecules (177). When these cells are treated with ZOL + IL-2 + IFN type I, their cytotoxic activity is increased and Vγ9Vδ2 cells may be able to efficiently destroy lymphoid and myeloid lineage LCs, as proposed by Watanabe et al. (171). In their study, γδ T cells were generated in vitro with ZOL + IL-2 for 14 days, and after this period they were activated with IFN type I for up to 3 days. Thus, the resultant γδ T cells were well expanded in the culture and showed a significant expression of CD69, TNF-related apoptosis-inducing ligand (TRAIL), IFN-γ and TNF, which suggests the acquisition of an activated phenotype and antileukemic reactivity.
In the same vein, sensitization with ZOL + Imatinib has also been shown to increase the cytotoxic synapse between Vγ9Vδ2 cells and LCs (178). Initially, Imatinib resistant or sensitive LCs had low susceptibility to γδ T cells, but in vitro treatment with ZOL + Imatinib was able to reverse this situation. The lysis of these LCs was mediated by TCR, NKG2D, TRAIL and perforins. This high cytotoxicity was dependent on ZOL, since it was observed that Vγ9Vδ2 cells exerted low antitumor activity that was slightly increased after sensitization of LCs. To validate these findings, it was further demonstrated that when Vγ9Vδ2 cells, ZOL and IL-2 are infused in a leukemia mouse model, they mediate tumor regression in vivo and confer greater survival in these mice (178).
The ability of N-BPs to invigorate exhausted Vγ9Vδ2 cells has also been reported in other investigations (179, 180) and appears to be a promising alternative for their use, given that a higher exhausted γδ T cell frequency has also observed in leukemia (27, 125). It is important to note that, in this context, these cells exhibit a low expression of CD107a, FcγRIII (CD16), IFN-γ and TNF, while B and T lymphocyte attenuator (BTLA), LAG3 and PD1 proteins are more highly regulated on their cell surface (179, 180). When cultured with allogeneic LCs, these lymphocytes had low cytotoxic activity, while γδ T cells from healthy patients responded efficiently (179). Notably, when Vγ9Vδ2 cells that were considered dysfunctional were cultured ex vivo with mature monocyte-derived dendritic cells (Mo-DC) and N-BPs for 8 days without the presence of LCs, the observed functional impairments could be reversed (179).
Ibrutinib has also been shown to activate γδ T cells against LCs, since Weerdt et al. reported that it was able to induce an antitumor phenotype (180). In their study, allogeneic and autologous γδ T cells were cultured with LCs. As already seen, γδ T cells from patients with leukemia proved to be dysfunctional in terms of cytokine production and cytotoxicity, while those from healthy patients had a strong antitumor activity (179). When Vγ9Vδ2 cells from both cases are treated with Ibrutinib, an effector Th1 phenotype and memory cells are induced. Overall, their antitumor properties can be recovered after ex vivo stimulation and after treatment with Ibrutinib, which binds to the IL-2–inducible T cell kinase molecule and promotes activation against LCs (180).
Other investigations have presented a new alternative: the combination of IL-15 plus N-BPs or pAgs promotes significantly greater expansion, high cytotoxicity and a more pronounced Th1 phenotype in γδ T cells, when compared to expansion methods using only IL-2 (174, 181, 182). IL-15 is a powerful growth factor for γδ T cells (183, 184) and can synergize with other molecules and enhance the antileukemic capacity of these cells (Figure 2), as we will highlight below.
The ex vivo tests carried out by Van Acker et al. (174) demonstrated that the administration of IL-15 + isopentenyl pyrophosphate (IPP) is able to improve γδ T cell cytotoxicity against LCs. In contrast, γδ T cells stimulated with IL-2 + IPP were more likely to deviate to a Th2 and Th17-like response phenotype when interacting with LCs (174). It is important to highlight that stimulation by IL-15 promoted a more robust IFN-γ and TNF secretion when compared to IL-2 stimulation. In addition, culturing these lymphocytes with IL-2, IL-15 and ZOL for 14 days critically enhanced the expansion rates to almost 1000-fold the total yield of viable cells, which showed a 590-fold increase in the γδ T cells cultured only with IL-2 + ZOL (174).
Interestingly, when IL-2 + IL-15 and ZOL are administered to γδ T cells isolated from patients with leukemia, during 14 days of culture, they assume different phenotypic states. Most of them may exhibit an effector memory phenotype (CD45RA- CD27-), followed by a central memory phenotype (CD45RA- CD27+) (174). In addition, positive regulation of CD56, CD80 and CD86 is also provided (174), suggesting that, in addition to exerting strong antileukemic activity, these cells may also act as APCs and improve the antitumor responses.
Vγ9Vδ2 cell expansion using IL-2 may not even promote satisfactory proliferative rates; however, it is clear that the synergism between IL-2 and IL-15 confers a substantial increase in an inflammatory profile (174, 181), as these cytokines promote a higher transcription factor T-bet expression (181), which in turn, is related to greater cytotoxicity. In addition, the advantage of γδ T cells expanded with IL-2 + IL-15 can be maintained under one of TME’s hallmarks in vivo, namely hypoxia (181). In fact, a striking feature of the leukemic microenvironment is the low partial pressure of oxygen that favors the tumor-associated immunosuppressive pathways, while at the same time promoting expansion of LCs (185). In this context, the persistence of γδ T cells in hypoxia further demonstrates their clinical importance.
Alternatively, the combination of ZOL, IL-2 + IL-18 also promotes the proliferation of effector cells (186, 187) since IL-18 is an important inducer of IFN-γ secretion (188). Given this, it has been reported that this cytokine indirectly induces the expansion of γδ T cells. Tsuda et al. (186) showed that Vγ9Vδ2 cells are efficiently expanded in response to ZOL, IL-2 + IL-18, but in a CD56bright CD11c+ NK-like cell dependent fashion (187). Many studies have reported that the involvement of NK-like cells in the proliferation of γδ T cells implies greater expansion efficiency when compared to methods using dendritic cells (DC) or monocytes (187, 189–191). These findings suggest an approach targeted at feeder cells that may be responsible for γδ T cell clonal proliferation in different methods in vitro and, perhaps, in vivo.
IL-18 can also directly support γδ T cell expansion, even in the absence of feeder cells (192). When Vγ9Vδ2 cells are treated only with ZOL, there is a delay in their in vitro expansion, as prolonged exposure subjects these cells to acute ZOL toxicity (193). However, when IL-18 combined with geranylgeranyl pyrophosphate (GGPP) is added, the proliferative capacity is restored by inhibiting the toxic effects of ZOL, which allows a substantial expansion of viable γδ T cells to occur. IL-18 + GGPP also were able to activate γδ T cells, exhibiting a central memory or effector memory phenotype and with higher IFN-γ production and CD56 expression (192).
In a subsequent study, treatment with ZOL + IL-2 and culture with Mo-DCs stimulated an activated phenotype in γδ T cells. In this context, immature Mo-DCs have been shown to have a particularly higher capacity to intensify γδ T cell cytotoxicity against LCs, whether in autologous or allogeneic condition (194). Furthermore, IL-15 producing DCs isolated from healthy patients and patients with leukemia (in remission) can potentiate γδ T cell cytotoxicity in vitro (182). These DCs induced NKp30, CD16, CD80 and CD86 expression in γδ T cells in an IL-15 dependent manner. This methodology was able to produce γδ T cells with higher expression of co-stimulatory molecules and low expression of inhibitory proteins. In addition, stimulation with DCs + IPP + allogeneic LCs led to high IFN-γ secretion and strong antitumor activity (182).
Deniger et al. (162) demonstrate a new strategy that involves the use of artificial APCs (aAPCs) derived from the K562 leukemic lineage. These feeder cells were modified to express molecules, such as CD19, CD64, CD86, 4-1BBL and IL-15, on their membrane surface. When γδ T cells are cultured with aAPCs + IL-2 + IL-21, there is a remarkably robust 4900 ± 1700-fold polyclonal expansion (162). Most of these cells expressed different γδ TCR domains. Resultant γδ T cells also were able to kill LCs via TCR, NKG2D and DNAM-1 (162).
In the same vein, Cho et al. (175) used CD80+, CD83L+ and 4-1BBL+ aAPCs. At low IL-2 concentrations, these co-stimulatory molecules promoted a remarkable Vγ9Vδ2 cell expansion that secreted higher levels of IFN-γ and TNF (175). Notwithstanding, there was no significant proliferation rate (106-fold increase) when compared to the hefty increase observed in the previous study (162). Triple co-stimulation with these molecules induced not only the high IFN-γ and TNF production, but also the positive regulation of a range of other molecules such as IL-2, IL-6, perforins, Gzm A and Fas ligand (FasL) (175). Most importantly, the expanded cells exhibited a terminal effector phenotype (CD27low CD45RAhigh), followed by an effector memory phenotype (175).
Unlike most of the investigations discussed above, other studies have focused on γδ T cells that express the Vδ1 TCR chain. Substantial evidence has demonstrated the ability of this subtype to kill LCs, as already reviewed. Unlike the Vγ9Vδ2 subtype, these cells do not show susceptibility to activation-induced cell death (AICD), which has been reported in several experimental trials (125, 195, 196). These cells can also exercise immune surveillance for long periods, favoring the longevity of cancer immunity (197–199). Several unique attributes have been discovered that particularly place Vδ1 cells as attractive targets in antileukemic therapies. So far, a few studies have emerged that have sought to translate the functional role of these lymphocytes and their applicability, as we will highlight below.
Siegers et al. (30) developed an in vitro expansion protocol that enabled the proliferation of γδ T cells isolated from PB after treatment with lectin-based compounds named Concanavalin-A (Con-A). Thus, it was possible to expand Vδ1 cells in a greater proportion than the Vγ9Vδ2 subtype when Con-A was combined with IL-2 + IL-4. The low Vγ9Vδ2 cell proportion was motivated by the period of exposure to Con-A, which induced AICD in these lymphocytes (30). Noteworthy, the resulting Vδ1 cells exerted an efficient cytotoxic activity against LCs through TCR, NKG2D, CD56 and FasL (30).
Subsequently, proof-of-concept studies were performed on leukemia xenograft models using a newly established cell generation protocol called Delta One T (DOT), which was designed by Almeida et al. (31). Specifically, this clinical-grade protocol consists of two steps. First, γδ T cells are isolated from PB of healthy donors or patients with leukemia using magnetic beads and are cultured in vitro for 14 days. During this time, these lymphocytes are expanded using a combination of molecules, such as IFN-γ, IL-1β, IL-4 + IL-21, in association with anti-CD3 antibodies (Abs). Then, the expanded cells are transferred to a new culture medium, where they are restimulated by anti-CD3 combined with IL-15 and IFN-γ for another 7 days (31). Overall, this is a 3-week protocol that involves γδ TCR and cytokine stimulation that can accomplish its goals efficiently.
When γδ T cells were submitted to the DOT protocol, expansion was obtained with rates greater than 1000-fold, thus allowing the viable and efficient proliferation of highly cytotoxic cells. It is noteworthy that, with this cell proportion rate, Vδ1 cells, which are generally less frequent in the blood (55, 56), expand from less than 0.5% of all circulating T cells to more than 70% (25, 31). Notably, Vδ1 cells with high expression of NKp30, NKp44, DNAM-1 and 2B4 are also provided, all well established as key-receptors in antileukemic responses (66, 74). These lymphocytes do not regulate inhibitory proteins on their membrane surface, even after 3 weeks of continuous stimulation. In addition, many cell adhesion molecules and chemokine receptors are positively regulated, while these lymphocytes can kill autologous and allogeneic LCs in vivo, and ignoring normal cells (31).
Finally, the same protocol was tested by Lorenzo et al. (200), in which γδ T cells from PB were reinforced using a range of stimulatory molecules (31). While the previous study sought to mobilize Vδ1 cells against a CLL xenograft model (31), the latter work applied the DOT protocol to an AML xenograft model (200). It is important to highlight that in both cases there was an efficient regression of tumors, and this increased mice survival (31, 200). In addition, γδ T cells avoided systemic metastasis of LCs (31). They exerted their antileukemic activity against AML blasts in a partially TCR-dependent manner, while they depended on the B7-H6 expression (200), which binds to NKp30 (67).
Blocking Immune Checkpoints in γδ T Cells and Leukemic Cells
Although they are potent, γδ T cell antitumor responses can be regulated by immune checkpoints (IC). Many inhibitory proteins, such as PD1, CTLA4, LAG3, BTLA, T cell immunoreceptor with Ig and ITIM domains (TIGIT) and T cell immunoglobulin and mucin domain-containing protein 3 (TIM3), are key mediators in inflammatory regression and cell suppression, in the context of the TME (93, 201, 202). Generally, these molecular interactions can act synergistically with the infiltration of suppressive cells that support tumor evasion through the establishment of a strongly tolerogenic environment (26, 76). However, recent advances in cancer immunotherapy using monoclonal Abs (mAbs) targeting ICs, the immune checkpoint inhibitors (ICI), have shown that combinatorial blocking of proteins, such as PD1 and PD-L1, can restore cellular functions and reestablish antitumor activity (203, 204).
The mechanisms of γδ T cell regulation mediated by ICs are diverse and poorly understood, but seemingly unified by the fact that these receptors functionally complement each other and ensure the adjustment of the immune response. PD1 and BTLA are the most potent ICs shown to suppress γδ T cell cytotoxicity in cancer (205, 206). Although CTLA4 expression has not been consistently assessed, it is known that this molecule is rarely expressed in activated γδ T cells (207, 208). Importantly, the expression of these ICs may vary between γδ T cell subtypes, where, for example, Vδ1 cells exhibit higher PD1 expression than their Vγ9Vδ2 counterpart (209).
Early after activation, when the γδ TCRs find their cognate ligands, γδ T cells begin to rapidly display many of these ICs on the cell surface (205, 207). Collectively, the expression of these proteins is low or stable, but temporary, and is sufficient to reduce cytokine production, proliferation and survival of γδ T cells (205, 206, 208, 210, 211). These changes can also be observed in leukemia, as γδ T cells increase the expression of PD1, CTLA4 and BTLA, while LCs strongly regulate the expression of their ligands, such as PD-L1, CD80 and/or CD86, and herpesvirus-entry mediator (HVEM), respectively (212). This represents an important barrier, as these molecules can prevent the efficient activation of γδ T cells and the associated antitumor response. Blocking the expression of these inhibitory receptors through the use of ICIs may be an interesting alternative to reverse the state of anergy and/or cell exhaustion.
The influence of ICIs on γδ T cells and their potential impact on the associated cytotoxic activity, in the context of the leukemic microenvironment, has not yet been characterized and is, therefore, an open question. Despite this, PD1 has been shown to negatively regulate Vγ9Vδ2 cell responses, while the addition of ZOL + anti–PD-L1 was able to bypass the inhibitory signals and promote γδ T cell reactivation against LCs in a TCR-dependent fashion (205). Therefore, this discovery allows us to suggest that γδ TCR-mediated activation is capable of overcoming the inhibitory effects of the PD1/PD-L1 pathway, since the application of ICIs plus ZOL, which is a strong Vγ9Vδ2 TCR stimulator, apparently synergizes the activation of γδ T cells and restores their tumor reactivity (205).
Notably, Hoeres et al. (213) demonstrated that although PD1 signaling can modulate the production of IFN-γ in leukemia-reactive γδ T cells, its additional blockage and stimulation with ZOL can increase the production of this cytokine. Although it did not show a significant effect on the destruction of LCs by γδ T cells, the action of anti-PD1 + ZOL in these lymphocytes was able to induce high IFN-γ secretion, which is a potent inflammatory and antitumor factor (213). As noted, cytokine secretion, such as IFN-γ, can be negatively regulated, and we can infer from this study that the application of ICIs potentially reverses this suppressive condition and is able to stimulate the triggering of an antitumor response.
In addition to PD1/PD-L1, other inhibitory proteins are highly regulated in LCs (i.e., CTLA4, BTLA, TIGIT, TIM3 and LAG3) and their effects on γδ T cells have not yet been fully investigated (26, 159, 214–216). However, previous studies have shown that some of these receptors have great potential for deregulating their antitumor activity, reflecting in cytokine production (213, 217). Nonetheless, evaluating these components before proceeding to a clinical application is important, since these molecules most likely prevent the efficient killing of LCs. This is one of several mechanisms of tumor escape that are commonly observed in recent and innovative treatment modalities, and which also include the chimeric antigen receptor (CAR) T cell therapy (218).
Focusing on γδ T Cell-Engager Molecules in the Leukemic Microenvironment
Antibodies Direct γδ T Cells Against LCs
As we have shown herein, data from in vitro experiments and mouse models unequivocally demonstrate the potential of γδ T cells against leukemia. Knowledge obtained regarding the many signals that regulate their activation and the tumor resistance underlying γδ T cells offers additional approaches that, in addition to inducing an activated status, a (poly)clonal expansion or a more pronounced Th1 phenotype, may also allow more specific targeting against the tumor. Improving γδ T cell efficiency against LCs, however, requires strategies based on their cytotoxic nature, which include, for example, antibody-dependent cell cytotoxicity (ADCC) (75, 219). Therefore, this implies a role for CD16, mAbs and bispecific antibodies (bsAbs) that bind to their respective target antigens.
CD16-mediated ADCC plays an important role in tumor destruction. For this to occur, CD16 must bind to the constant fraction of Abs IgG, thus constituting an optional axis in target cell killing. γδ T cells constitute the major blood T cell population that expresses CD16 (220, 221), although this expression is variable (222). Given this, the potential engagement of therapeutic Abs with the product of Vγ9Vδ2 cells can provide an efficient alternative against LCs (223). Several studies have shown that γδ T cells mediate leukemic regression via a CD16-dependent pathway (136, 223–226), in particular the Vγ9Vδ2 subtype, which positively regulates CD16 and TNF expression when stimulated with pAgs (227).
mAbs-coated LCs are efficiently destroyed by CD16+ γδ T cells via ADCC and these lymphocytes subsequently exhibit APC functions and activate αβ T cells, apparently through the tumor Ags presentation by MHC class II (228). It has been shown that the application of therapeutic CD20-targeting Abs, such as Rituximab (RTX), improves the antileukemic effect of these lymphocytes through tumor destruction by ADCC in vitro. This leads γδ T cells to secrete high levels of IFN-γ, perforins and CCL5 (219). In addition, BrHPP implementation potentiates the RTX bioactivity and consequently also increases γδ T cell cytotoxicity against CD20+ LCs in vitro and in vivo (75).
When peripheral blood mononuclear cells (PBMC) are stimulated with ZOL + IL-2 ex vivo and then cultured with LCs and Obinutuzumab (anti-CD20), it is observed that γδ T cells perform ADCC more efficiently than NK cells (223). Most importantly, the cytotoxicity of these lymphocytes cultured with Obinutuzumab is more potent compared to other tested mAbs, such as RTX. This view was reinforced when LCs treated with Obinutuzumab were substantially lysed in a CD16-dependent manner (223).
Benyamine et al. (136) demonstrated that BTN3A-targeting mAbs (anti-BTN3A 20.1) sensitize LCs and act indirectly in tumor destruction. This is due to the anti-BTN3A Abs binding in three different target molecules: BTN3A1, BTN3A2 and BTN3A3. The combination of these mAbs with γδ T cells and the subsequent infusion in a leukemia murine model was able to decrease the leukemic load in the PB and BM, increasing survival in these mice (136). Taken together, these data create the expectation that targeting mAbs to BTN proteins can be potentially useful in new therapeutic approaches.
Like most other surface molecules expressed in LCs, CD19 is also a potential target to be considered. When LCs are incubated with γδ T cells and modified anti-CD19 Abs (Ab 4G7SDIE), a significant increase in the degranulation marker CD107a is observed, as well as the strong IFN-γ and TNF production (224). In addition, the adoption of bsAbs targeting CD19/CD16 (bsAbs N19-C16) is also able to increase the expression of these inflammatory molecules (224). Interestingly, bsAbs targeting CD19/CD3 (bsAbs N19-CU) also strongly activated γδ T cells and, unlike the other previously tested Abs, mediated the lysis of LCs (224). It should be noted that the use of Abs modified to have a triple specificity to CD16 and CD19 (triplebody SPM-1) was also able to activate these lymphocytes against CD19+ target cells, which was evidenced by the expression of antitumor mediators (225).
The projection of a bsAbs targeting the Vγ9 TCR chain and CD123 (anti-TRGV9/CD123 engager) was also able to recruit γδ T cells against AML blasts (229). This engagement induced its activation and cytotoxicity against endogenous LCs, as evidenced by CD69, CD25 and Gzm B positive regulation. Interestingly, these activated γδ T cells exhibited a low secretion of IL-6 and IL-10, which are cytokines that are highly related to cytokine release syndrome (CRS) in patients undergoing αβ T cell-based therapies (229–231). The efficacy of this approach is evidenced when anti-Vγ9/CD123 directed γδ T cells were infused into a leukemia mouse model and controlled the leukemic proliferation in different compartments in these mice (229).
Finally, it has been shown that CD1d is also an attractive target. A recent study showed that CD1d specific single domain Abs can guide γδ T cells (226). These engagers were able to mobilize and activate these lymphocytes against autologous LCs from patients with CLL. This allowed γδ T cells to produce many inflammatory molecules and maintain their pAgs reactivity (226). Taken together, the many studies reviewed here allow us to suggest that the therapeutic application of Abs can be improved with the use of N-BPs that enhance γδ T cell activation. However, their therapeutic application against leukemia still needs more detailed investigation.
γδ T Cells Expressing CARs
While the application of therapeutic Abs has significantly increased the effectiveness of leukemia treatments, other approaches are also emerging with promising healing potential. Current advances in genetic engineering enable CAR transduction in NK cells, macrophages and T cells, thus offering new horizons for cell therapy, although this has been primarily focused on conventional αβ T cells (232, 233). In this context, γδ T cells are also undergoing a number of improvements in order to enhance their antitumor capacities.
The fact is that γδ T cells can be redirected with CARs against surface molecules expressed by LCs (234). Their unique innate properties and their high capacity for tumor sensing and killing place them in an interesting position in potential approaches against leukemia. CAR γδ T cells can offer a triple activity because, for example, they can recognize LCs (i) through the direct engagement of γδ TCR to their cognate ligand, (ii) through NKRs and their associated ligands, or (iii) through CAR specificity to the target antigen (Figure 3) (234, 235). Besides this, their APC functions (211) may allow the prolongation of immune response in the TME (228), since the CAR acquisition preserves the ability of γδ T cells to present tumor Ags (235).
The applicability of these genetically modified T cells has been established by some of the previous studies that evaluated the viability of viral transduction (236, 237) or electroporation (238) of the CAR. Rischer et al. (236) demonstrated for the first time that Vγ9Vδ2 cells can be efficiently transduced with CAR genes. Their study also showed that γδ T cells expressing anti-CD19 CARs destroy CD19+ LCs and produce high levels of IFN-γ in a target-dependent fashion (236). Subsequently, Deniger et al. (238) showed that the introduction of CAR by electroporation in PB-derived γδ T cells is able to produce polyclonal CAR T cells that express Vδ1, Vδ2 and Vδ3 TCR chains (238). For this to happen, approaches already reviewed here were used (162).
Noteworthy, one study demonstrated that CAR γδ T cells adopt a highly activated, but not exhausted, phenotype, as highlighted by the low regulation of CD57 (238). In addition, these lymphocytes tend to assume distinct phenotypic states of effector memory, while positively regulating homing molecules. Specifically, these homing receptors included CXCR4, a molecule associated with migration to BM, as well as CD62L and CCR7, which are linked to migration to lymph nodes (238). This is encouraging since BM and lymph nodes are sites of high tumor growth in acute and chronic leukemias (1, 4, 26, 78, 239–241).
Surprisingly, it has also been confirmed that CAR γδ T cells recognize and kill LCs in BM regardless of the CD19 target. Rozenbaum et al. (242) recently showed that these modified lymphocytes have high IFN-γ production and reactivity to CD19+/- LCs in vitro, which was even enhanced with the addition of ZOL. To investigate in vivo efficacy, the authors injected CAR γδ T cells in a leukemia mouse model. Although it did not induce a complete remission, the infusion of these cells led to a drastic reduction in the leukemic burden in the BM of these mice, which was even more pronounced when ZOL was administered (242).
These studies demonstrate that the production of CAR γδ T cells is viable and supports the high effectiveness of these lymphocytes against many malignancies, especially in leukemias. In contrast to conventional CAR T cell therapy, approaches based on γδ T cells can overcome several currently reported limitations, such as modulation of tumor antigen expression (242, 243) and CRS (229–231).
How About Molecular Switching of TCRs?
One interesting strategy for targeting lymphocytes against the tumor is to design γδ T cells with αβ TCRs or to design αβ T cells with γδ TCRs (244). This therapeutic concept has great potential for combining some unique γδ T cell properties, such as the rapid responsiveness to the tumor, the expression of individual molecules, and the absence of alloreactivity, with the high proliferative capacity and specific reactivity of conventional αβ T cells. Combining these unique aspects through TCR transduction leads us to expect that the resulting antileukemic responses will be long-lasting and based on immunological memory.
This new concept of modified T cells, named T cells engineered with defined γδ TCRs (TEG), was adopted in some studies that showed that TEGs kill LCs in vitro and in vivo models (245). TEGs tend to deregulate the intrinsic αβ TCR expression in their membrane surface, avoiding the graft-vs-host disease (GvHD) (245, 246). In addition, CD4+ TEGs retain their ability to induce a complete maturation of DCs, and stimulation with PAM can potentiate the cytotoxicity of CD8+ or CD4+ TEGs since it promotes higher production of inflammatory molecules, such as IFN-γ, TNF, and IL- 2, in vivo (245).
Similar results were obtained when TEGs cultured with LCs reduced the tumor in vitro (247). In addition, the infusion of TEGs plus IL-2 + PAM in an AML murine model enabled reactivity directed to LCs without affecting the healthy hematopoietic compartment and without being influenced by the TME, when inserted into mice that expressed IL-3, granulocyte-macrophage colony-stimulating factor (GM-CSF), and stem cell factor (SCF) (247), which are molecules that support tumor growth in vivo (248). Therefore, TEGs demonstrated efficiency in reducing the tumor in xenograft models with minimal alloreactivity, which stimulated the projection of a robust manufacturing procedure of TEGs that were validated under good manufacturing practice (GMP) conditions (244, 249).
Finally, γδ T cells transduced with αβ TCR plus CD4 and CD8 co-receptors showed high antitumor activity against LCs (250). As similarly observed in TEGs, transduction of αβ TCR induced a low expression of endogenous γδ TCR. In addition, modified CD8+ or CD4+ γδ T cells expressed high levels of IFN-γ and IL-4, although IFN-γ production was more pronounced in CD8+ cells. Most importantly, these transduced cells were able to kill LCs in vitro, although CD8+ γδ T cells have shown more efficiency than CD4+ cells (250, 251). This evidence supports the important role of γδ T cells in TCR gene transfer-based approaches while suggesting an improved antileukemic capacity when TCR transduction is combined with co-receptors, in particular, with the CD8 protein.
Converting γδ T Cells Into Living Drugs
Source, Isolation and Pre-Activation
γδ T cells and their subtypes are present in several tissues, but the ideal source for obtaining all these lymphocytes is still being determined. Despite this, therapeutic γδ T cells for infusion can be obtained from peripheral blood (252, 253) or umbilical cord blood (UCB) (254, 255). It is important to note that the frequency of γδ T cells varies between 5-10% of peripheral blood T cells (51, 52), while they constitute <1% of T cells in UCB (254). The functional differences between γδ T cell subtypes in these sources are not yet clear, but it is already established that while the subtype expressing Vγ9Vδ2 TCR predominates in PB (51, 55, 56), polyclonal γδ T cells expressing the Vδ1 TCR domain predominate in UCB (52, 256, 257).
γδ T cell expansion from PB is a well-established method and is usually adopted in clinical and experimental trials. For isolation of these lymphocytes, the starting material is the product of leukapheresis, which can be initially enriched through stimuli with several soluble factors (e.g., cytokines and N-BPs) and later undergoes removal of αβ T cells and CD19+ B cells through the use of magnetic beads, depletion or separation kits (optionally maintaining NK cells) (169, 252, 253). Since increasing the γδ T cell product from leukapheresis can further improve its therapeutic handling, adopting the use of molecules as the granulocyte colony-stimulating factor (G-CSF) may mobilize a large amount of antileukemic γδ T cells for peripheral blood, as shown in several studies (258–262).
Alternatively, physical exercise and the consequent systemic activation of β-adrenergic receptors (β-AR), immediately before PBMC isolation, has been shown to substantially increase mobilization for PB, ex vivo expansion and antitumor capacity. In their study, Baker et al. (263) showed that the practice of physical exercises can predict the expansion potential of γδ T cells, which is mobilized in a β-AR type 2 dependent fashion. Therefore, patients with high levels of physical activity mobilized γδ T cells that expanded ex vivo in much higher percentages compared to blood at rest when stimulated with IL-2 + ZOL for 14 days (263). These cells had higher expression of CD56 and NKG2D and showed high cytotoxicity against LCs in vitro.
On the other hand, γδ T cell isolation from UCB is still poorly investigated and so far, it has not been the target of cell expansion protocols in clinical trials. Berglund et al. (264) showed that it is possible to expand γδ T cells derived from UCB in vitro. The authors developed an expansion protocol based on the application of ZOL + IL-2 in culture for 14 days. This promotes the growth of Vγ9Vδ2 cells that mostly adopt a central memory phenotype and secrete higher levels of IL-1β, IL-2 and IL-8 (264). In general, the acquisition and handling of UCB-derived γδ T cells still need to be investigated more fully. Some factors, such as the low frequency of Vγ9Vδ2 cells (more easily expanded in vitro) in UCB and the poorly defined phenotypic diversity in this environment, make handling more limited (254). The approaches discussed here are viable targets for adoptive cell therapy because they also serve as adequate and economical adjuvants for hematopoietic stem cell transplantation (HSCT) (263, 264).
It is not clear whether pre-activation with ZOL + IL-2 can trigger the total antitumor capacity of γδ T cells. However, many in vitro approaches that use other molecules, such as IL-15, have demonstrated greater potential in stimulating the activation of these lymphocytes. As already reviewed, IL-15 associated with pAgs promotes high cytotoxicity in γδ T cells, which is evidenced by the high T-bet expression (181). In addition, the combined use of IL-2 + IL-15 can provide γδ T cells with antileukemic properties (174, 181, 182) even in hypoxia (181).
A mix of cytokines combined with Abs can also promote a pre-activated state in γδ T cells, as evidenced in studies using the DOT protocol. Notably, the use of IFN-γ, IL-1β, IL-4, IL-15, and IL-21 with anti-CD3 Abs positively regulates many NKRs, while ICs, such as PD1, CTLA4 and CD94/NK group 2 member A (NKG2A), are negatively regulated on the cell surface (31, 200). In addition, many homing receptors, such as signal-regulatory protein alpha (SIRPα), integrin-β7, CD31, CD56, CD96 and intercellular adhesion molecule 1 (ICAM-1), are expressed, as well as chemokine receptors, such as CXCR3, CCR6 and CX3C chemokine receptor 1 (CX3CR1) (31). Noteworthy, the junction of these cytokines promotes γδ T cells with APC functions and a higher potential to migrate and recirculate between blood and tissues (31, 174). Therefore, pre-activation using these approaches may lead to better crosstalk with other cytotoxic cells (e.g., NK) or LCs in different compartments (265).
The HSCT Questions
The functional importance of γδ T cells in HSCT has received enormous attention after many years of research. The fact is that the frequency of these lymphocytes may fluctuate between treated and untreated individuals, either during chemotherapy (27, 266) or after HSCT (267–273), implying relevant roles for γδ T cells in the patient’s recovery (274). Several initial reports have shown that αβ TCR depleted allogeneic HSTC (allo-HSCT) was able to increase disease-free survival (2-5 years) after transplantation (267, 268, 273). Notably, this was correlated with a high γδ T cell frequency circulating in the PB and mediating the graft-vs-leukemia (GvL) effect (267). The Vδ1 subtype represented the highest proportion of these cells in the blood of patients (267, 273, 275).
Given that γδ TCRs are not restricted to HLA expression, the triggering of the GvHD effect is less likely, since tumor detection depends on more ubiquitous targets (273, 276). Therefore, the high frequency of these cells contributes to the restoration of the hematopoietic niche and is related to antileukemic responses (273); although this is not their only contribution to the success of HSCT. Higher γδ T cell percentages and a lower incidence of infection was been observed in many patients after HSCT, indicating protective roles in fungal, bacterial and viral infections (268, 273, 276). This made it possible to increase survival in patients with a high frequency of these cells when compared to patients with low or normal counts (277).
Cytomegalovirus (CMV) infection and its reactivation is a major concern after HSCT and, notably, γδ T cells can be essential effectors in controlling viral expansion. Knight et al. (278) reported for the first time that Vδ1 and Vδ3 cells expand as a result of an active response against CMV in patients after allo-HSCT; although there were previous data that showed that these subtypes expand in CMV infection in immunocompetent individuals (275, 276, 279). Interestingly, CMV reactivation after allo-HSCT mobilized these non-Vδ2 subtypes against infected cells and against LCs in vivo (280). This is intriguing and leads us to infer that the reactivation of CMV after HSCT can benefit patients with leukemia, as it impacts the incidence of disease recurrence (281).
Epstein-Barr virus (EBV) infection is also a problem. Farnaut et al. (282) showed that EBV infection resulted in a significant Vδ1 cell expansion in a patient with ALL transplanted with UCB, which represented more than 80% of the total circulating γδ T cells. One year after transplantation, these cells were highly differentiated and exhibit CD57 and CD8 expression while minimally expressing the BTLA protein (282). These data suggest a strongly adaptive response from Vδ1 and Vδ3 cells that possibly improves the efficacy of allografts (269).
Overall, the graft enriched with γδ T cells provides a lower relapse incidence during immune reconstitution after HSCT (274). This is evidenced when patients with low frequencies of these lymphocytes have a high rate of death from relapse (283). In addition, γδ T cell innate and adaptive responses can also prevent the occurrence of infections after HSCT (269, 284, 285). Finally, their functional plasticity can assist in immunological tolerance to the graft and avoid GvHD, as evidenced in many studies (258, 260). Therefore, the data highlighted here position γδ T cells as potential targets in applications aimed at improving clinical results after HSCT, since they induce a potent GvL effect in the absence of GvHD.
The State-of-the-Art for Clinical Trials
Although promising, γδ T cells have not yet been fully translated into clinical research that targets leukemia. Although clinical studies carried out over two decades have shown that γδ T cells have low toxicity and reactivity against the host (274), the clinical efficacy of adoptive therapy with γδ T cells has not been consistently reported (Table 2). In vivo stimulation, that is, the activation of autologous γδ T cells using N-BPs + IL-2, induced few measurable responses in patients with leukemia. Wilhelm et al. (286) included 4 patients with CLL in a clinical study based on PAM + IL-2 in vivo infusion. None of the 4 patients were able to obtain objective or complete responses, which was also evidenced by the low expansion of endogenous γδ T cells in vitro when isolated from these patients.
Kunzmann et al. (287) evaluated stimulation with ZOL + IL-2 in several tumors. In this clinical trial, 8 patients with AML were included. Only 2 of them had an objective response, and they achieved a partial remission. Notably, ZOL infusion in pediatric patients with acute leukemia after HSCT depleted for αβ TCR and CD19+ B cells prolonged the disease-free survival in these patients, since it was associated with high numbers of circulating γδ T cells (271). This was also reported in a subsequent clinical trial that evaluated 46 pediatric patients with acute leukemia and reported that 3 or more repeated ZOL infusions offer a lower rate of transplant-related death, lower occurrence of relapses and absence of GvHD. Global disease-free survival is also improved (272).
The efficiency degree of donor γδ T cell ex vivo expansion is evidenced when the graft is depleted for αβ TCR, as this was able to induce a remarkable clinical recovery in 74 patients with acute and chronic leukemia, in which 43 achieved an objective response and 25 achieved complete remission, with no risk of recurrence and with improved survival after allo-HSCT (267). The subsequent follow-up of 153 patients with acute leukemia after allo-HSCT showed that γδ T cell-enriched graft, even inducing few complete remissions (36 patients), was able to confer a long-term survival advantage in patients who exhibited high γδ T cell frequency in the blood (268). Finally, ZOL + IL-2 in vivo stimulation after infusion of PBMC depleted for αβ T cells in 2 patients resulted in a higher in vivo expansion of donor γδ T cells and NK cells that induced complete remission in these patients (288).
It is important to highlight that many Phase I clinical trials are emerging to investigate γδ T cells as alternative axes in several established therapies since the available clinical and preclinical data suggest that γδ T cell-based strategies be combined with agents that better target these cells against the tumor. Therefore, several studies aiming at the optimization of γδ T cell antitumor reactivity through genetic engineering approaches are currently registered (Table 3). The use of these lymphocytes as platforms for CAR (NCT02656147) and TEG (NTR6541) engineering can overcome many obstacles observed in conventional adoptive therapy with αβ T cells and NK cells, although they also have their limitations (215, 228). Finally, in vivo stimulation and ex vivo expansion are also being insistently evaluated in the context of allo-HSCT (NCT02508038, NCT03862833) and the γδ T cell product infusion (NCT03885076, NCT04008381, NCT04028440, NCT03533816) in the expectation that a safe, effective and tolerable method for the treatment of patients will be discovered.
Concluding Remarks and Outlooks for the Future
Through this review, we hope to shed light on a relatively unexplored unconventional T cell. Nonetheless, it is one that has proven to be an important component in the leukemic microenvironment, since it responds effectively against the tumor and is able to affect the clinical outcome in patients with leukemia, as we recently reviewed (289). γδ T cells have unique immunological properties that allow the development of an off-the-shelf immunotherapy with universal applicability, that is, independent of histocompatibility related factors since γδ T cells respond regardless of MHC/HLA expression and recognize Ags presented by ubiquitous monomorphic molecules in many tumors in humans.
Furthermore, the clinical responses reported in clinical and pre-clinical trials, already reviewed here, highlight the importance of further increasing γδ T cell reactivity, either by raising intracellular pAg concentrations to “sensitize” LCs or by projecting γδ T cells with higher expression of receptors associated with cytotoxicity, adhesion and homing, as this allows recirculation and immune surveillance in different tumor compartments, even under hypoxia. The fact that these cells predominate in the blood and healthy or malignant tissues provides a migratory advantage over αβ T cells or NK cells and a greater ability to infiltrate and respond in the leukemic microenvironment; in particular the Vδ1 subtype, which has improved cytotoxicity and resistance to exhaustion or AICD.
The difficulty that still needs to be overcome for the therapeutic use of these cells is, in fact, is that of how to obtain a clinically significant cell proportion. As such, new techniques for cell expansion (or improvement) are necessary. In addition, ensuring that γδ T cell antileukemic phenotype is not diverted by TME stimuli also represents another challenge to be faced. Therefore, the modulation and effective targeting of these cells need to be achieved. Finally, improving and maintaining their in vivo persistence and invigorating exhausted γδ T cells also represent additional barriers that can be reversed using molecular factors that support their cytotoxicity in TME in vivo. The fact is that the innate and adaptive γδ T cell properties will lead to advances in better antileukemic approaches and potentially establish which of these will provide a real and applicable translational perspective.
Author Contributions
NDA and MSB established the initial conception, projected, and wrote this manuscript. NDA, MSB and TLPR collected, analyzed, and reviewed the data. MSB designed the illustrations and tables. NDA, FM-G, FSHA, AMT, AM and AGC supervised the project development, interpreted the data, and reviewed this manuscript. All authors contributed to the article and approved the submitted version.
Funding
This work was funded by Fundação de Amparo à Pesquisa do Estado do Amazonas (FAPEAM) (Pró-Estado Program - #002/2008, PAPAC Program - #005/2019 and and POSGRAD Program-#006/2020), Conselho Nacional de Desenvolvimento Científico e Tecnológico (CNPq), Coordenação de Aperfeiçoamento de Pessoal de Nível Superior (CAPES) (PROCAD-Amazônia 2018 Program-#88881.200581/2018-01) and the Brazilian Ministry of Health. MSB, TLPR, NDA, FM-G and FSHA have fellowships from FAPEAM, CAPES and CNPq (SI and PhD students). AM is a level 2 research fellow from CNPq. The funders had no role in study design and decision to publish, or preparation of the manuscript.
Conflict of Interest
The authors declare that the research was conducted in the absence of any commercial or financial relationships that could be construed as a potential conflict of interest.
Publisher’s Note
All claims expressed in this article are solely those of the authors and do not necessarily represent those of their affiliated organizations, or those of the publisher, the editors and the reviewers. Any product that may be evaluated in this article, or claim that may be made by its manufacturer, is not guaranteed or endorsed by the publisher.
Acknowledgments
We would like to thank all the authors, researchers at HEMOAM, UFAM and FMT-HVD for their critical discussions and insightful and encouraging ideas. We are also grateful for the support and thoughts that helped shape our intuition and the perspectives highlighted in this manuscript.
References
1. Höpken UE, Rehm A. Targeting the Tumor Microenvironment of Leukemia and Lymphoma. Trends Cancer (2019) 5:351–64. doi: 10.1016/j.trecan.2019.05.001
2. Batsivari A, Haltalli MLR, Passaro D, Pospori C, Lo Celso C, Bonnet D. Dynamic Responses of the Haematopoietic Stem Cell Niche to Diverse Stresses. Nat Cell Biol (2020) 22:7–17. doi: 10.1038/s41556-019-0444-9
3. Méndez-Ferrer S, Bonnet D, Steensma DP, Hasserjian RP, Ghobrial IM, Gribben JG, et al. Bone Marrow Niches in Haematological Malignancies. Nat Rev Cancer (2020) 20:285–98. doi: 10.1038/s41568-020-0245-2
4. Witkowski MT, Kousteni S, Aifantis I. Mapping and Targeting of the Leukemic Microenvironment. J Exp Med (2020) 217(1–13):e20190589. doi: 10.1084/jem.20190589
5. Carlsten M, Järås M. Natural Killer Cells in Myeloid Malignancies: Immune Surveillance, NK Cell Dysfunction, and Pharmacological Opportunities to Bolster the Endogenous NK Cells. Front Immunol (2019) 10:1–18. doi: 10.3389/fimmu.2019.02357
6. Sander FE, Rydström A, Bernson E, Kiffin R, Riise R, Aurelius J, et al. Dynamics of Cytotoxic T Cell Subsets During Immunotherapy Predicts Outcome in Acute Myeloid Leukemia. Oncotarget (2016) 7:7586–96. doi: 10.18632/oncotarget.7210
7. Wang M, Zhang C, Tian T, Zhang T, Wang R, Han F, et al. Increased Regulatory T Cells in Peripheral Blood of Acute Myeloid Leukemia Patients Rely on Tumor Necrosis Factor (TNF)-α-TNF Receptor-2 Pathway. Front Immunol (2018) 9:1274. doi: 10.3389/fimmu.2018.01274
8. Godfrey DI, Le Nours J, Andrews DM, Uldrich AP, Rossjohn J. Unconventional T Cell Targets for Cancer Immunotherapy. Immunity (2018) 48:453–73. doi: 10.1016/j.immuni.2018.03.009
9. Godfrey DI, Uldrich AP, Mccluskey J, Rossjohn J, Moody DB. The Burgeoning Family of Unconventional T Cells. Nat Immunol (2015) 16:1114–23. doi: 10.1038/ni.3298
10. Lepore M, Mori L, De Libero G. The Conventional Nature of Non-MHC-Restricted T Cells. Front Immunol (2018) 9:1365. doi: 10.3389/fimmu.2018.01365
11. Davey MS, Willcox CR, Baker AT, Hunter S, Willcox BE. Recasting Human Vδ1 Lymphocytes in an Adaptive Role. Trends Immunol (2018) 39:446–59. doi: 10.1016/j.it.2018.03.003
12. La Gruta NL, Gras S, Daley SR, Thomas PG, Rossjohn J. Understanding the Drivers of MHC Restriction of T Cell Receptors. Nat Rev Immunol (2018) 18:467–78. doi: 10.1038/s41577-018-0007-5
13. Melandri D, Zlatareva I, Chaleil RAG, Dart RJ, Chancellor A, Nussbaumer O, et al. The γδtcr Combines Innate Immunity With Adaptive Immunity by Utilizing Spatially Distinct Regions for Agonist Selection and Antigen Responsiveness. Nat Immunol (2018) 19:1352–65. doi: 10.1038/s41590-018-0253-5
14. Sebestyen Z, Prinz I, Déchanet-Merville J, Silva-Santos B, Kuball J. Translating Gammadelta (γδ) T Cells and Their Receptors Into Cancer Cell Therapies. Nat Rev Drug Discovery (2020) 19:169–84. doi: 10.1038/s41573-019-0038-z
15. Willcox CR, Davey MS, Willcox BE. Development and Selection of the Human Vγ9vδ2+ T-Cell Repertoire. Front Immunol (2018) 9:1501. doi: 10.3389/fimmu.2018.01501
16. Davey MS, Willcox CR, Hunter S, Kasatskaya SA, Remmerswaal EBM, Salim M, et al. The Human Vδ2+ T-Cell Compartment Comprises Distinct Innate-Like Vγ9+ and Adaptive Vγ9- Subsets. Nat Commun (2018) 9:1–14. doi: 10.1038/s41467-018-04076-0
17. Bosch F, Dalla-Favera R. Chronic Lymphocytic Leukaemia: From Genetics to Treatment. Nat Rev Clin Oncol (2019) 16:684–701. doi: 10.1038/s41571-019-0239-8
18. Richard-Carpentier G, Kantarjian H, Jabbour E. Recent Advances in Adult Acute Lymphoblastic Leukemia. Curr Hematol Malig Rep (2019) 14:106–18. doi: 10.1007/s11899-019-00503-1
19. Vetrie D, Helgason GV, Copland M. The Leukaemia Stem Cell: Similarities, Differences and Clinical Prospects in CML and AML. Nat Rev Cancer (2020) 20:158–73. doi: 10.1038/s41568-019-0230-9
20. Iacobucci I, Mullighan CG. Genetic Basis of Acute Lymphoblastic Leukemia. J Clin Oncol (2017) 35:975–83. doi: 10.1200/JCO.2016.70.7836
21. Greaves M. A Causal Mechanism for Childhood Acute Lymphoblastic Leukaemia. Nat Rev Cancer (2018) 18:471–84. doi: 10.1038/s41568-018-0015-6
22. Campo E, Swerdlow SH, Harris NL, Pileri S, Stein H, Jaffe ES. The 2008 WHO Classification of Lymphoid Neoplasms and Beyond: Evolving Concepts and Practical Applications. Blood (2011) 117:5019–32. doi: 10.1182/blood-2011-01-293050
23. Swerdlow SH, Campo E, Pileri SA, Lee Harris N, Stein H, Siebert R, et al. The 2016 Revision of the World Health Organization Classification of Lymphoid Neoplasms. Blood (2016) 127:2375–90. doi: 10.1182/blood-2016-01-643569
24. Zhao Y, Niu C, Cui J. Gamma-Delta (γδ) T Cells: Friend or Foe in Cancer Development. J Transl Med (2018) 16:1–13. doi: 10.1186/s12967-017-1378-2
25. Silva-Santos B, Mensurado S, Coffelt SB. γδ T Cells: Pleiotropic Immune Effectors With Therapeutic Potential in Cancer. Nat Rev Cancer (2019) 19:392–404. doi: 10.1038/s41568-019-0153-5
26. Curran EK, Godfrey J, Kline J. Mechanisms of Immune Tolerance in Leukemia and Lymphoma. Trends Immunol (2017) 38:513–25. doi: 10.1016/j.it.2017.04.004
27. Aswald JM, Wang XH, Aswald S, Lutynski A, Minden MD, Messner HA, et al. Flow Cytometric Assessment of Autologous γδ T Cells in Patients With Acute Myeloid Leukemia: Potential Effector Cells for Immunotherapy? Cytom Part B - Clin Cytom (2006) 70:379–90. doi: 10.1002/cyto.b.20115
28. Simões C, Silva I, Carvalho A, Silva S, Santos S, Marques G, et al. Quantification and Phenotypic Characterization of Peripheral Blood Vδ1 + T Cells in Chronic Lymphocytic Leukemia and Monoclonal B Cell Lymphocytosis. Cytom Part B - Clin Cytom (2019) 96:164–8. doi: 10.1002/cyto.b.21645
29. Poggi A, Venturino C, Catellani S, Clavio M, Miglino M, Gobbi M, et al. Vδ1 T Lymphocytes From B-CLL Patients Recognize ULBP3 Expressed on Leukemic B Cells and Up-Regulated by Trans-Retinoic Acid. Cancer Res (2004) 64:9172–9. doi: 10.1158/0008-5472.CAN-04-2417
30. Siegers GM, Dhamko H, Wang XH, Mathieson AM, Kosaka Y, Felizardo TC, et al. Human Vδ1 γδ T Cells Expanded From Peripheral Blood Exhibit Specific Cytotoxicity Against B-Cell Chronic Lymphocytic Leukemia-Derived Cells. Cytotherapy (2011) 13:753–64. doi: 10.3109/14653249.2011.553595
31. Almeida AR, Correia DV, Fernandes-Platzgummer A, Da Silva CL, Da Silva MG, Anjos DR, et al. Delta One T Cells for Immunotherapy of Chronic Lymphocytic Leukemia: Clinical-Grade Expansion/Differentiation and Preclinical Proof of Concept. Clin Cancer Res (2016) 22:5795–804. doi: 10.1158/1078-0432.CCR-16-0597
32. Muro R, Takayanagi H, Nitta T. T Cell Receptor Signaling for γδt Cell Development. Inflammation Regener (2019) 39:1–11. doi: 10.1186/s41232-019-0095-z
33. Pellicci DG, Koay HF, Berzins SP. Thymic Development of Unconventional T Cells: How NKT Cells, MAIT Cells and γδ T Cells Emerge. Nat Rev Immunol (2020) 20:756–70. doi: 10.1038/s41577-020-0345-y
34. Muñoz-Ruiz M, Sumaria N, Pennington DJ, Silva-Santos B. Thymic Determinants of γδ T Cell Differentiation. Trends Immunol (2017) 38:336–44. doi: 10.1016/j.it.2017.01.007
35. Saito H, Kranz DM, Takagaki Y, Hayday AC, Eisen HN, Tonegawa S, et al. and Expressed Gene in a Clone of Cytotoxic T Lymphocytes. Nature (1984) 312:36–40. doi: 10.1038/312036a0
36. Brenner MB, McLean J, Dialynas DP, Strominger JL, Smith JA, Owen FL, et al. Identification of a Putative Second T-Cell Receptor. Nature (1986) 322:145–9. doi: 10.1038/322145a0
37. Bank I, Depinho RA, Brenner MB, Cassimeris J, Alt FW, Chess L. A Functional T3 Molecule Associated With a Novel Heterodimer on the Surface of Immature Human Thymocytes. Nature (1986) 322:179–81. doi: 10.1038/322179a0
38. Borst J, Van De Griend RJ, Van Oostveen JW, Ang SL, Melief CJ, Seidman JG. Bolhuis RLH. A T-Cell Receptor γ/CD3 Complex Found on Cloned Functional Lymphocytes. Nature (1987) 325:683–8. doi: 10.1038/325683a0
39. Di Lorenzo B, Ravens S, Silva-Santos B. High-Throughput Analysis of the Human Thymic Vδ1+ T Cell Receptor Repertoire. Sci Data (2019) 6:115. doi: 10.1038/s41597-019-0118-2
40. Ribot JC, Lopes N, Silva-Santos B. γδ T Cells in Tissue Physiology and Surveillance. Nat Rev Immunol (2020) 21:1–12. doi: 10.1038/s41577-020-00452-4
41. Parker ME, Ciofani M. Regulation of γδ T Cell Effector Diversification in the Thymus. Front Immunol (2020) 11:42. doi: 10.3389/fimmu.2020.00042
42. LeFranc MP, Forster A, Baer R, Stinson MA, Rabbitts TH. Diversity and Rearrangement of the Human T Cell Rearranging γ Genes: Nine Germ-Line Variable Genes Belonging to Two Subgroups. Cell (1986) 45:237–46. doi: 10.1016/0092-8674(86)90388-0
43. Willcox CR, Pitard V, Netzer S, Couzi L, Salim M, Silberzahn T, et al. Cytomegalovirus and Tumor Stress Surveillance by Binding of a Human γδ T Cell Antigen Receptor to Endothelial Protein C Receptor. Nat Immunol (2012) 13:872–9. doi: 10.1038/ni.2394
44. Petrasca A, Melo AM, Breen EP, Doherty DG. Human Vδ3 + γδ T Cells Induce Maturation and IgM Secretion by B Cells. Immunol Lett (2018) 196:126–34. doi: 10.1016/j.imlet.2018.02.002
45. Vyborova A, Beringer DX, Fasci D, Karaiskaki F, van Diest E, Kramer L, et al. γ9δ2t Cell Diversity and the Receptor Interface With Tumor Cells. J Clin Invest (2020) 130:4637–51. doi: 10.1172/JCI132489
46. Kenna T, Golden-Mason L, Norris S, Hegarty JE, O’Farrelly C, Doherty DG. Distinct Subpopulations of γδ T Cells Are Present in Normal and Tumor-Bearing Human Liver. Clin Immunol (2004) 113:56–63. doi: 10.1016/j.clim.2004.05.003
47. Kashani E, Föhse L, Raha S, Sandrock I, Oberdörfer L, Koenecke C, et al. A Clonotypic Vγ4jγ1/Vδ5dδ2jδ1 Innate γδ T-Cell Population Restricted to the CCR6+CD27- Subset. Nat Commun (2015) 6:1–11. doi: 10.1038/ncomms7477
48. Alejenef A, Pachnio A, Halawi M, Christmas SE, Moss PAH, Khan N. Cytomegalovirus Drives Vδ2neg γδ T Cell Inflation in Many Healthy Virus Carriers With Increasing Age. Clin Exp Immunol (2014) 176:418–28. doi: 10.1111/cei.12297
49. Marlin R, Pappalardo A, Kaminski H, Willcox CR, Pitard V, Netzer S, et al. Sensing of Cell Stress by Human γδ TCR-Dependent Recognition of Annexin A2. Proc Natl Acad Sci USA (2017) 114:3163–8. doi: 10.1073/pnas.1621052114
50. Kabelitz D, Hinz T, Dobmeyer T, Mentzel U, Marx S, Böhme A, et al. Clonal Expansion of Vγ3/Vδ3-Expressing γδ T Cells in a HIV-1/2-Negative Patient With CD4 T-Cell Deficiency. Br J Haematol (1997) 96:266–71. doi: 10.1046/j.1365-2141.1997.d01-2027.x
51. Kozbor D, Trinchieri G, Monos DS, Isobe M, Russo G, Haney JA, et al. Human TCR-γ+/δ+, CD8+ T Lymphocytes Recognize Tetanus Toxoid in an MHC-Restricted Fashion. J Exp Med (1989) 169:1847–51. doi: 10.1084/jem.169.5.1847
52. Parker CM, Groh V, Band H, Porcelli SA, Morita C, Fabbi M, et al. Evidence for Extrathymic Changes in the T Cell Receptor γ/δ Repertoire. J Exp Med (1990) 171:1597–612. doi: 10.1084/jem.171.5.1597
53. Khairallah C, Chu TH, Sheridan BS. Tissue Adaptations of Memory and Tissue-Resident Gamma Delta T Cells. Front Immunol (2018) 9:2636. doi: 10.3389/fimmu.2018.02636
54. Mayassi T, Jabri B. Human Intraepithelial Lymphocytes. Mucosal Immunol (2018) 11:1281–9. doi: 10.1038/s41385-018-0016-5
55. Hinz T, Wesch D, Halary F, Marx S, Choudhary A, Arden B, et al. Identification of the Complete Expressed Human TCR V(γ) Repertoire by Flow Cytometry. Int Immunol (1997) 9:1065–72. doi: 10.1093/intimm/9.8.1065
56. Wesch D, Hinz T, Kabelitz D. Analysis of the TCR V(γ) Repertoire in Healthy Donors and HIV-1-Infected Individuals. Int Immunol (1998) 10:1067–75. doi: 10.1093/intimm/10.8.1067
57. Willcox BE, Mohammed F, Willcox CR. γδ TCR Recognition of MR1: Adapting to Life on the Flip Side. Trends Biochem Sci (2020) 45:551–3. doi: 10.1016/j.tibs.2020.03.012
58. Willcox CR, Mohammed F, Willcox BE. The Distinct MHC-Unrestricted Immunobiology of Innate-Like and Adaptive-Like Human γδ T Cell Subsets—Nature’s CAR-T Cells. Immunol Rev (2020) 298:25–46. doi: 10.1111/imr.12928
59. Papadopoulou M, Sanchez Sanchez G, Vermijlen D. Innate and Adaptive γδ T Cells: How, When, and Why. Immunol Rev (2020) 298:99–116. doi: 10.1111/imr.12926
60. Mangan BA, Dunne MR, O’Reilly VP, Dunne PJ, Exley MA, O’Shea D, et al. Cutting Edge: CD1d Restriction and Th1/Th2/Th17 Cytokine Secretion by Human Vδ3 T Cells. J Immunol (2013) 191:30–4. doi: 10.4049/jimmunol.1300121
61. Wang L, Xu M, Wang C, Zhu L, Hu J, Chen S, et al. The Feature of Distribution and Clonality of Tcr γ/δ Subfamilies T Cells in Patients With B-Cell Non-Hodgkin Lymphoma. J Immunol Res (2014) 2014:1–6. doi: 10.1155/2014/241246
62. Christopoulos P, Bukatz D, Kock S, Malkovsky M, Finke J, Fisch P. Improved Analysis of Tcrγδ Variable Region Expression in Humans. J Immunol Methods (2016) 434:66–72. doi: 10.1016/j.jim.2016.04.009
63. Zheng H, Wang X, Ma Y, Xu B, Chen S, Yang L, et al. The TCR γδ Repertoire and Relative Gene Expression Characteristics of T-ALL Cases With Biclonal Malignant Vδ1 and Vδ2 T Cells. DNA Cell Biol (2014) 33:49–56. doi: 10.1089/dna.2013.2199
64. Porcelli S, Brenner MB, Greenstein JL, Balk SP, Terhorst C, Bleicher PA. Recognition of Cluster of Differentiation 1 Antigens by Human CD4-CD8- Cytolytic T Lymphocyte. Nature (1989) 341:447–50. doi: 10.1038/341447a0
65. Le Nours J, Gherardin NA, Ramarathinam SH, Awad W, Wiede F, Gully BS, et al. A Class of Gd T Cell Receptors Recognize the Underside of the Antigen-Presenting Molecule MR1. Science (80-) (2019) 366:1522–7. doi: 10.1126/science.aav3900
66. Correia DV, Fogli M, Hudspeth K, Gomes Da Silva M, Mavilio D, Silva-Santos B. Differentiation of Human Peripheral Blood Vδ1+ T Cells Expressing the Natural Cytotoxicity Receptor NKp30 for Recognition of Lymphoid Leukemia Cells. Blood (2011) 118:992–1001. doi: 10.1182/blood-2011-02-339135
67. Barrow AD, Martin CJ, Colonna M. The Natural Cytotoxicity Receptors in Health and Disease. Front Immunol (2019) 10:909. doi: 10.3389/fimmu.2019.00909
68. Mikulak J, Oriolo F, Bruni E, Roberto A, Colombo FS, Villa A, et al. NKp46-Expressing Human Gut-Resident Intraepithelial Vδ1 T Cell Subpopulation Exhibits High Antitumor Activity Against Colorectal Cancer. JCI Insight (2019) 4:17. doi: 10.1172/jci.insight.125884
69. Lakshmikanth T, Burke S, Ali TH, Kimpfler S, Ursini F, Ruggeri L, et al. NCRs and DNAM-1 Mediate NK Cell Recognition and Lysis of Human and Mouse Melanoma Cell Lines In Vitro and In Vivo. J Clin Invest (2009) 119:1251–63. doi: 10.1172/JCI36022
70. Glasner A, Ghadially H, Gur C, Stanietsky N, Tsukerman P, Enk J, et al. Recognition and Prevention of Tumor Metastasis by the NK Receptor Nkp46/NCR1. J Immunol (2012) 188:2509–15. doi: 10.4049/jimmunol.1102461
71. Rincon-Orozco B, Kunzmann V, Wrobel P, Kabelitz D, Steinle A, Herrmann T. Activation of Vγ9vδ2 T Cells by NKG2D. J Immunol (2005) 175:2144–51. doi: 10.4049/jimmunol.175.4.2144
72. Rigau M, Ostrouska S, Fulford TS, Johnson DN, Woods K, Ruan Z, et al. Butyrophilin 2A1 Is Essential for Phosphoantigen Reactivity by Gd T Cells. Science (80-) (2020) 367(1–23):eaay5516. doi: 10.1126/science.aay5516
73. Karunakaran MM, Willcox CR, Salim M, Paletta D, Fichtner AS, Noll A, et al. Butyrophilin-2a1 Directly Binds Germline-Encoded Regions of the Vγ9vδ2 TCR and Is Essential for Phosphoantigen Sensing. Immunity (2020) 52:487–498.e6. doi: 10.1016/j.immuni.2020.02.014
74. Gertner-Dardenne J, Castellano R, Mamessier E, Garbit S, Kochbati E, Etienne A, et al. Human Vγ9vδ2 T Cells Specifically Recognize and Kill Acute Myeloid Leukemic Blasts. J Immunol (2012) 188:4701–8. doi: 10.4049/jimmunol.1103710
75. Gertner-Dardenne J, Bonnafous C, Bezombes C, Capietto AH, Scaglione V, Ingoure S, et al. Bromohydrin Pyrophosphate Enhances Antibody-Dependent Cell-Mediated Cytotoxicity Induced by Therapeutic Antibodies. Blood (2009) 113:4875–84. doi: 10.1182/blood-2008-08-172296
76. Chiarini F, Lonetti A, Evangelisti C, Buontempo F, Orsini E, Evangelisti C, et al. Advances in Understanding the Acute Lymphoblastic Leukemia Bone Marrow Microenvironment: From Biology to Therapeutic Targeting. Biochim Biophys Acta - Mol Cell Res (2016) 1863:449–63. doi: 10.1016/j.bbamcr.2015.08.015
77. Rovatti PE, Gambacorta V, Lorentino F, Ciceri F, Vago L. Mechanisms of Leukemia Immune Evasion and Their Role in Relapse After Haploidentical Hematopoietic Cell Transplantation. Front Immunol (2020) 11:147. doi: 10.3389/fimmu.2020.00147
78. Herndon TM, Chen SS, Saba NS, Valdez J, Emson C, Gatmaitan M, et al. Direct In Vivo Evidence for Increased Proliferation of CLL Cells in Lymph Nodes Compared to Bone Marrow and Peripheral Blood. Leukemia (2017) 31:1340–7. doi: 10.1038/leu.2017.11
79. Hanahan D, Weinberg RA. Hallmarks of Cancer: The Next Generation. Cell (2011) 144:646–74. doi: 10.1016/j.cell.2011.02.013
80. Zhao Y, Shao Q, Peng G. Exhaustion and Senescence: Two Crucial Dysfunctional States of T Cells in the Tumor Microenvironment. Cell Mol Immunol (2020) 17:27–35. doi: 10.1038/s41423-019-0344-8
81. Rotte A, Bhandaru M, Rotte A, Bhandaru M. Mechanisms of Immune Evasion by Cancer. In: Immunotherapy of Melanoma. Springer, Cham. (2016). doi: 10.1007/978-3-319-48066-4_8
82. Duarte D, Hawkins ED, Lo Celso C. The Interplay of Leukemia Cells and the Bone Marrow Microenvironment. Blood (2018) 131:1507–11. doi: 10.1182/blood-2017-12-784132
83. Zhang L, Gajewski TF, Kline J. PD-1/PD-L1 Interactions Inhibit Antitumor Immune Responses in a Murine Acute Myeloid Leukemia Model. Blood (2009) 114:1545–52. doi: 10.1182/blood-2009-03-206672
84. Yang H, Bueso-Ramos C, Dinardo C, Estecio MR, Davanlou M, Geng QR, et al. Expression of PD-L1, PD-L2, PD-1 and CTLA4 in Myelodysplastic Syndromes is Enhanced by Treatment With Hypomethylating Agents. Leukemia (2014) 28:1280–8. doi: 10.1038/leu.2013.355
85. Kornblau SM, McCue D, Singh N, Chen W, Estrov Z, Coombes KR. Recurrent Expression Signatures of Cytokines and Chemokines Are Present and Are Independently Prognostic in Acute Myelogenous Leukemia and Myelodysplasia. Blood (2010) 116:4251–61. doi: 10.1182/blood-2010-01-262071
86. Curti A, Aluigi M, Pandolfi S, Ferri E, Isidori A, Salvestrini V, et al. Acute Myeloid Leukemia Cells Constitutively Express the Immunoregulatory Enzyme Indoleamine 2,3-Dioxygenase [3]. Leukemia (2007) 21:353–5. doi: 10.1038/sj.leu.2404485
87. Corm S, Berthon C, Imbenotte M, Biggio V, Lhermitte M, Dupont C, et al. Indoleamine 2,3-Dioxygenase Activity of Acute Myeloid Leukemia Cells Can be Measured From Patients’ Sera by HPLC and is Inducible by IFN-γ. Leuk Res (2009) 33:490–4. doi: 10.1016/j.leukres.2008.06.014
88. Mussai F, De Santo C, Abu-Dayyeh I, Booth S, Quek L, McEwen-Smith RM, et al. Acute Myeloid Leukemia Creates an Arginase-Dependent Immunosuppressive Microenvironment. Blood (2013) 122:749–58. doi: 10.1182/blood-2013-01-480129
89. Fechter K, Dorronsoro A, Jakobsson E, Ferrin I, Lang V, Sepulveda P, et al. Ifnγ Regulates Activated Vδ2+ T Cells Through a Feedback Mechanism Mediated by Mesenchymal Stem Cells. PloS One (2017) 12:e0169362. doi: 10.1371/journal.pone.0169362
90. Pérez-García A, Brunet S, Berlanga JJ, Tormo M, Nomdedeu J, Guardia R, et al. CTLA-4 Genotype and Relapse Incidence in Patients With Acute Myeloid Leukemia in First Complete Remission After Induction Chemotherapy. Leukemia (2009) 23:486–91. doi: 10.1038/leu.2008.339
91. Zhong RK, Loken M, Lane TA, Ball ED. CTLA-4 Blockade by a Human MAb Enhances the Capacity of AML-Derived DC to Induce T-Cell Responses Against AML Cells in an Autologous Culture System. Cytotherapy (2006) 8:3–12. doi: 10.1080/14653240500499507
92. Zhou Q, Munger ME, Highfill SL, Tolar J, Weigel BJ, Riddle M, et al. Program Death-1 Signaling and Regulatory T Cells Collaborate to Resist the Function of Adoptively Transferred Cytotoxic T Lymphocytes in Advanced Acute Myeloid Leukemia. Blood (2010) 116:2484–93. doi: 10.1182/blood-2010-03-275446
93. Chen X, Liu S, Wang L, Zhang W, Ji Y, Ma X. Clinical Significance of B7-H1 (PD-L1) Expression in Human Acute Leukemia. Cancer Biol Ther (2008) 7:622–7. doi: 10.4161/cbt.7.5.5689
94. Krönig H, Kremmler L, Haller B, Englert C, Peschel C, Andreesen R, et al. Interferon-Induced Programmed Death-Ligand 1 (PD-L1/B7-H1) Expression Increases on Human Acute Myeloid Leukemia Blast Cells During Treatment. Eur J Haematol (2014) 92:195–203. doi: 10.1111/ejh.12228
95. Vago L, Perna SK, Zanussi M, Mazzi B, Barlassina C, Stanghellini MTL, et al. Loss of Mismatched HLA in Leukemia After Stem-Cell Transplantation. N Engl J Med (2009) 361:478–88. doi: 10.1056/nejmoa0811036
96. Stölzel F, Hackmann K, Kuithan F, Mohr B, Füssel M, Oelschlägel U, et al. Clonal Evolution Including Partial Loss of Human Leukocyte Antigen Genes Favoring Extramedullary Acute Myeloid Leukemia Relapse After Matched Related Allogeneic Hematopoietic Stem Cell Transplantation. Transplantation (2012) 93:744–9. doi: 10.1097/TP.0b013e3182481113
97. Masuda K, Hiraki A, Fujii N, Watanabe T, Tanaka M, Matsue K, et al. Loss or Down-Regulation of HLA Class I Expression at the Allelic Level in Freshly Isolated Leukemic Blasts. Cancer Sci (2007) 98:102–8. doi: 10.1111/j.1349-7006.2006.00356.x
98. Berrien-Elliott MM, Jackson SR, Meyer JM, Rouskey CJ, Nguyen TLM, Yagita H, et al. Durable Adoptive Immunotherapy for Leukemia Produced by Manipulation of Multiple Regulatory Pathways of CD8+ T-Cell Tolerance. Cancer Res (2013) 73:605–16. doi: 10.1158/0008-5472.CAN-12-2179
99. Moqattash S, Lutton JD. Leukemia Cells and the Cytokine Network. Proc Soc Exp Biol Med (1998) 219:8–27. doi: 10.3181/00379727-219-44311
100. Chen H, He W. Human Regulatory γδt Cells and Their Functional Plasticity in the Tumor Microenvironment. Cell Mol Immunol (2018) 15:411–3. doi: 10.1038/cmi.2017.73
101. Presti EL, Pizzolato G, Corsale AM, Caccamo N, Sireci G, Dieli F, et al. γδ T Cells and Tumor Microenvironment: From Immunosurveillance to Tumor Evasion. Front Immunol (2018) 9:1395. doi: 10.3389/fimmu.2018.01395
102. Lo Presti E, Di Mitri R, Pizzolato G, Mocciaro F, Dieli F, Meraviglia S. γδ Cells and Tumor Microenvironment: A Helpful or a Dangerous Liason? J Leukoc Biol (2018) 103:485–92. doi: 10.1002/JLB.5MR0717-275RR
103. Peters C, Häsler R, Wesch D, Kabelitz D. Human Vδ2 T Cells are a Major Source of Interleukin-9. Proc Natl Acad Sci USA (2016) 113:12520–5. doi: 10.1073/pnas.1607136113
104. Presti EL, Pizzolato G, Gulotta E, Cocorullo G, Gulotta G, Dieli F, et al. Current Advances in γδ T Cell-Based Tumor Immunotherapy. Front Immunol (2017) 8:1401. doi: 10.3389/fimmu.2017.01401
105. Angkasekwinai P, Dong C. IL-9-Producing T Cells: Potential Players in Allergy and Cancer. Nat Rev Immunol (2021) 21:37–48. doi: 10.1038/s41577-020-0396-0
106. Fleming C, Morrissey S, Cai Y, Yan J. γδ T Cells: Unexpected Regulators of Cancer Development and Progression. Trends Cancer (2017) 3:561–70. doi: 10.1016/j.trecan.2017.06.003
107. Raverdeau M, Cunningham SP, Harmon C, Lynch L. γδ T Cells in Cancer: A Small Population of Lymphocytes With Big Implications. Clin Transl Immunol (2019) 8(1–15):e1080. doi: 10.1002/cti2.1080
108. Li C, Donninger H, Eaton J, Yaddanapudi K. Regulatory Role of Immune Cell-Derived Extracellular Vesicles in Cancer: The Message Is in the Envelope. Front Immunol (2020) 11:1525. doi: 10.3389/fimmu.2020.01525
109. Wan J, Wu Y, Ji X, Huang L, Cai W, Su Z, et al. IL-9 and IL-9-Producing Cells in Tumor Immunity. Cell Commun Signal (2020) 18:50. doi: 10.1186/s12964-020-00538-5
110. Hayday AC, Vantourout P. The Innate Biologies of Adaptive Antigen Receptors. Annu Rev Immunol (2020) 38:487–510. doi: 10.1146/annurev-immunol-102819-023144
111. Lança T, Costa MF, Gonçalves-Sousa N, Rei M, Grosso AR, Penido C, et al. Protective Role of the Inflammatory CCR2/CCL2 Chemokine Pathway Through Recruitment of Type 1 Cytotoxic γδ T Lymphocytes to Tumor Beds. J Immunol (2013) 190:6673–80. doi: 10.4049/jimmunol.1300434
112. Ye J, Ma C, Wang F, Hsueh EC, Toth K, Huang Y, et al. Specific Recruitment of γδ Regulatory T Cells in Human Breast Cancer. Cancer Res (2013) 73:6137–48. doi: 10.1158/0008-5472.CAN-13-0348
113. Glatzel A, Wesch D, Schiemann F, Brandt E, Janssen O, Kabelitz D. Patterns of Chemokine Receptor Expression on Peripheral Blood γδ T Lymphocytes: Strong Expression of CCR5 Is a Selective Feature of Vδ2/Vγ9 γδ T Cells. J Immunol (2002) 168:4920–9. doi: 10.4049/jimmunol.168.10.4920
114. Zhou J, Kang N, Cui L, Ba D, He W. Anti-γδ TCR Antibody-Expanded γδ T Cells: A Better Choice for the Adoptive Immunotherapy of Lymphoid Malignancies. Cell Mol Immunol (2012) 9:34–44. doi: 10.1038/cmi.2011.16
115. Nicol AJ, Tokuyama H, Mattarollo SR, Hagi T, Suzuki K, Yokokawa K, et al. Clinical Evaluation of Autologous Gamma Delta T Cell-Based Immunotherapy for Metastatic Solid Tumours. Br J Cancer (2011) 105:778–86. doi: 10.1038/bjc.2011.293
116. Devaud C, Bilhere E, Loizon S, Pitard V, Behr C, Moreau JF, et al. Antitumor Activity of γδ T Cells Reactive Against Cytomegalovirus- Infected Cells in a Mouse Xenograft Tumor Model. Cancer Res (2009) 69:3971–8. doi: 10.1158/0008-5472.CAN-08-3037
117. Geherin SA, Wilson RP, Jennrich S, Debes GF. CXCR4 is Dispensable for T Cell Egress From Chronically Inflamed Skin via the Afferent Lymph. PloS One (2014) 9:95626. doi: 10.1371/journal.pone.0095626
118. Poggi A, Carosio R, Fenoglio D, Brenci S, Murdaca G, Setti M, et al. Migration of Vδ1 and Vδ2 T Cells in Response to CXCR3 and CXCR4 Ligands in Healthy Donors and HIV-1-Infected Patients: Competition by HIV-1 Tat. Blood (2004) 103:2205–13. doi: 10.1182/blood-2003-08-2928
119. Poggi A, Zancolli M, Catellani S, Borsellino G, Battistini L, Zocchi MR. Migratory Pathways of γδ T Cells and Response to CXCR3 and CXCR4 Ligands: Adhesion Molecules Involved and Implications for Multiple Sclerosis Pathogenesis. In: Annals of the New York Academy of Sciences. (2007) Blackwell Publishing Inc. p. 68–78. doi: 10.1196/annals.1381.008
120. Mingari MC, Varese P, Bottino C, Melioli G, Moretta A, Moretta L. Clonal Analysis of CD4 CD8- Human Thymocytes Expressing a T Cell Receptor γ/δ Chain. Direct Evidence for the De Novo Expression of CD8 Surface Antigen and of Cytolytic Activity Against Tumor Targets*. Eur J Immunol (1988) 18:1831–4. doi: 10.1002/eji.1830181127
121. Rivas A, Koide J, Cleary ML, Engleman EG. Evidence for Involvement of the γ,δ T Cell Antigen Receptor in Cytotoxicity Mediated by Human Alloantigen-Specific T Cell Clones J Immunol (1989) 142:1840–6.
122. Bensussan A, Lagabrielle JF, Castaigne S, Boisson N, Miclea JM, Benbunan M, et al. Human CD3 γδ + Activated Lymphocytes Exhibit Killer Activity In Vitro Against Autologous Leukemic Cells Nouv Rev Fr Hematol (1989) 31:129–32.
123. Duval M, Yotnda P, Bensussan A, Oudhiri N, Guidal C, Rohrlich P, et al. Potential Antileukemic Effect of γδ T Cells in Acute Lymphoblastic Leukemia. Leukemia (1995) 9:863–8.
124. Tosolini M, Pont F, Poupot M, Vergez F, Nicolau-Travers ML, Vermijlen D, et al. Assessment of Tumor-Infiltrating Tcrvγ9vδ2 γδ Lymphocyte Abundance by Deconvolution of Human Cancers Microarrays. Oncoimmunology (2017) 6(1–10):e1284723. doi: 10.1080/2162402X.2017.1284723
125. Coscia M, Vitale C, Peola S, Foglietta M, Rigoni M, Griggio V, et al. Dysfunctional Vγ9vδ2 T Cells are Negative Prognosticators and Markers of Dysregulated Mevalonate Pathway Activity in Chronic Lymphocytic Leukemia Cells. Blood (2012) 120:3271–9. doi: 10.1182/blood-2012-03-417519
126. Hilpert J, Zeiser M, Kanz L, Baessler T, Steinle A, Salih HR. NKG2D and Its Ligands In Leukemia: Comprehensive Analysis of Expression, Release and Modulation of NK Cell Reactivity. Blood (2010) 116:1686–6. doi: 10.1182/blood.v116.21.1686.1686
127. Salih HR, Antropius H, Gieseke F, Lutz SZ, Kanz L, Rammensee HG, et al. Functional Expression and Release of Ligands for the Activating Immunoreceptor NKG2D in Leukemia. Blood (2003) 102:1389–96. doi: 10.1182/blood-2003-01-0019
128. Paczulla AM, Rothfelder K, Raffel S, Konantz M, Steinbacher J, Wang H, et al. Absence of NKG2D Ligands Defines Human Acute Myeloid Leukaemia Stem Cells and Mediates Their Immune Evasion. Blood (2018) 132:769–9. doi: 10.1182/blood-2018-99-118047
129. Paczulla AM, Rothfelder K, Raffel S, Konantz M, Steinbacher J, Wang H, et al. Absence of NKG2D Ligands Defines Leukaemia Stem Cells and Mediates Their Immune Evasion. Nature (2019) 572:254–9. doi: 10.1038/s41586-019-1410-1
130. Lança T, Correia DV, Moita CF, Raquel H, Neves-Costa A, Ferreira C, et al. The MHC Class Ib Protein ULBP1 is a Nonredundant Determinant of Leukemia/Lymphoma Susceptibility to γδ T-Cell Cytotoxicity. Blood (2010) 115:2407–11. doi: 10.1182/blood-2009-08-237123
131. Gomes AQ, Correia DV, Grosso AR, Lança T, Ferreira C, Lacerda JF, et al. Identification of a Panel of Ten Cell Surface Protein Antigens Associated With Immunotargeting of Leukemias and Lymphomas by Peripheral Blood γδ T Cells. Haematologica (2010) 95:1397–404. doi: 10.3324/haematol.2009.020602
132. Gundermann S, Klinker E, Kimmel B, Flierl U, Wilhelm M, Einsele H, et al. A Comprehensive Analysis of Primary Acute Myeloid Leukemia Identifies Biomarkers Predicting Susceptibility to Human Allogeneic Vγ9vδ2 T Cells. J Immunother (2014) 37:321–30. doi: 10.1097/CJI.0000000000000043
133. Peipp M, Wesch D, Oberg HH, Lutz S, Muskulus A, Van De Winkel JGJ, et al. CD20-Specific Immunoligands Engaging NKG2D Enhance γδ T Cell-Mediated Lysis of Lymphoma Cells. Scand J Immunol (2017) 86:196–206. doi: 10.1111/sji.12581
134. Zöller T, Wittenbrink M, Hoffmeister M, Steinle A. Cutting an NKG2D Ligand Short: Cellular Processing of the Peculiar Human NKG2D Ligand Ulbp4. Front Immunol (2018) 9:620. doi: 10.3389/fimmu.2018.00620
135. Han B, Zhao Y, Lin Y, Fu S, Limengmeng W, Mingming Z, et al. Hydroxychloroquine Sensitizes Chronic Myeloid Leukemia Cells to Vγ9vδ2 T Cell-Mediated Lysis Independent of Autophagy. Int J Oncol (2017) 50:1810–20. doi: 10.3892/ijo.2017.3934
136. Benyamine A, Le Roy A, Mamessier E, Gertner-Dardenne J, Castanier C, Orlanducci F, et al. BTN3A Molecules Considerably Improve Vγ9vδ2T Cells-Based Immunotherapy in Acute Myeloid Leukemia. Oncoimmunology (2016) 5(1–10):e1146843. doi: 10.1080/2162402X.2016.1146843
137. Sandstrom A, Peigné CM, Léger A, Crooks JE, Konczak F, Gesnel MC, et al. The Intracellular B30.2 Domain of Butyrophilin 3A1 Binds Phosphoantigens to Mediate Activation of Human Vγ9vδ2t Cells. Immunity (2014) 40:490–500. doi: 10.1016/j.immuni.2014.03.003
138. Rhodes DA, Chen H-C, Price AJ, Keeble AH, Davey MS, James LC, et al. Activation of Human γδ T Cells by Cytosolic Interactions of BTN3A1 With Soluble Phosphoantigens and the Cytoskeletal Adaptor Periplakin. J Immunol (2015) 194:2390–8. doi: 10.4049/jimmunol.1401064
139. Sebestyen Z, Scheper W, Vyborova A, Gu S, Rychnavska Z, Schiffler M, et al. RhoB Mediates Phosphoantigen Recognition by Vγ9vδ2 T Cell Receptor. Cell Rep (2016) 15:1973–85. doi: 10.1016/j.celrep.2016.04.081
140. Agea E, Russano A, Bistoni O, Mannucci R, Nicoletti I, Corazzi L, et al. Human CD1-Restricted T Cell Recognition of Lipids From Pollens. J Exp Med (2005) 202:295–308. doi: 10.1084/jem.20050773
141. Russano AM, Bassotti G, Agea E, Bistoni O, Mazzocchi A, Morelli A, et al. CD1-Restricted Recognition of Exogenous and Self-Lipid Antigens by Duodenal γδ + T Lymphocytes. J Immunol (2007) 178:3620–6. doi: 10.4049/jimmunol.178.6.3620
142. Luoma AM, Castro CD, Mayassi T, Bembinster LA, Bai L, Picard D, et al. Crystal Structure of Vδ1t Cell Receptor in Complex With CD1d-Sulfatide Shows MHC-Like Recognition of a Self-Lipid by Human γδ T Cells. Immunity (2013) 39:1032–42. doi: 10.1016/j.immuni.2013.11.001
143. Bai L, Picard D, Anderson B, Chaudhary V, Luoma A, Jabri B, et al. The Majority of CD1d-Sulfatide-Specific T Cells in Human Blood Use a Semiinvariant Vδ1 TCR. Eur J Immunol (2012) 42:2505–10. doi: 10.1002/eji.201242531
144. Lepore M, de Lalla C, Gundimeda R, Gsellinger H, Consonni M, Garavaglia C, et al. A Novel Self-Lipid Antigen Targets Human T Cells Against CD1c+ Leukemias. J Exp Med (2014) 211:1363–77. doi: 10.1084/jem.20140410
145. Luoma AM, Castro CD, Adams EJ. γδ T Cell Surveillance via CD1 molecules. Trends Immunol (2014) 35:613–21. doi: 10.1016/j.it.2014.09.003
146. Reijneveld JF, Ocampo TA, Shahine A, Gully BS, Vantourout P, Hayday AC, et al. Human γδ T Cells Recognize CD1b by Two Distinct Mechanisms. Proc Natl Acad Sci USA (2020) 117:22944–52. doi: 10.1073/pnas.2010545117
147. Spada FM, Grant EP, Peters PJ, Sugita M, Melián A, Leslie DS, et al. Self-Recognition of CD1 by γ/δ T Cells: Implications for Innate Immunity. J Exp Med (2000) 191:937–48. doi: 10.1084/jem.191.6.937
148. Uldrich AP, Le Nours J, Pellicci DG, Gherardin NA, Mcpherson KG, Lim RT, et al. CD1d-Lipid Antigen Recognition by the γδ TCR. Nat Immunol (2013) 14:1137–45. doi: 10.1038/ni.2713
149. Bojarska-Junak A, Hus I, Chocholska S, Tomczak W, Woś J, Czubak P, et al. CD1d Expression is Higher in Chronic Lymphocytic Leukemia Patients With Unfavorable Prognosis. Leuk Res (2014) 38:435–42. doi: 10.1016/j.leukres.2013.12.015
150. Anastasiadis A, Kotsianidis I, Papadopoulos V, Spanoudakis E, Margaritis D, Christoforidou A, et al. CD1d Expression as a Prognostic Marker for Chronic Lymphocytic Leukemia. Leuk Lymphoma (2014) 55:320–5. doi: 10.3109/10428194.2013.803222
151. Kotsianidis I, Nakou E, Spanoudakis E, Bouchliou I, Moustakidis E, Miltiades P, et al. The Diagnostic Value of CD1d Expression in a Large Cohort of Patients With B-Cell Chronic Lymphoproliferative Disorders. Am J Clin Pathol (2011) 136:400–8. doi: 10.1309/AJCP2F2DOXOTXHZA
152. Guo W, Dong A, Xing C, Lin X, Pan X, Lin Y, et al. CD1d Levels in Peripheral Blood of Patients With Acute Myeloid Leukemia and Acute Lymphoblastic Leukemia. Oncol Lett (2014) 8:825–30. doi: 10.3892/ol.2014.2208
153. Bartkowiak J, Kulczycka-Wojdala D, Blonski JZ, Robak T. Molecular Diversity of γδ T Cells in Peripheral Blood From Patients With B-Cell Chronic Lymphocytic Leukaemia. Neoplasma (2002) 49:86–90.
154. Kjer-Nielsen L, Patel O, Corbett AJ, Le Nours J, Meehan B, Liu L, et al. MR1 Presents Microbial Vitamin B Metabolites to MAIT Cells. Nature (2012) 491:717–23. doi: 10.1038/nature11605
155. Corbett AJ, Eckle SBG, Birkinshaw RW, Liu L, Patel O, Mahony J, et al. T-Cell Activation by Transitory Neo-Antigens Derived From Distinct Microbial Pathways. Nature (2014) 509:361–5. doi: 10.1038/nature13160
156. Crowther MD, Dolton G, Legut M, Caillaud ME, Lloyd A, Attaf M, et al. Genome-Wide CRISPR–Cas9 Screening Reveals Ubiquitous T Cell Cancer Targeting via the Monomorphic MHC Class I-Related Protein MR1. Nat Immunol (2020) 21:178–85. doi: 10.1038/s41590-019-0578-8
157. Lepore M, Kalinichenko A, Calogero S, Kumar P, Paleja B, Schmaler M, et al. Functionally Diverse Human T Cells Recognize non-Microbial Antigens Presented by MR1. Elife (2017) 6(1–22):e24476. doi: 10.7554/eLife.24476
158. Bottino C, Castriconi R, Pende D, Rivera P, Nanni M, Carnemolla B, et al. Identification of PVR (CD155) and Nectin-2 (CD112) as Cell Surface Ligands for the Human DNAM-1 (CD226) Activating Molecule. J Exp Med (2003) 198:557–67. doi: 10.1084/jem.20030788
159. Jin Z, Ye W, Lan T, Zhao Y, Liu X, Chen J, et al. Characteristic of TIGIT and DNAM-1 Expression on Foxp3+ γδ T Cells in AML Patients. BioMed Res Int (2020) 2020:1–10. doi: 10.1155/2020/4612952
160. Yazdanifar M, Barbarito G, Bertaina A, Airoldi I. γδ T Cells: The Ideal Tool for Cancer Immunotherapy. Cells (2020) 9(1–26):1305. doi: 10.3390/cells9051305
161. Li Y, Li G, Zhang J, Wu X, Chen X. The Dual Roles of Human γδ T Cells: Anti-Tumor or Tumor-Promoting. Front Immunol (2021) 11:619954. doi: 10.3389/fimmu.2020.619954
162. Deniger DC, Maiti SN, Mi T, Switzer KC, Ramachandran V, Hurton LV, et al. Activating and Propagating Polyclonal Gamma Delta T Cells With Broad Specificity for Malignancies. Clin Cancer Res (2014) 20:5708–19. doi: 10.1158/1078-0432.CCR-13-3451
163. Xiao L, Chen C, Li Z, Zhu S, Tay JC, Zhang X, et al. Large-Scale Expansion of Vγ9vδ2 T Cells With Engineered K562 Feeder Cells in G-Rex Vessels and Their Use as Chimeric Antigen Receptor–Modified Effector Cells. Cytotherapy (2018) 20:420–35. doi: 10.1016/j.jcyt.2017.12.014
164. Idrees ASM, Sugie T, Inoue C, Murata-Hirai K, Okamura H, Morita CT, et al. Comparison of γδ T Cell Responses and Farnesyl Diphosphate Synthase Inhibition in Tumor Cells Pretreated With Zoledronic Acid. Cancer Sci (2013) 104:536–42. doi: 10.1111/cas.12124
165. Gober HJ, Kistowska M, Angman L, Jenö P, Mori L, De Libero G. Human T Cell Receptor γδ Cells Recognize Endogenous Mevalonate Metabolites in Tumor Cells. J Exp Med (2003) 197:163–8. doi: 10.1084/jem.20021500
166. Roelofs AJ, Jauhiainen M, Mönkkönen H, Rogers MJ, Mönkkönen J, Thompson K. Peripheral Blood Monocytes are Responsible for γδ T Cell Activation Induced by Zoledronic Acid Through Accumulation of IPP/DMAPP. Br J Haematol (2009) 144:245–50. doi: 10.1111/j.1365-2141.2008.07435.x
167. Soriano-Sarabia N, Sandvold H, Jomaa H, Kubin T, Bein G, Hackstein H. Primary MHC-Class II + Cells Are Necessary To Promote Resting Vδ2 Cell Expansion in Response to (E)-4-Hydroxy-3-Methyl-But-2-Enyl-Pyrophosphate and Isopentenyl Pyrophosphate. J Immunol (2012) 189:5212–22. doi: 10.4049/jimmunol.1200093
168. Green AE, Lissina A, Hutchinson SL, Hewitt RE, Temple B, James D, et al. Recognition of Nonpeptide Antigens by Human Vγ9vδ2 T Cells Requires Contact With Cells of Human Origin. Clin Exp Immunol (2004) 136:472–82. doi: 10.1111/j.1365-2249.2004.02472.x
169. Wang S. Expansion of Gamma Delta T Cells - A Short Review on Bisphosphonate and K562-Based Methods. J Immunol Sci (2018) 2:6–12. doi: 10.29245/2578-3009/2018/3.1133
170. Espinosa E, Belmant C, Pont F, Luciani B, Poupot R, Romagné F, et al. Chemical Synthesis and Biological Activity of Bromohydrin Pyrophosphate, a Potent Stimulator of Human γδ T Cells. J Biol Chem (2001) 276:18337–44. doi: 10.1074/jbc.M100495200
171. Watanabe N, Narita M, Yokoyama A, Sekiguchi A, Saito A, Tochiki N, et al. Type I IFN-Mediated Enhancement of Anti-Leukemic Cytotoxicity of γδ T Cells Expanded From Peripheral Blood Cells by Stimulation With Zoledronate. Cytotherapy (2006) 8:118–29. doi: 10.1080/14653240600620200
172. Saito A, Narita M, Watanabe N, Tochiki N, Satoh N, Yano T, et al. Anti-Tumor Cytotoxicity of γδ T Cells Expanded From Blood Cells of Myeloma and Leukemia Patients Against Self Tumor Cells — Enhancement of the Anti-Tumor Cytotoxicity by Type I IFN, Dendritic Cells, and Activated αβ T Cells. Blood (2007) 110:4762–2. doi: 10.1182/blood.v110.11.4762.4762
173. Saito A, Narita M, Watanabe N, Yokoyama A, Sekiguchi A, Tochiki N, et al. Anti-Tumor Cytotoxicity of Blood γδ T Cells Expanded From Leukemia Patients Against Autologous Leukemia Cells—Enhancement of the Anti-Tumor Cytotoxicity by Type I IFN. Blood (2005) 106:2385–5. doi: 10.1182/blood.v106.11.2385.2385
174. Van Acker HH, Anguille S, Willemen Y, Van Den Bergh JM, Berneman ZN, Lion E, et al. Interleukin-15 Enhances the Proliferation, Stimulatory Phenotype, and Antitumor Effector Functions of Human Gamma Delta T Cells. J Hematol Oncol (2016) 9:1–13. doi: 10.1186/s13045-016-0329-3
175. Cho H-WH-I, Kim S-Y, Sohn D-H, Lee M-J, Park M-Y, Sohn H-J, et al. Triple Costimulation via CD80, 4-1BB, and CD83 Ligand Elicits the Long-Term Growth of Vγ9vδ2 T Cells in Low Levels of IL-2. J Leukoc Biol (2016) 99:521–9. doi: 10.1189/jlb.1hi0814-409rr
176. Kunzmann V, Bauer E, Feurle J, Weißinger F, Tony HP, Wilhelm M. Stimulation of γδ T Cells by Aminobisphosphonates and Induction of Antiplasma Cell Activity in Multiple Myeloma. Blood (2000) 96:384–92. doi: 10.1182/blood.v96.2.384.013k07_384_392
177. Herrmann T, Fichtner AS, Karunakaran MM. An Update on the Molecular Basis of Phosphoantigen Recognition by Vγ9vδ2 T Cells. Cells (2020) 9:1433. doi: 10.3390/cells9061433
178. D’Asaro M, La Mendola C, Di Liberto D, Orlando V, Todaro M, Spina M, et al. Vγ9vδ2 T Lymphocytes Efficiently Recognize and Kill Zoledronate-Sensitized, Imatinib-Sensitive, and Imatinib-Resistant Chronic Myelogenous Leukemia Cells. J Immunol (2010) 184:3260–8. doi: 10.4049/jimmunol.0903454
179. de Weerdt I, Terpstra S, Hofland T, Lameris R, de Bruin RCG, Levin M-D, et al. Chronic Lymphocytic Leukemia (CLL) Cells Are Susceptible to γδ-T Cell Mediated Killing, Provided CLL-Derived γδ-T Cell Dysfunction Can be Reversed. Blood (2015) 126:2914–4. doi: 10.1182/blood.v126.23.2914.2914
180. De Weerdt I, Hofland T, Lameris R, Endstra S, Jongejan A, Moerland PD, et al. Improving CLL Vγ9vδ2-T-Cell Fitness for Cellular Therapy by Ex Vivo Activation and Ibrutinib. Blood (2018) 132:2260–72. doi: 10.1182/blood-2017-12-822569
181. Aehnlich P, Carnaz Simões AM, Skadborg SK, Holmen Olofsson G, thor Straten P. Expansion With IL-15 Increases Cytotoxicity of Vγ9vδ2 T Cells and Is Associated With Higher Levels of Cytotoxic Molecules and T-Bet. Front Immunol (2020) 11:1868. doi: 10.3389/fimmu.2020.01868
182. Van Acker HH, Anguille S, De Reu H, Berneman ZN, Smits EL, Van Tendeloo VF. Interleukin-15-Cultured Dendritic Cells Enhance Anti-Tumor Gamma Delta T Cell Functions Through IL-15 Secretion. Front Immunol (2018) 9:658. doi: 10.3389/fimmu.2018.00658
183. Ribot JC, Ribeiro ST, Correia DV, Sousa AE, Silva-Santos B. Human γδ Thymocytes Are Functionally Immature and Differentiate Into Cytotoxic Type 1 Effector T Cells Upon IL-2/IL-15 Signaling. J Immunol (2014) 192:2237–43. doi: 10.4049/jimmunol.1303119
184. García VE, Jullien D, Song M, Uyemura K, Shuai K, Morita CT, et al. IL-15 Enhances the Response of Human γδ T Cells to Nonpetide Microbial Antigens(1998). Available at: http://www.jimmunol.org/content/160/9/http://www.jimmunol.org/content/160/9/4322.full#ref-list-1 (Accessed January 17, 2021).
185. Deynoux M, Sunter N, Hérault O, Mazurier F. Hypoxia and Hypoxia-Inducible Factors in Leukemias. Front Oncol (2016) 6:41. doi: 10.3389/fonc.2016.00041
186. Tsuda J, Li W, Yamanishi H, Yamamoto H, Okuda A, Kubo S, et al. Involvement of CD56 Bright CD11c + Cells in IL-18–Mediated Expansion of Human γδ T Cells. J Immunol (2011) 186:2003–12. doi: 10.4049/jimmunol.1001919
187. Li W, Okuda A, Yamamoto H, Yamanishi K, Terada N, Yamanishi H, et al. Regulation of Development of CD56brightCD11c+ NK-Like Cells With Helper Function by IL-18. PloS One (2013) 8:e82586. doi: 10.1371/journal.pone.0082586
188. Okamura H, Tsutsul H, Komatsu T, Yutsudo M, Tanimoto T, Torigoe K, et al. Cloning of a New Cytokine That Induces IFN-γ Production by T Cells. Nature (1995) 378:88–91. doi: 10.1038/378088a0
189. Li W, Kubo S, Okuda A, Yamamoto H, Ueda H, Tanaka T, et al. Effect of IL-18 on Expansion of γδ T Cells Stimulated by Zoledronate and IL-2. J Immunother (2010) 33:287–96. doi: 10.1097/CJI.0b013e3181c80ffa
190. Sugie T, Murata-Hirai K, Iwasaki M, Morita CT, Li W, Okamura H, et al. Zoledronic Acid-Induced Expansion of γδ T Cells From Early-Stage Breast Cancer Patients: Effect of IL-18 on Helper NK Cells. Cancer Immunol Immunother (2013) 62:677–87. doi: 10.1007/s00262-012-1368-4
191. Ramírez-Ramírez D, Vadillo E, Arriaga-Pizano LA, Mayani H, Estrada-Parra S, Velasco-Velázquez MA, et al. Early Differentiation of Human CD11c+NK Cells With γδ T Cell Activation Properties Is Promoted by Dialyzable Leukocyte Extracts. J Immunol Res (2016) 2016:1–12. doi: 10.1155/2016/4097642
192. Nussbaumer O, Gruenbacher G, Gander H, Komuczki J, Rahm A, Thurnher M. Essential Requirements of Zoledronate-Induced Cytokine and γδ T Cell Proliferative Responses. J Immunol (2013) 191:1346–55. doi: 10.4049/jimmunol.1300603
193. Wang H, Sarikonda G, Puan K-J, Tanaka Y, Feng J, Giner J-L, et al. Indirect Stimulation of Human Vγ2vδ2 T Cells Through Alterations in Isoprenoid Metabolism. J Immunol (2011) 187:5099–113. doi: 10.4049/jimmunol.1002697
194. Saito A, Narita M, Yokoyama A, Watanabe N, Tochiki N, Satoh N, et al. Enhancement of Anti-Tumor Cytotoxicity of Expanded Gammadelta T Cells by Stimulation With Monocyte-Derived Dendritic Cells. J Clin Exp Hematop (2007) 47:61–72. doi: 10.3960/jslrt.47.61
195. Sicard H, Ingoure S, Luciani B, Serraz C, Fournié J-J, Bonneville M, et al. In Vivo Immunomanipulation of Vγ9vδ2 T Cells With a Synthetic Phosphoantigen in a Preclinical Nonhuman Primate Model. J Immunol (2005) 175:5471–80. doi: 10.4049/jimmunol.175.8.5471
196. Ferrarini M, Delfanti F, Gianolini M, Rizzi C, Alfano M, Lazzarin A, et al. NF-κb Modulates Sensitivity to Apoptosis, Proinflammatory and Migratory Potential in Short- Versus Long-Term Cultured Human γδ Lymphocytes. J Immunol (2008) 181:5857–64. doi: 10.4049/jimmunol.181.9.5857
197. Wu D, Wu P, Wu X, Ye J, Wang Z, Zhao S, et al. Ex Vivo Expanded Human Circulating Vδ1 γδt Cells Exhibit Favorable Therapeutic Potential for Colon Cancer. Oncoimmunology (2015) 4:1–13. doi: 10.4161/2162402X.2014.992749
198. Knight A, MacKinnon S, Lowdell MW. Human Vdelta1 Gamma-Delta T Cells Exert Potent Specific Cytotoxicity Against Primary Multiple Myeloma Cells. Cytotherapy (2012) 14:1110–8. doi: 10.3109/14653249.2012.700766
199. Siegers GM, Lamb LS. Cytotoxic and Regulatory Properties of Circulating Vδ1+ γδ T Cells: A New Player on the Cell Therapy Field? Mol Ther (2014) 22:1416–22. doi: 10.1038/mt.2014.104
200. Di Lorenzo B, Simões AE, Caiado F, Tieppo P, Correia DV, Carvalho T, et al. Broad Cytotoxic Targeting of Acute Myeloid Leukemia by Polyclonal Delta One T Cells. Cancer Immunol Res (2019) 7:552–8:e4304 (1–7). doi: 10.1158/2326-6066.CIR-18-0647
201. Chen C, Liang C, Wang S, Chio CL, Zhang Y, Zeng C, et al. Expression Patterns of Immune Checkpoints in Acute Myeloid Leukemia. J Hematol Oncol (2020) 13:28. doi: 10.1186/s13045-020-00853-x
202. He X, Xu C. Immune Checkpoint Signaling and Cancer Immunotherapy. Cell Res (2020) 30:660–9. doi: 10.1038/s41422-020-0343-4
203. Liao D, Wang M, Liao Y, Li J. Niu T. A Review of Efficacy and Safety of Checkpoint Inhibitor for the Treatment of Acute Myeloid Leukemia. Front Pharmacol (2019) 10:609. doi: 10.3389/fphar.2019.00609
204. Miao YR, Zhang Q, Lei Q, Luo M, Xie GY, Wang H, et al. ImmuCellAI: A Unique Method for Comprehensive T-Cell Subsets Abundance Prediction and Its Application in Cancer Immunotherapy. Adv Sci (2020) 7:1902880. doi: 10.1002/advs.201902880
205. Iwasaki M, Tanaka Y, Kobayashi H, Murata-Hirai K, Miyabe H, Sugie T, et al. Expression and Function of PD-1 in Human γδ T Cells That Recognize Phosphoantigens. Eur J Immunol (2011) 41:345–55. doi: 10.1002/eji.201040959
206. Gertner-Dardenne J, Fauriat C, Orlanducci F, Thibult ML, Pastor S, Fitzgibbon J, et al. The Co-Receptor BTLA Negatively Regulates Human Vg9Vd2 T-Cell Proliferation: A Potential Way of Immune Escape for Lymphoma Cells. Blood (2013) 122:922–31. doi: 10.1182/blood-2012-11-464685
207. Peters C, Oberg HH, Kabelitz D, Wesch D. Phenotype and Regulation of Immunosuppressive Vδ2-Expressing γδ T Cells. Cell Mol Life Sci (2014) 71:1943–60. doi: 10.1007/s00018-013-1467-1
208. Ribeiro ST, Ribot JC, Silva-Santos B. Five Layers of Receptor Signaling in γδ T-Cell Differentiation and Activation. Front Immunol (2015) 6:15. doi: 10.3389/fimmu.2015.00015
209. Paquin-Proulx D, Barsotti NS, Santos BAN, Marinho AKBB, Kokron CM, Carvalho KI, et al. Inversion of the Vδ1 to Vδ2 γδ T Cell Ratio in CVID Is Not Restored by IVIg and Is Associated With Immune Activation and Exhaustion. Med (United States) (2016) 95(1-7):e4304. doi: 10.1097/MD.0000000000004304
210. Zumwalde NA, Sharma A, Xu X, Ma S, Schneider CL, Romero-Masters JC, et al. Adoptively Transferred Vγ9vδ2 T Cells Show Potent Antitumor Effects in a Preclinical B Cell Lymphomagenesis Model. JCI Insight (2017) 2(1–15):e93179. doi: 10.1172/jci.insight.93179
211. Bekiaris V, Šedý JR, Macauley MG, Rhode-Kurnow A, Ware CF. The Inhibitory Receptor BTLA Controls γδ T Cell Homeostasis and Inflammatory Responses. Immunity (2013) 39:1082–94. doi: 10.1016/j.immuni.2013.10.017
212. Kang SH, Hwang HJ, Yoo JW, Kim H, Choi ES, Hwang SH, et al. Expression of Immune Checkpoint Receptors on T-Cells and Their Ligands on Leukemia Blasts in Childhood Acute Leukemia. Anticancer Res (2019) 39:5531–9. doi: 10.21873/anticanres.13746
213. Hoeres T, Holzmann E, Smetak M, Birkmann J, Wilhelm M. PD-1 Signaling Modulates Interferon-γ Production by Gamma Delta (γδ) T-Cells in Response to Leukemia. Oncoimmunology (2019) 8(1–11):e1550618. doi: 10.1080/2162402X.2018.1550618
214. Chen C, Liang C, Wang S, Chio CL, Zhang Y, Zeng C, et al. Expression Patterns of Immune Checkpoints in Acute Myeloid Leukemia. J Hematol Oncol (2020) 13:28. doi: 10.1186/s13045-020-00853-x
215. Karabon L, Partyka A, Ciszak L, Pawlak-Adamska E, Tomkiewicz A, Bojarska-Junak A, et al. Abnormal Expression of BTLA and CTLA-4 Immune Checkpoint Molecules in Chronic Lymphocytic Leukemia Patients. J Immunol Res (2020) 2020:1–12. doi: 10.1155/2020/6545921
216. Jin Z, Lan T, Zhao Y, Du J, Chen J, Lai J, et al. Higher TIGIT+CD226- γδ T Cells in Patients With Acute Myeloid Leukemia. Immunol Invest (2020) 2020:1–11. doi: 10.1080/08820139.2020.1806868
217. Wu K, Feng J, Xiu Y, Li Z, Lin Z, Zhao H, et al. Vδ2 T Cell Subsets, Defined by PD-1 and TIM-3 Expression, Present Varied Cytokine Responses in Acute Myeloid Leukemia Patients. Int Immunopharmacol (2020) 80:106122. doi: 10.1016/j.intimp.2019.106122
218. Hosseinkhani N, Derakhshani A, Kooshkaki O, Shadbad MA, Hajiasgharzadeh K, Baghbanzadeh A, et al. Immune Checkpoints and Car-T Cells: The Pioneers in Future Cancer Therapies? Int J Mol Sci (2020) 21:1–28. doi: 10.3390/ijms21218305
219. Tokuyama H, Hagi T, Mattarollo SR, Morley J, Wang Q, Fai-So H, et al. Vγ9vδ2 T Cell Cytotoxicity Against Tumor Cells Is Enhanced by Monoclonal Antibody Drugs - Rituximab and Trastuzumab. Int J Cancer (2008) 122:2526–34. doi: 10.1002/ijc.23365
220. Lanier LL, Kipps TJ, Phillips JH. Functional Properties of a Unique Subset of Cytotoxic CD3+ T Lymphocytes That Express Fc Receptors for IgG (CD16/LEU-11 Antigen). J Exp Med (1985) 162:2089–106. doi: 10.1084/jem.162.6.2089
221. Braakman E, van de Winkel JGJ, van Krimpen BA, Jansze M, Bolhuis RLH. CD16 on Human γδ T Lymphocytes: Expression, Function, and Specificity for Mouse IgG Isotypes. Cell Immunol (1992) 143:97–107. doi: 10.1016/0008-8749(92)90008-D
222. Fonseca S, Pereira V, Lau C, Teixeira M dos A, Bini-Antunes M, Lima M. Human Peripheral Blood Gamma Delta T Cells: Report on a Series of Healthy Caucasian Portuguese Adults and Comprehensive Review of the Literature. Cells (2020) 9:729. doi: 10.3390/cells9030729
223. Hoeres T, Pretscher D, Holzmann E, Smetak M, Birkmann J, Triebel J, et al. Improving Immunotherapy Against B-Cell Malignancies Using γδ T-Cell-Specific Stimulation and Therapeutic Monoclonal Antibodies. J Immunother (2019) 42:331–44. doi: 10.1097/CJI.0000000000000289
224. Seidel UJE, Vogt F, Grosse-Hovest L, Jung G, Handgretinger R, Lang P. γδ T Cell-Mediated Antibody-Dependent Cellular Cytotoxicity With CD19 Antibodies Assessed by an Impedance-Based Label-Free Real-Time Cytotoxicity Assay. Front Immunol (2014) 5:618. doi: 10.3389/fimmu.2014.00618
225. Schiller CB, Braciak TA, Fenn NC, Seidel UJE, Roskopf CC, Wildenhain S, et al. CD19-Specific Triplebody SPM-1 Engages NK and Gd T Cells for Rapid and Efficient Lysis of Malignant B-Lymphoid Cells. Oncotarget (2016) 7:83392–408. doi: 10.18632/oncotarget.13110
226. de Weerdt I, Lameris R, Ruben JM, de Boer R, Kloosterman J, King LA, et al. Clin Cancer Res (2021) 27(6). doi: 10.1158/1078-0432.ccr-20-4576
227. Lafont V, Liautard J, Liautard JP, Favero J. Production of TNF-α by Human Vγ9vδ2 T Cells Via Engagement of Fcγriiia, the Low Affinity Type 3 Receptor for the Fc Portion of IgG, Expressed Upon TCR Activation by Nonpeptidic Antigen. J Immunol (2001) 166:7190–9. doi: 10.4049/jimmunol.166.12.7190
228. Himoudi N, Morgenstern DA, Yan M, Vernay B, Saraiva L, Wu Y, et al. Human γδ T Lymphocytes Are Licensed for Professional Antigen Presentation by Interaction With Opsonized Target Cells. J Immunol (2012) 188:1708–16. doi: 10.4049/jimmunol.1102654
229. Ganesan R, Chennupati V, Ramachandran B, Hansen MR, Singh S, Grewal IS. Selective Recruitment of γδ T Cells by a Bispecific Antibody for the Treatment of Acute Myeloid Leukemia. Leukemia (2021) 35:1–11. doi: 10.1038/s41375-021-01122-7
230. Harrer DC, Simon B, Fujii S, Shimizu K, Uslu U, Schuler G, et al. RNA-Transfection of γ/δ T Cells With a Chimeric Antigen Receptor or an α/β T-Cell Receptor: A Safer Alternative to Genetically Engineered α/β T Cells for the Immunotherapy of Melanoma. BMC Cancer (2017) 17:1–17. doi: 10.1186/s12885-017-3539-3
231. Xu Y, Yang Z, Horan LH, Zhang P, Liu L, Zimdahl B, et al. A Novel Antibody-TCR (AbTCR) Platform Combines Fab-Based Antigen Recognition With Gamma/Delta-TCR Signaling to Facilitate T-Cell Cytotoxicity With Low Cytokine Release. Cell Discovery (2018) 4:62. doi: 10.1038/s41421-018-0066-6
232. Cortés-Selva D, Dasgupta B, Singh S, Grewal IS. Innate and Innate-Like Cells: The Future of Chimeric Antigen Receptor (CAR) Cell Therapy. Trends Pharmacol Sci (2021) 42:45–59. doi: 10.1016/j.tips.2020.11.004
233. Fleischer LC, Spencer HT, Raikar SS. Targeting T Cell Malignancies Using CAR-Based Immunotherapy: Challenges and Potential Solutions. J Hematol Oncol (2019) 12:1–21. doi: 10.1186/s13045-019-0801-y
234. Fisher J, Anderson J. Engineering Approaches in Human Gamma Delta T Cells for Cancer Immunotherapy. Front Immunol (2018) 9:1409. doi: 10.3389/fimmu.2018.01409
235. Capsomidis A, Benthall G, Van Acker HH, Fisher J, Kramer AM, Abeln Z, et al. Chimeric Antigen Receptor-Engineered Human Gamma Delta T Cells: Enhanced Cytotoxicity With Retention of Cross Presentation. Mol Ther (2018) 26:354–65. doi: 10.1016/j.ymthe.2017.12.001
236. Rischer M, Pscherer S, Duwe S, Vormoor J, Jürgens H, Rossig C. Human γδ T Cells as Mediators of Chimaeric-Receptor Redirected Anti-Tumour Immunity. Br J Haematol (2004) 126:583–92. doi: 10.1111/j.1365-2141.2004.05077.x
237. Wang RN, Wen Q, He WT, Yang JH, Zhou CY, Xiong WJ, et al. Optimized Protocols for γδ T Cell Expansion and Lentiviral Transduction. Mol Med Rep (2019) 19:1471–80. doi: 10.3892/mmr.2019.9831
238. Deniger DC, Switzer K, Mi T, Maiti S, Hurton L, Singh H, et al. Bispecific T-Cells Expressing Polyclonal Repertoire of Endogenous γδ T-Cell Receptors and Introduced CD19-Specific Chimeric Antigen Receptor. Mol Ther (2013) 21:638–47. doi: 10.1038/mt.2012.267
239. Lee JY, Kim HJ. Lymph Angiogenic Influences on Hematopoietic Cells in Acute Myeloid Leukemia. Exp Mol Med (2014) 46:e122–2. doi: 10.1038/emm.2014.72
240. Verma D, Zanetti C, Godavarthy PS, Kumar R, Minciacchi VR, Pfeiffer J, et al. Bone Marrow Niche-Derived Extracellular Matrix-Degrading Enzymes Influence the Progression of B-Cell Acute Lymphoblastic Leukemia. Leukemia (2020) 34:1540–52. doi: 10.1038/s41375-019-0674-7
241. Baryawno N, Przybylski D, Kowalczyk MS, Kfoury Y, Severe N, Gustafsson K, et al. A Cellular Taxonomy of the Bone Marrow Stroma in Homeostasis and Leukemia. Cell (2019) 177:1915–1932.e16. doi: 10.1016/j.cell.2019.04.040
242. Rozenbaum M, Meir A, Aharony Y, Itzhaki O, Schachter J, Bank I, et al. Gamma-Delta CAR-T Cells Show CAR-Directed and Independent Activity Against Leukemia. Front Immunol (2020) 11:1347. doi: 10.3389/fimmu.2020.01347
243. Greenbaum U, Mahadeo KM, Kebriaei P, Shpall EJ, Saini NY. Chimeric Antigen Receptor T-Cells in B-Acute Lymphoblastic Leukemia: State of the Art and Future Directions. Front Oncol (2020) 10:1594. doi: 10.3389/fonc.2020.01594
244. Straetemans T, Kierkels GJJ, Doorn R, Jansen K, Heijhuurs S, dos Santos JM, et al. GMP-Grade Manufacturing of T Cells Engineered to Express a Defined γδtcr. Front Immunol (2018) 9:1062. doi: 10.3389/fimmu.2018.01062
245. Marcu-Malina V, Heijhuurs S, Van Buuren M, Hartkamp L, Strand S, Sebestyen Z, et al. Redirecting αβt Cells Against Cancer Cells by Transfer of a Broadly Tumor-Reactive γδt-Cell Receptor. Blood (2011) 118:50–9. doi: 10.1182/blood-2010-12-325993
246. Gründer C, Van Dorp S, Hol S, Drent E, Straetemans T, Heijhuurs S, et al. γ9 and δ2cdr3 Domains Regulate Functional Avidity of T Cells Harboring γ9δ2tcrs. Blood (2012) 120:5153–62. doi: 10.1182/blood-2012-05-432427
247. Johanna I, Straetemans T, Heijhuurs S, Aarts-Riemens T, Norell H, Bongiovanni L, et al. Evaluating In Vivo Efficacy - Toxicity Profile of TEG001 in Humanized Mice Xenografts Against Primary Human AML Disease and Healthy Hematopoietic Cells. J Immunother Cancer (2019) 7:69. doi: 10.1186/s40425-019-0558-4
248. Wunderlich M, Chou FS, Link KA, Mizukawa B, Perry RL, Carroll M, et al. AML Xenograft Efficiency Is Significantly Improved in NOD/SCID-IL2RG Mice Constitutively Expressing Human SCF, GM-CSF and IL-3. Leukemia (2010) 24:1785–8. doi: 10.1038/leu.2010.158
249. Straetemans T, Gründer C, Heijhuurs S, Hol S, Slaper-Cortenbach I, Bönig H, et al. Untouched GMP-Ready Purified Engineered Immune Cells to Treat Cancer. Clin Cancer Res (2015) 21:3957–68. doi: 10.1158/1078-0432.CCR-14-2860
250. Van Der Veken LT, Hagedoorn RS, Van Loenen MM, Willemze R, Falkenburg JHF, Heemskerk MHM. αβ T-Cell Receptor Engineered γδ T Cells Mediate Effective Antileukemic Reactivity. Cancer Res (2006) 66:3331–7. doi: 10.1158/0008-5472.CAN-05-4190
251. Hiasa A, Nishikawa H, Hirayama M, Kitano S, Okamoto S, Chono H, et al. Rapid αβ TCR-Mediated Responses in γδ T Cells Transduced With Cancer-Specific TCR Genes. Gene Ther (2009) 16:620–8. doi: 10.1038/gt.2009.6
252. Kondo M, Izumi T, Fujieda N, Kondo A, Morishita T, Matsushita H, et al. Expansion of Human Peripheral Blood γδ T Cells Using Zoledronate. J Vis Exp (2011) 55:e3182. doi: 10.3791/3182
253. Kondo M, Sakuta K, Noguchi A, Ariyoshi N, Sato K, Sato S, et al. Zoledronate Facilitates Large-Scale Ex Vivo Expansion of Functional γδ T Cells From Cancer Patients for Use in Adoptive Immunotherapy. Cytotherapy (2008) 10:842–56. doi: 10.1080/14653240802419328
254. Placido R, Auricchio G, Gabriele I, Galli E, Brunetti E, Colizzi V, et al. Characterization of the Immune Response of Human Cord-Blood Derived γδ T Cells to Stimulation With Aminobisphosphonate Compounds. Int J Immunopathol Pharmacol (2011) 24:101–10. doi: 10.1177/039463201102400112
255. Cairo C, Sagnia B, Cappelli G, Colizzi V, Leke RGF, Leke RJ, et al. Human Cord Blood γδ T Cells Expressing Public Vγ2 Chains Dominate the Response to Bisphosphonate Plus Interleukin-15. Immunology (2013) 138:346–60. doi: 10.1111/imm.12039
256. Kalyan S, Kabelitz D. Defining the Nature of Human γδ T Cells: A Biographical Sketch of the Highly Empathetic. Cell Mol Immunol (2013) 10:21–9. doi: 10.1038/cmi.2012.44
257. Dimova T, Brouwer M, Gosselin F, Tassignon J, Leo O, Donner C, et al. Effector Vγ9vδ2 T Cells Dominate the Human Fetal γδ T-Cell Repertoire. Proc Natl Acad Sci USA (2015) 112:E556–65. doi: 10.1073/pnas.1412058112
258. Xuan L, Wu X, Zhang Y, Fan Z, Ling Y, Huang F, et al. Granulocyte Colony-Stimulating Factor Affects the Distribution and Clonality of TRGV and TRDV Repertoire of T Cells and Graft-Versus-Host Disease. J Transl Med (2011) 9:215. doi: 10.1186/1479-5876-9-215
259. Bian Z, Xu LP, Fu Q, Huo M, Liu L, Zhao X, et al. Homeostatic γδ T Cell Contents Are Preserved by Granulocyte Colony-Stimulating Factor Priming and Correlate With the Early Recovery of γδ T Cell Subsets After Haploidentical Hematopoietic Stem Cell Transplantation. Biol Blood Marrow Transplant (2018) 24:252–9. doi: 10.1016/j.bbmt.2017.10.027
260. Xuan L, Wu X, Qiu D, Gao L, Liu H, Fan Z, et al. Regulatory γδ T Cells Induced by G-CSF Participate in Acute Graft-Versus-Host Disease Regulation in G-CSF-Mobilized Allogeneic Peripheral Blood Stem Cell Transplantation. J Transl Med (2018) 16:144. doi: 10.1186/s12967-018-1519-2
261. Otto M, Barfield RC, Iyengar R, Gatewood J, Müller I, Holladay MS, et al. Human γδ T Cells From G-CSF-Mobilized Donors Retain Strong Tumoricidal Activity and Produce Immunomodulatory Cytokines After Clinical-Scale Isolation. J Immunother (2005) 28:73–8. doi: 10.1097/00002371-200501000-00009
262. Dolstra H, Fredrix H, van der Meer A, De Witte T, Figdor C, Van De Wiel-Van Kemenade E. TCR γδ Cytotoxic T Lymphocytes Expressing the Killer Cell-Inhibitory Receptor P58.2 (CD158b) Selectively Lyse Acute Myeloid Leukemia Cells. Bone Marrow Transplant (2001) 27:1087–93. doi: 10.1038/sj.bmt.1703043
263. Baker FL, Bigley AB, Agha NH, Pedlar CR, O’Connor DP, Bond RA, et al. Systemic β-Adrenergic Receptor Activation Augments the Ex Vivo Expansion and Anti-Tumor Activity of Vγ9vδ2 T-Cells. Front Immunol (2020) 10:3082. doi: 10.3389/fimmu.2019.03082
264. Berglund S, Gaballa A, Sawaisorn P, Sundberg B, Uhlin M. Expansion of Gammadelta T Cells From Cord Blood: A Therapeutical Possibility. Stem Cells Int (2018) 2018:1–15. doi: 10.1155/2018/8529104
265. Maniar A, Zhang X, Lin W, Gastman BR, Pauza CD, Strome SE, et al. Human γδ T Lymphocytes Induce Robust NK Cell-Mediated Antitumor Cytotoxicity Through CD137 Engagement. Blood (2010) 116:1726–33. doi: 10.1182/blood-2009-07-234211
266. Kreutzman A, Juvonen V, Kairisto V, Ekblom M, Stenke L, Seggewiss R, et al. Mono/oligoclonal T and NK Cells are Common in Chronic Myeloid Leukemia Patients at Diagnosis and Expand During Dasatinib Therapy. Blood (2010) 116:772–82. doi: 10.1182/blood-2009-12-256800
267. Lamb LS, Henslee-Downey PJ, Parrish RS, Godder K, Thompson J, Lee C, et al. Increased Frequency of Tcrγδ+ T Cells in Disease-Free Survivors Following T Cell-Depleted, Partially Mismatched, Related Donor Bone Marrow Transplantation for Leukemia. J Hematother Stem Cell Res (1996) 5:503–9. doi: 10.1089/scd.1.1996.5.503
268. Godder KT, Henslee-Downey PJ, Mehta J, Park BS, Chiang KY, Abhyankar S, et al. Long Term Disease-Free Survival in Acute Leukemia Patients Recovering With Increased γδ T Cells After Partially Mismatched Related Donor Bone Marrow Transplantation. Bone Marrow Transplant (2007) 39:751–7. doi: 10.1038/sj.bmt.1705650
269. Ravens S, Schultze-Florey C, Raha S, Sandrock I, Drenker M, Oberdörfer L, et al. Human γδ T Cells are Quickly Reconstituted After Stem-Cell Transplantation and Show Adaptive Clonal Expansion in Response to Viral Infection. Nat Immunol (2017) 18:393–401. doi: 10.1038/ni.3686
270. Vilmer E, Guglielmi P, David V, Leca G, Rabian C, Degos L, et al. Predominant Expression of Circulating CD3+ Lymphocytes Bearing Gamma T Cell Receptor in a Prolonged Immunodeficiency After Allogeneic Bone Marrow Transplantation. J Clin Invest (1988) 82:755–61. doi: 10.1172/JCI113675
271. Bertaina A, Zorzoli A, Petretto A, Barbarito G, Inglese E, Merli P, et al. Zoledronic Acid Boosts γδ T-Cell Activity in Children Receiving αβ+ T and CD19+ Cell-Depleted Grafts From an HLA-Haplo-Identical Donor. Oncoimmunology (2017) 6:e1216291. doi: 10.1080/2162402X.2016.1216291
272. Merli P, Algeri M, Galaverna F, Milano GM, Bertaina V, Biagini S, et al. Immune Modulation Properties of Zoledronic Acid on Tcrγδ T-Lymphocytes After Tcrαβ/CD19-Depleted Haploidentical Stem Cell Transplantation: An Analysis on 46 Pediatric Patients Affected by Acute Leukemia. Front Immunol (2020) 11:699. doi: 10.3389/fimmu.2020.00699
273. Lamb LS, Gee AP, Hazlett LJ, Musk P, Parrish RS, O’Hanlon TP, et al. Influence of T Cell Depletion Method on Circulating γδ T Cell Reconstitution and Potential Role in the Graft-Versus-Leukemia Effect. Cytotherapy (1999) 1:7–19. doi: 10.1080/0032472031000141295
274. Arruda LCM, Gaballa A, Uhlin M. Impact of γδ T Cells on Clinical Outcome of Hematopoietic Stem Cell Transplantation: Systematic Review and Meta-Analysis. Blood Adv (2019) 3:3436–48. doi: 10.1182/bloodadvances.2019000682
275. Hirokawa M, Horiuchi T, Kawabata Y, Kitabayashi A, Miura AB. Reconstitution of γδ T Cell Repertoire Diversity After Human Allogeneic Hematopoietic Cell Transplantation and the Role of Peripheral Expansion of Mature T Cell Population in the Graft. Bone Marrow Transplant (2000) 26:177–85. doi: 10.1038/sj.bmt.1702478
276. Cela ME, Holladay MS, Rooney CM, Richardson S, Alexander B, Krance RA, et al. γδ T Lymphocyte Regeneration After T Lymphocyte-Depleted Bone Marrow Transplantation From Mismatched Family Members or Matched Unrelated Donors(1996). Available at: https://europepmc.org/article/med/8640174 (Accessed August 4, 2020).
277. Perko R, Kang G, Sunkara A, Leung W, Thomas PG, Dallas MH. Gamma Delta T Cell Reconstitution Is Associated With Fewer Infections and Improved Event-Free Survival After Hematopoietic Stem Cell Transplantation for Pediatric Leukemia. Biol Blood Marrow Transplant (2015) 21:130–6. doi: 10.1016/j.bbmt.2014.09.027
278. Knight A, Madrigal AJ, Grace S, Sivakumaran J, Kottaridis P, Mackinnon S, et al. The Role of Vδ2-Negative γδ T Cells During Cytomegalovirus Reactivation in Recipients of Allogeneic Stem Cell Transplantation. Blood (2010) 116:2164–72. doi: 10.1182/blood-2010-01-255166
279. Pitard V, Roumanes D, Lafarge X, Couzi L, Garrigue I, Lafon M-E, et al. Long-Term Expansion of Effector/Memory Vδ2– γδ T Cells is a Specific Blood Signature of CMV Infection. Blood (2008) 112:1317–24. doi: 10.1182/blood-2008-01-136713
280. Scheper W, Van Dorp S, Kersting S, Pietersma F, Lindemans C, Hol S, et al. γδt Cells Elicited by CMV Reactivation After Allo-SCT Cross-Recognize CMV and Leukemia. Leukemia (2013) 27:1328–38. doi: 10.1038/leu.2012.374
281. Elmaagacli AH, Steckel NK, Koldehoff M, Hegerfeldt Y, Trenschel R, Ditschkowski M, et al. Early Human Cytomegalovirus Replication After Transplantation is Associated With a Decreased Relapse Risk: Evidence for a Putative Virus-Versus-Leukemia Effect in Acute Myeloid Leukemia Patients. Blood (2011) 118:1402–12. doi: 10.1182/blood-2010-08-304121
282. Farnault L, Gertner-Dardenne J, Gondois-Rey F, Michel G, Chambost H, Hirsch I, et al. Clinical Evidence Implicating Gamma-Delta T Cells in EBV Control Following Cord Blood Transplantation. Bone Marrow Transplant (2013) 48:1478–9. doi: 10.1038/bmt.2013.75
283. Minculescu L, Marquart HV, Ryder LP, Andersen NS, Schjoedt I, Friis LS, et al. Improved Overall Survival, Relapse-Free-Survival, and Less Graft-vs.-Host-Disease in Patients With High Immune Reconstitution of TCR Gamma Delta Cells 2 Months After Allogeneic Stem Cell Transplantation. Front Immunol (2019) 10:1997. doi: 10.3389/fimmu.2019.01997
284. Liu J, Bian Z, Wang X, Xu LP, Fu Q, Wang C, et al. Inverse Correlation of Vδ2+ T-Cell Recovery With EBV Reactivation After Haematopoietic Stem Cell Transplantation. Br J Haematol (2018) 180:276–85. doi: 10.1111/bjh.15037
285. Liu J, Gao H, Xu LP, Mo XD, Liu R, Liang S, et al. Immunosuppressant Indulges EBV Reactivation and Related Lymphoproliferative Disease by Inhibiting Vδ2+ T Cells Activities After Hematopoietic Transplantation for Blood Malignancies. J Immunother Cancer (2020) 8(1–13):e000208. doi: 10.1136/jitc-2019-000208
286. Wilhelm M, Kunzmann V, Eckstein S, Reimer P, Weissinger F, Ruediger T, et al. γδ T Cells for Immune Therapy of Patients With Lymphoid Malignancies. Blood (2003) 102:200–6. doi: 10.1182/blood-2002-12-3665
287. Kunzmann V, Smetak M, Kimmel B, Weigang-Koehler K, Goebeler M, Birkmann J, et al. Tumor-Promoting Versus Tumor-Antagonizing Roles of γδ T Cells in Cancer Immunotherapy: Results From a Prospective Phase I/II Trial. J Immunother (2012) 35:205–13. doi: 10.1097/CJI.0b013e318245bb1e
288. Wilhelm M, Smetak M, Schaefer-Eckart K, Kimmel B, Birkmann J, Einsele H, et al. Successful Adoptive Transfer and In Vivo Expansion of Haploidentical γδ T Cells. J Transl Med (2014) 12:1–5. doi: 10.1186/1479-5876-12-45
Keywords: gamma-delta T cells, leukemic microenvironment, off-the-shelf cell therapy, clinical trials, cell transplantation
Citation: Barros MS, de Araújo ND, Magalhães-Gama F, Pereira Ribeiro TL, Alves Hanna FS, Tarragô AM, Malheiro A and Costa AG (2021) γδ T Cells for Leukemia Immunotherapy: New and Expanding Trends. Front. Immunol. 12:729085. doi: 10.3389/fimmu.2021.729085
Received: 22 June 2021; Accepted: 30 August 2021;
Published: 22 September 2021.
Edited by:
Nicolas Dulphy, Université de Paris, FranceCopyright © 2021 Barros, de Araújo, Magalhães-Gama, Pereira Ribeiro, Alves Hanna, Tarragô, Malheiro and Costa. This is an open-access article distributed under the terms of the Creative Commons Attribution License (CC BY). The use, distribution or reproduction in other forums is permitted, provided the original author(s) and the copyright owner(s) are credited and that the original publication in this journal is cited, in accordance with accepted academic practice. No use, distribution or reproduction is permitted which does not comply with these terms.
*Correspondence: Allyson Guimarães Costa, allyson.gui.costa@gmail.com
†These authors have contributed equally to this work