The Role of Activity-Dependent DNA Demethylation in the Adult Brain and in Neurological Disorders
- 1RG Neuroplasticity, Leibniz Institute for Neurobiology, Magdeburg, Germany
- 2Leibniz Group ‘Dendritic Organelles and Synaptic Function’, Center for Molecular Neurobiology (ZMNH), University Medical Center Hamburg-Eppendorf, Hamburg, Germany
Over the last decade, an increasing number of reports underscored the importance of epigenetic regulations in brain plasticity. Epigenetic elements such as readers, writers and erasers recognize, establish, and remove the epigenetic tags in nucleosomes, respectively. One such regulation concerns DNA-methylation and demethylation, which are highly dynamic and activity-dependent processes even in the adult neurons. It is nowadays widely believed that external stimuli control the methylation marks on the DNA and that such processes serve transcriptional regulation in neurons. In this mini-review, we cover the current knowledge on the regulatory mechanisms controlling in particular DNA demethylation as well as the possible functional consequences in health and disease.
Introduction
Among several other epigenetic tags, methyl tags on the DNA were generally considered as repressive marks. However, an increasing number of studies showed that the DNA methylation at intergenic regions as well as gene regulatory regions might enhance gene expression (Bayraktar and Kreutz, 2017). How, the key enzymes in DNA methylation, DNA methyltransferases (DNMTs), are differentially regulated and perform the DNA methylation are well characterized (Goll and Bestor, 2005; Bayraktar and Kreutz, 2017). However, the removal of methyl tags from the DNA has been more perplexing. The reversal of DNA methylation can take place passively by diluting the DNA methylation of both copies of the genome following multiple rounds of cell division in the absence of maintenance of DNA methylation (Inoue and Zhang, 2011). In postmitotic neurons, other mechanisms must be in place. Current opinion disfavors the direct removal of the covalent bond between the methyl groups and cytosines (Ooi and Bestor, 2008). A unifying mechanistic process on how active DNA demethylation is still lacking. We, therefore, discuss how active DNA demethylation is achieved by the interplay of DNA oxidative reactions and repair mechanisms.
Mechanisms of Active DNA Demethylation
Several proteins have been identified that are part of neuronal demethylation machinery. These include Growth Arrest and DNA Damage-inducible (GADD) 45 proteins (GADD45A and GADD45B) that in neurons take part in active DNA demethylation processes (Barreto et al., 2007; Ma et al., 2009). Termed initially as the MyD118 (myeloid differentiation), Gadd45b was identified as an immediate gene whose expression was induced following the induction of long-term potentiation (LTP) in vivo (Hevroni et al., 1998). GADD45B mediated activity-dependent demethylation was first shown for the promoter of fibroblast growth factor 1, isoform B (Fgf1B) and Brain-derived neurotrophic factor (Bdnf) 9. It is nowadays widely believed that GADD45B contributes to demethylation in conjunction with other modifiers which will be discussed below.
5-hydroxymethyl cytosine (5hmC) was first described in the 1972 (Penn et al., 1972) and more than three decades later enzymatic activity of Ten-eleven translocation (TET) proteins was discovered to biochemically convert 5mC to 5hmC (Tahiliani et al., 2009; Ito et al., 2010). The characterization of TET enzymes (Tahiliani et al., 2009; Ito et al., 2010) and 5hmC in the brain (Kriaucionis and Heintz, 2009) also advanced our understanding of active DNA demethylation in neurons. In successive oxidation steps 5mC is initially converted to 5hmC which is followed by the conversion to 5-formylcytosine (5fC) and subsequently 5-carboxylcytosine (5cC). Each of these steps requires one of the three TET enzymes (Ito et al., 2011; Figure 1). 5fC and 5caC can be recognized and excised by Thymine DNA Glycosylase (TDG) generating an apyrimidinic (AP) site (He et al., 2011; Maiti and Drohat, 2011). The AP site is then corrected by specific base-excision repair mechanism (BER) with the replacement of cytosine in mammals (Zhu, 2009). TDG depletion in embryonic stem cells causes enhanced levels of 5fC and 5caC at proximal and distal gene regulatory elements (Raiber et al., 2012; Shen et al., 2013). TDG knockout or catalytical inactivation leads to embryonic lethality in mice and hypermethylated CpG islands (Cortellino et al., 2011). Along these lines, the perturbation of BER enzymes by genetic and pharmacological inhibition results in the partial block of global DNA demethylation in mouse germ line (Hajkova et al., 2010). Collectively this evidence suggests that BER has an evolutionarily conserved role in active DNA demethylation.
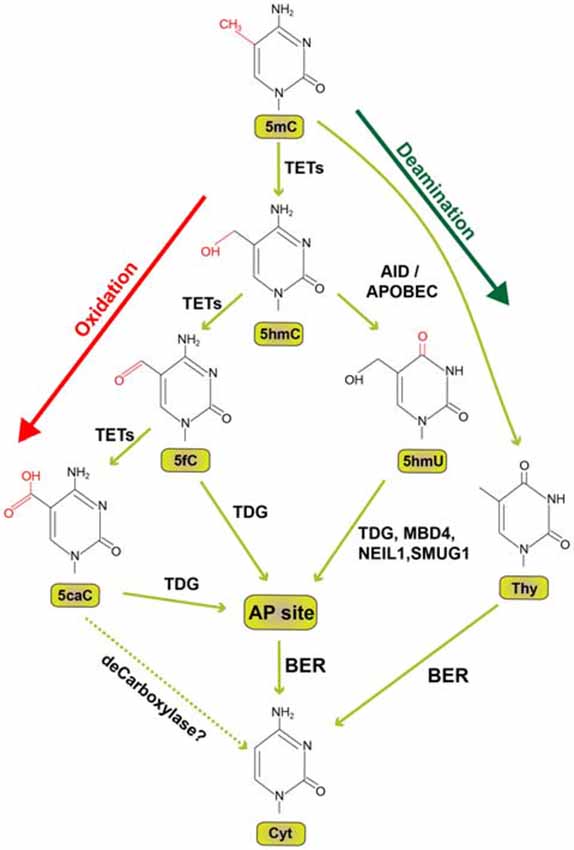
Figure 1. Pathways of active DNA demethylation. Since the formerly hypothesized demethylase to directly convert 5-methylcytosine (5mC) to cytosine (Cyt) has not been identified, we depict here the current view how active DNA demethylation might take place. 5mC is oxidized by ten-eleven translocation (TET) family of dioxygenases to generate 5-hydroxymethylcytosine (5hmC). In successive steps TET enzymes further hydroxylate 5hmC to generate 5-formylcytosine (5fC) and 5-carboxylcytosine (5caC). Thymine DNA glycosylase (TDG) recognizes intermediate DNA forms 5fC and 5caC and excises the glycosidic bond resulting in an apyrimidinic (AP) site. In an alternative deamination pathway 5hmC can be deaminated by activity-induced cytidine deaminase/apolipoprotein B mRNA editing complex (AID/APOBEC) deaminases to form 5-hydroxymethyluracil (5hmU) or 5mC can be converted to Thymine (Thy). 5hmU can be cleaved by TDG, single-strand-selective monofunctional uracil-DNA glycosylase 1 (SMUG1), Nei-Like DNA Glycosylase 1 (NEIL1), or methyl-CpG binding protein 4 (MBD4). AP sites and T:G mismatches can be efficiently repaired by Base Excision Repair (BER) enzymes. Dotted lines indicate a proposed but not experimentally proven path.
The finding that TDG rapidly processes the oxidation products by TET enzymes corroborated the view of a TET-initiated, TDG-processed and BER-terminated active DNA demethylation mechanism. In support of this picture and the surmised recruiting function of GADD45B, Li et al. (2015) reported that GADD45A as well as GADD45B promote demethylation of an in vitro methylated promoter through TDG. TDG physically interacts with GADD45 and in the presence of the triple complex, GADD45B, TDG and TET2, a complete demethylation of reporters could be achieved (Li et al., 2015). TDG has several interesting and yet not well-understood features. It interacts with DNMT3A either via the PWWP or the catalytic domain of DNMT3A (see Figure 2). This interaction enhances TDG activity possibly by facilitating the binding of TDG to the mismatch sites while binding to TDG at the same time represses DNMT3A methyltransferase activity (Li et al., 2007). TDG is one of the two enzymes that together with the methyl-CpG binding domain protein 4 (MBD4) is known to initiate this repair mechanism (Hardeland et al., 2001; Krokan et al., 2002). Both TDG and MBD4 possess low levels of 5mC DNA glycosylase activity in vitro (Zhu et al., 2000a,b).
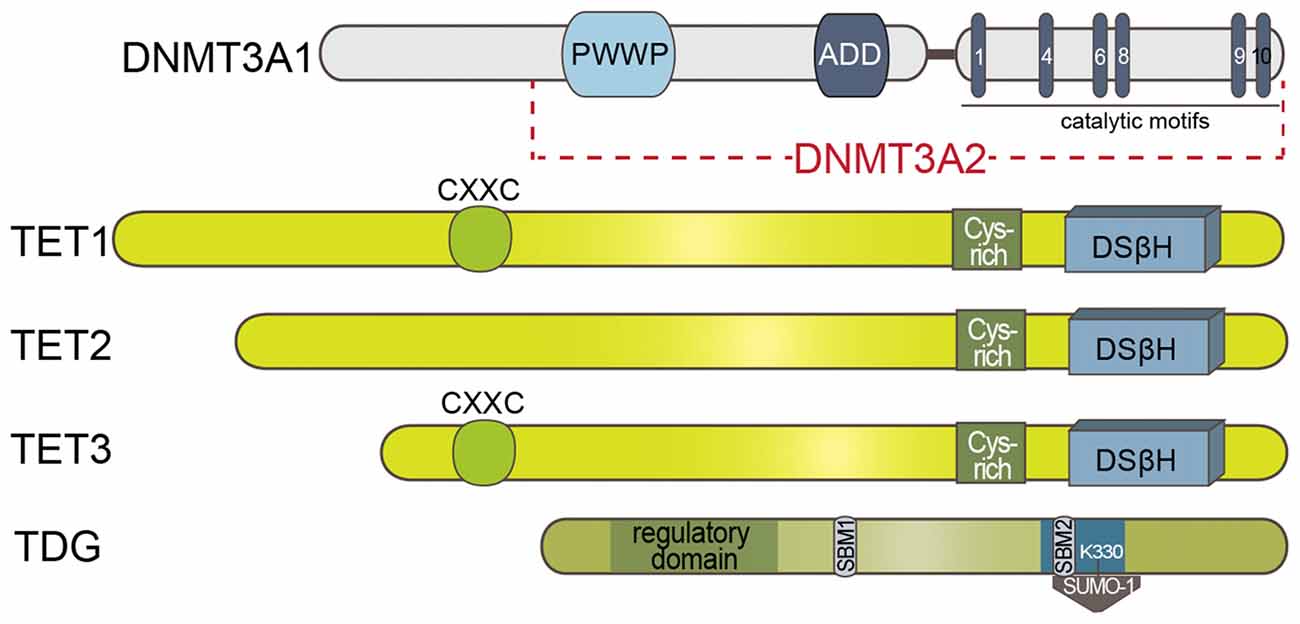
Figure 2. Schematic presentation of the known domain structures of proteins involved in DNA methylation/demethylation. DNMT3A1/2: ADD domain of DNMT3A1/2 is involved in the allosteric control of the enzyme. TET enzymes: DNA binding CXXC motif is present in TET1 and TET3. Double-stranded β-helix (DSβH) is the fold core oxygenase domain is preceded by a cysteine (Cys)-rich domain. Sumo-binding motifs (SBM) and catalytic residues (in blue) of TDG is represented. PWWP: Pro-Trp-Trp-Pro; CXXC: Cys-X-X-Cys motif.
Deamination of 5mC and 5hmC by Activity Induced Cytosine Deaminase (AID) or apolipoprotein B mRNA editing complex (APOBEC) is an alternative path to successive oxidation reactions by TET enzymes for the initiation of DNA demethylation (Figure 1). The modified nucleotide can then be replaced by BER. Shortly after the discovery of AID (Muramatsu et al., 1999), a functional role in DNA demethylation by deamination was proposed (Rai et al., 2008; Bhutani et al., 2010). The identification of AID in a ternary complex with GADD45A and TDG also indicates a contribution of AID in the demethylation process (Cortellino et al., 2011). On the contrary, several independent studies revealed that 5mC and 5hmC are poorer substrates for AID as compared to cytosine (Larijani et al., 2005; Nabel et al., 2012; Rangam et al., 2012; Abdouni et al., 2013). The contribution of AID for the deamination pathway to demethylate 5mC in association with BER mechanism is rather elusive since the enzyme cannot efficiently deaminate 5mC (Wijesinghe and Bhagwat, 2012). However, the same study showed efficient 5mC deamination capability of APOBEC3 (Wijesinghe and Bhagwat, 2012). In conclusion, the role of AID in DNA demethylation particularly in the adult brain is still unclear (for review, see Bochtler et al., 2017).
Regulation of Neuronal Gene Expression by Active DNA Demethylation
In the long-lived nature of postmitotic neurons, genomic stability needs to be maintained for decades while at the same time their remarkable plasticity has to be kept at a poised state ready to respond (Baker-Andresen et al., 2013). How are stability and permissiveness for changes in DNA methylation achieved upon enhanced neuronal activity? It is tempting to speculate that due to its plastic nature the basal epigenomic state of hippocampal neuron determines the permissiveness for an initial wave of transcription of DNA modifiers, including demethylation machinery component expressions, which precedes effector gene expression (Oliveira, 2016). An example for a methylation mark keeping the gene in a silent but in a transcriptionally poised state is the promoter methylation of the Bdnf gene that is quite well investigated in the context of synaptic plasticity and learning (Miller and Sweatt, 2007; Lubin et al., 2008). In differentiated neurons, the Bdnf promoter is methylated at basal conditions and thereby kept in a repressed state by the occupation of repressor complex involving RE1-Silencing Transcription factor corepressor (CoRest), methyl CpG binding protein 2 (MeCP2), histone deacetylases (HDAC) 1 and 2. The repressor complex dissociates following phosphorylation of MeCP2 and nitrosylation of HDAC2 in response to Ca+2 influx (Chen et al., 2003; Nott et al., 2008). Activity-induced deaminase (AID) regulates the induced expression of Bdnf IV in a stimulus-dependent manner (Ratnu et al., 2014). However, the yet unclear status of AID in DNA demethylation makes it hard to directly link the effect of AID on Bdnf expression to activity-dependent DNA demethylation.
Unfortunately, conflicting reports have been published on the role of GADD45B in learning and memory processes. Fear conditioning induces Gadd45b gene expression (Keeley et al., 2006) and deletion of the gene results in hippocampus-dependent long-term memory deficits including fear conditioning (Leach et al., 2012). However, others found improved long-term memory following Gadd45b knockout mice employing a similar contextual fear-conditioning paradigm (Sultan et al., 2012). Of note, the mice strains used in the latter two studies had a different genetic background (C57BL/6 and B6:129VJ mice) which might account for the discrepant results. Moreover, targeted siRNA delivery to transiently knock down Gadd45b expression in the neonatal rat amygdala results in altered juvenile behavior with consequences for the expression of Bdnf, MeCP2 and Reelin (Kigar et al., 2015). NF-κB, which is known to be important in hippocampus-dependent memory formation (Kaltschmidt and Kaltschmidt, 2015), was proposed to regulate Gadd45b gene expression and thereby DNA demethylation activity (Jarome et al., 2015). Interestingly, overexpression of TET1 leads to enhanced expression of several memory-related genes but surprisingly to impairment of contextual fear memory (Kaas et al., 2013). It is possible that TET proteins might have functions independent of DNA hydroxymethylation. TET3, for instance, was recently shown to have a functional role in scaling-down synaptic strength in hippocampal neurons (Yu et al., 2015).
Interestingly, 5hmC is not only an intermediate DNA demethylation form but also an epigenetic mark on its own, which is enriched within promoters and gene bodies (Kaas et al., 2013). This enrichment correlates with a depletion of 5mC in actively transcribed genes. Moreover, gene body 5mC and gene expression are inversely correlated (Mellen et al., 2012). Recent advances in whole epigenome analysis identified gradually accumulating non-CG methylation (mCH, H = A/C/T) from post-natal week one onwards in the genome peaking in the adult mouse brain (Xie et al., 2012; Lister et al., 2013) and at several hundred genomic positions in the adult human brain (Varley et al., 2013). Genes expressed in the mammalian brain are devoid of intragenic and promoter mCH (Xie et al., 2012) and mCH correlates with decreased gene expression in a highly cell type-specific manner (Mo et al., 2015). mCH accumulation is implicated in X chromosome inactivation and might therefore contribute to gender specific gene expression (Keown et al., 2017). Reconfiguration of the global DNA methylome during development coincides with synaptogenesis, a period in which mCH accumulates in neurons but not in glial cells (Lister et al., 2013). On the other hand, methylated CpG-rich DNA regions are not only found in transcription initiation sites but also in gene bodies and intergenic regions (Jones, 2012). Collectively these studies illustrate that it is important to identify the location and type of DNA methylation to assess its contribution to gene expression.
Another critical issue is cell-type specificity of DNA demethylation. In most studies brain tissue that contains different neuronal and glial cell types was used. The current knowledge on how the DNA demethylation machinery functions in different cell-types and responds to neuronal activity is therefore very limited. DNA methylation patterns vary between neurons and non-neuronal cells. Ventromedial prefrontal cortex neurons have higher global DNA methylation levels compared to non-neuronal cells (Li et al., 2014a). Early life stress (ELS) alters DNA methylation and Bdnf expression in medial prefrontal cortex neurons in a cell-type and sex-specific manner (Blaze and Roth, 2017). The expression of Bdnf IX and Fgf1B genes, which are crucially involved in neurogenesis and plasticity, is also regulated by Gadd45b in an activity-dependent manner in granule cells of the dentate gyrus (Ma et al., 2009). Furthermore, Halder et al. (2016) showed that DNA methylation and changes in histone acetylation occur in parallel following contextual fear conditioning learning and alterations in DNA methylation may also arise in non-neuronal cells potentially supporting an epigenetic code for memory formation. Interestingly, in contrast to hippocampal neurons, TET3 but not TET1 is expressed in cortical neurons in an activity-dependent manner (Li et al., 2014b). Gephyrin stabilizes GABA receptors to postsynaptic membrane and takes part in fear extinction (Chhatwal et al., 2005). Li et al. (2014b) further validated the enhanced expression of TET3 on the gephyrin locus where they showed increased occupancy of TET3 in association with an accumulation of the demethylation intermediate mark 5hmC.
Role of DNA Demethylation in Neurological Disorders
Given the principal functions of chromatin modifications in regulating gene transcription programs, it’s not surprising that the number of studies, which report the involvement of DNA demethylation machinery in neurological disorders, is steadily increasing. Enhanced GADD45B levels were reported in two different cohorts of major psychotic patients (Gavin et al., 2012). However, reduced occupancy of GADD45B on the Bdnf IX promoter was found, which is in line with reduced Bdnf IX expression (Gavin et al., 2012). Recently, GADD45B expression was shown to be regulated by transforming growth factor beta (TGFB) signaling and protein levels of GADD45B are reduced in a model of chronic mild stress (Grassi et al., 2017). Moreover, a reduction in expression levels of the immediate early gene Arc was also associated with reduced levels of GADD45B and DNA demethylation in this stress model (Grassi et al., 2017). Although its neurobiological underpinnings have not been fully understood, electroconvulsive therapy (ECT) is currently in clinical practice for the treatment of several psychiatric diseases including depression (Singh and Kar, 2017). In an animal model ECT reduces the methylation levels of Bdnf 9 promoter, hence inducing the mRNA expression of the gene, however, in the transgenic mice model in which Gadd45b was knocked out the effect of ECT on the Bdnf IX promoter methylation level is abolished and mRNA expression is perturbed (Ma et al., 2009). Prenatally stressed mice exhibit not only similar behavioral traits like psychotic patients but also similar epigenetic signatures (Dong et al., 2015). DNA methyltransferase1 and TET1 enzyme level increase in prenatally stressed mice correlates with enhanced 5mC and 5hmC in the regulatory DNA regions and hence decreased Bdnf gene expression (Dong et al., 2015). Samples from patients that suffered from bipolar disorder and schizophrenia show enhanced methylation of associated gene promoters resulting in suppressed expression (Grayson and Guidotti, 2013). This is linked to the enhanced expression of DNMTs (Veldic et al., 2004; Zhubi et al., 2009). However, it’s not clear whether the lack of active DNA demethylation can also be responsible for the disease etiology in some cases. The contribution of methylation and active DNA demethylation in Alzheimer’s disease (AD) remains to be determined. The varying global methylation levels reported in the postmortem brain samples can be region specific (Roubroeks et al., 2017). There are conflicting studies on the increase in 5mC and 5hmC in the hippocampus whereas no changes in the entorhinal cortex in AD as compared to controls were reported (Bradley-Whitman and Lovell, 2013; Lashley et al., 2015). Contradictory evidence on the global decrease in methylation levels in the hippocampus and entorhinal cortex was published by others (Mastroeni et al., 2010; Chouliaras et al., 2013). In a complex disease like AD, the readout from brain samples and genome-wide association studies on various chromatin modifiers is hard to interpret because of the readout’s variability due to the initiation, progression or terminal stage of the disease.
Concluding Remarks
Based on the initial GADD45B-dependent demethylation hypothesis (Gavin et al., 2012), current data suggest that active demethylation in postmitotic neurons is initiated by TET family enzymes in conjunction with TDG. While GADD45B apparently lacks enzymatic activity, it seems to recruit demethylation machinery components to certain promoters by yet unknown mechanisms. The cascade of events in active DNA demethylation finally requires the contribution of BER mechanism to generate mark-free cytosine. There are several gaps in our understanding of the DNA demethylation pathway in neurons. For instance, how are the DNA demethylation components targeted to specific genomic sites? Finally, it is yet unclear how one can interfere with this machinery to regulate activity-dependent gene expression and whether this machinery has druggable pathways in the context of neurological disorders.
Author Contributions
GB and MK are invited to contribute to the article collection for the special issue of the “Epigenetic Mechanisms Regulating Neural Plasticity”. GB outlined the mini review and GB and MK wrote the manuscript.
Funding
This work was supported by grants from the Deutsche Forschungsgemeinschaft (DFG; Kr1879/5-1/6-1/SFB 779 TPB8), BMBF “Energi” FKZ: 01GQ1421B, The EU Joint Programme—Neurodegenerative Disease Research (JPND) project STAD and Leibniz Foundation to MK.
Conflict of Interest Statement
The authors declare that the research was conducted in the absence of any commercial or financial relationships that could be construed as a potential conflict of interest.
References
Abdouni, H., King, J. J., Suliman, M., Quinlan, M., Fifield, H., and Larijani, M. (2013). Zebrafish AID is capable of deaminating methylated deoxycytidines. Nucleic Acids Res. 41, 5457–5468. doi: 10.1093/nar/gkt212
Baker-Andresen, D., Ratnu, V. S., and Bredy, T. W. (2013). Dynamic DNA methylation: a prime candidate for genomic metaplasticity and behavioral adaptation. Trends Neurosci. 36, 3–13. doi: 10.1016/j.tins.2012.09.003
Barreto, G., Schäfer, A., Marhold, J., Stach, D., Swaminathan, S. K., Handa, V., et al. (2007). Gadd45a promotes epigenetic gene activation by repair-mediated DNA demethylation. Nature 445, 671–675. doi: 10.1038/nature05515
Bayraktar, G., and Kreutz, M. R. (2017). Neuronal DNA methyltransferases: epigenetic mediators between synaptic activity and gene expression? Neuroscientist 24, 171–185. doi: 10.1177/1073858417707457
Bhutani, N., Brady, J. J., Damian, M., Sacco, A., Corbel, S. Y., and Blau, H. M. (2010). Reprogramming towards pluripotency requires AID-dependent DNA demethylation. Nature 463, 1042–1047. doi: 10.1038/nature08752
Blaze, J., and Roth, T. L. (2017). Caregiver maltreatment causes altered neuronal DNA methylation in female rodents. Dev. Psychopathol. 29, 477–489. doi: 10.1017/s0954579417000128
Bochtler, M., Kolano, A., and Xu, G. L. (2017). DNA demethylation pathways: additional players and regulators. Bioessays 39, 1–13. doi: 10.1002/bies.201600178
Bradley-Whitman, M. A., and Lovell, M. A. (2013). Epigenetic changes in the progression of Alzheimer’s disease. Mech. Ageing Dev. 134, 486–495. doi: 10.1016/j.mad.2013.08.005
Chen, W. G., Chang, Q., Lin, Y., Meissner, A., West, A. E., Griffith, E. C., et al. (2003). Derepression of BDNF transcription involves calcium-dependent phosphorylation of MeCP2. Science 302, 885–889. doi: 10.1126/science.1086446
Chhatwal, J. P., Myers, K. M., Ressler, K. J., and Davis, M. (2005). Regulation of gephyrin and GABAA receptor binding within the amygdala after fear acquisition and extinction. J. Neurosci. 25, 502–506. doi: 10.1523/JNEUROSCI.3301-04.2005
Chouliaras, L., Mastroeni, D., Delvaux, E., Grover, A., Kenis, G., Hof, P. R., et al. (2013). Consistent decrease in global DNA methylation and hydroxymethylation in the hippocampus of Alzheimer’s disease patients. Neurobiol. Aging 34, 2091–2099. doi: 10.1016/j.neurobiolaging.2013.02.021
Cortellino, S., Xu, J., Sannai, M., Moore, R., Caretti, E., Cigliano, A., et al. (2011). Thymine DNA glycosylase is essential for active DNA demethylation by linked deamination-base excision repair. Cell 146, 67–79. doi: 10.1016/j.cell.2011.06.020
Dong, E., Ruzicka, W. B., Grayson, D. R., and Guidotti, A. (2015). DNA-methyltransferase1 (DNMT1) binding to CpG rich GABAergic and BDNF promoters is increased in the brain of schizophrenia and bipolar disorder patients. Schizophr. Res. 167, 35–41. doi: 10.1016/j.schres.2014.10.030
Gavin, D. P., Sharma, R. P., Chase, K. A., Matrisciano, F., Dong, E., and Guidotti, A. (2012). Growth arrest and DNA-damage-inducible, β (GADD45b)-mediated DNA demethylation in major psychosis. Neuropsychopharmacology 37, 531–542. doi: 10.1038/npp.2011.221
Goll, M. G., and Bestor, T. H. (2005). Eukaryotic cytosine methyltransferases. Annu. Rev. Biochem. 74, 481–514. doi: 10.1146/annurev.biochem.74.010904.153721
Grassi, D., Franz, H., Vezzali, R., Bovio, P., Heidrich, S., Dehghanian, F., et al. (2017). Neuronal activity, TGFβ-signaling and unpredictable chronic stress modulate transcription of Gadd45 family members and DNA methylation in the hippocampus. Cereb. Cortex 27, 4166–4181. doi: 10.1093/cercor/bhx095
Grayson, D. R., and Guidotti, A. (2013). The dynamics of DNA methylation in schizophrenia and related psychiatric disorders. Neuropsychopharmacology 38, 138–166. doi: 10.1038/npp.2012.125
Hajkova, P., Jeffries, S. J., Lee, C., Miller, N., Jackson, S. P., and Surani, M. A. (2010). Genome-wide reprogramming in the mouse germ line entails the base excision repair pathway. Science 329, 78–82. doi: 10.1126/science.1187945
Halder, R., Hennion, M., Vidal, R. O., Shomroni, O., Rahman, R. U., Rajput, A., et al. (2016). DNA methylation changes in plasticity genes accompany the formation and maintenance of memory. Nat. Neurosci. 19, 102–110. doi: 10.1038/nn.4194
Hardeland, U., Bentele, M., Lettieri, T., Steinacher, R., Jiricny, J., and Schar, P. (2001). Thymine DNA glycosylase. Prog. Nucleic Acid Res. Mol. Biol. 68, 235–253. doi: 10.1016/S0079-6603(01)68103-0
He, Y. F., Li, B. Z., Li, Z., Liu, P., Wang, Y., Tang, Q., et al. (2011). Tet-mediated formation of 5-carboxylcytosine and its excision by TDG in mammalian DNA. Science 333, 1303–1307. doi: 10.1126/science.1210944
Hevroni, D., Rattner, A., Bundman, M., Lederfein, D., Gabarah, A., Mangelus, M., et al. (1998). Hippocampal plasticity involves extensive gene induction and multiple cellular mechanisms. J. Mol. Neurosci. 10, 75–98. doi: 10.1007/bf02737120
Inoue, A., and Zhang, Y. (2011). Replication-dependent loss of 5-hydroxymethylcytosine in mouse preimplantation embryos. Science 334:194. doi: 10.1126/science.1212483
Ito, S., D’Alessio, A. C., Taranova, O. V., Hong, K., Sowers, L. C., and Zhang, Y. (2010). Role of Tet proteins in 5mC to 5hmC conversion, ES-cell self-renewal and inner cell mass specification. Nature 466, 1129–1133. doi: 10.1038/nature09303
Ito, S., Shen, L., Dai, Q., Wu, S. C., Collins, L. B., Swenberg, J. A., et al. (2011). Tet proteins can convert 5-methylcytosine to 5-formylcytosine and 5-carboxylcytosine. Science 333, 1300–1303. doi: 10.1126/science.1210597
Jarome, T. J., Butler, A. A., Nichols, J. N., Pacheco, N. L., and Lubin, F. D. (2015). NF-κB mediates Gadd45β expression and DNA demethylation in the hippocampus during fear memory formation. Front. Mol. Neurosci. 8:54. doi: 10.3389/fnmol.2015.00054
Jones, P. A. (2012). Functions of DNA methylation: islands, start sites, gene bodies and beyond. Nat. Rev. Genet. 13, 484–492. doi: 10.1038/nrg3230
Kaas, G. A., Zhong, C., Eason, D. E., Ross, D. L., Vachhani, R. V., Ming, G. L., et al. (2013). TET1 controls CNS 5-methylcytosine hydroxylation, active DNA demethylation, gene transcription and memory formation. Neuron 79, 1086–1093. doi: 10.1016/j.neuron.2013.08.032
Kaltschmidt, B., and Kaltschmidt, C. (2015). NF-KappaB in long-term memory and structural plasticity in the adult mammalian brain. Front. Mol. Neurosci. 8:69. doi: 10.3389/fnmol.2015.00069
Keeley, M. B., Wood, M. A., Isiegas, C., Stein, J., Hellman, K., Hannenhalli, S., et al. (2006). Differential transcriptional response to nonassociative and associative components of classical fear conditioning in the amygdala and hippocampus. Learn. Mem. 13, 135–142. doi: 10.1101/lm.86906
Keown, C. L., Berletch, J. B., Castanon, R., Nery, J. R., Disteche, C. M., Ecker, J. R., et al. (2017). Allele-specific non-CG DNA methylation marks domains of active chromatin in female mouse brain. Proc. Natl. Acad. Sci. U S A 114, E2882–E2890. doi: 10.1073/pnas.1611905114
Kigar, S. L., Chang, L., and Auger, A. P. (2015). Gadd45b is an epigenetic regulator of juvenile social behavior and alters local pro-inflammatory cytokine production in the rodent amygdala. Brain Behav. Immun. 46, 60–69. doi: 10.1016/j.bbi.2015.02.018
Kriaucionis, S., and Heintz, N. (2009). The nuclear DNA base 5-hydroxymethylcytosine is present in Purkinje neurons and the brain. Science 324, 929–930. doi: 10.1126/science.1169786
Krokan, H. E., Drablos, F., and Slupphaug, G. (2002). Uracil in DNA—occurrence, consequences and repair. Oncogene 21, 8935–8948. doi: 10.1038/sj.onc.1205996
Larijani, M., Frieder, D., Sonbuchner, T. M., Bransteitter, R., Goodman, M. F., Bouhassira, E. E., et al. (2005). Methylation protects cytidines from AID-mediated deamination. Mol. Immunol. 42, 599–604. doi: 10.1016/j.molimm.2004.09.007
Lashley, T., Gami, P., Valizadeh, N., Li, A., Revesz, T., and Balazs, R. (2015). Alterations in global DNA methylation and hydroxymethylation are not detected in Alzheimer’s disease. Neuropathol. Appl. Neurobiol. 41, 497–506. doi: 10.1111/nan.12183
Leach, P. T., Poplawski, S. G., Kenney, J. W., Hoffman, B., Liebermann, D. A., Abel, T., et al. (2012). Gadd45b knockout mice exhibit selective deficits in hippocampus-dependent long-term memory. Learn. Mem. 19, 319–324. doi: 10.1101/lm.024984.111
Li, X., Baker-Andresen, D., Zhao, Q., Marshall, V., and Bredy, T. W. (2014a). Methyl CpG binding domain ultra-sequencing: a novel method for identifying inter-individual and cell-type-specific variation in DNA methylation. Genes Brain Behav. 13, 721–731. doi: 10.1111/gbb.12150
Li, X., Wei, W., Zhao, Q. Y., Widagdo, J., Baker-Andresen, D., Flavell, C. R., et al. (2014b). Neocortical Tet3-mediated accumulation of 5-hydroxymethylcytosine promotes rapid behavioral adaptation. Proc. Natl. Acad. Sci. U S A 111, 7120–7125. doi: 10.1073/pnas.1318906111
Li, Z., Gu, T. P., Weber, A. R., Shen, J. Z., Li, B. Z., Xie, Z. G., et al. (2015). Gadd45a promotes DNA demethylation through TDG. Nucleic Acids Res. 43, 3986–3997. doi: 10.1093/nar/gkv283
Li, Y. Q., Zhou, P. Z., Zheng, X. D., Walsh, C. P., and Xu, G. L. (2007). Association of Dnmt3a and thymine DNA glycosylase links DNA methylation with base-excision repair. Nucleic Acids Res. 35, 390–400. doi: 10.1093/nar/gkl1052
Lister, R., Mukamel, E. A., Nery, J. R., Urich, M., Puddifoot, C. A., Johnson, N. D., et al. (2013). Global epigenomic reconfiguration during mammalian brain development. Science 341:1237905. doi: 10.1126/science.1237905
Lubin, F. D., Roth, T. L., and Sweatt, J. D. (2008). Epigenetic regulation of BDNF gene transcription in the consolidation of fear memory. J. Neurosci. 28, 10576–10586. doi: 10.1523/JNEUROSCI.1786-08.2008
Ma, D. K., Jang, M. H., Guo, J. U., Kitabatake, Y., Chang, M. L., Pow-Anpongkul, N., et al. (2009). Neuronal activity-induced Gadd45b promotes epigenetic DNA demethylation and adult neurogenesis. Science 323, 1074–1077. doi: 10.1126/science.1166859
Maiti, A., and Drohat, A. C. (2011). Thymine DNA glycosylase can rapidly excise 5-formylcytosine and 5-carboxylcytosine: potential implications for active demethylation of CpG sites. J. Biol. Chem. 286, 35334–35338. doi: 10.1074/jbc.C111.284620
Mastroeni, D., Grover, A., Delvaux, E., Whiteside, C., Coleman, P. D., and Rogers, J. (2010). Epigenetic changes in Alzheimer’s disease: decrements in DNA methylation. Neurobiol. Aging 31, 2025–2037. doi: 10.1016/j.neurobiolaging.2008.12.005
Mellen, M., Ayata, P., Dewell, S., Kriaucionis, S., and Heintz, N. (2012). MeCP2 binds to 5hmC enriched within active genes and accessible chromatin in the nervous system. Cell 151, 1417–1430. doi: 10.1016/j.cell.2012.11.022
Miller, C. A., and Sweatt, J. D. (2007). Covalent modification of DNA regulates memory formation. Neuron 53, 857–869. doi: 10.1016/j.neuron.2007.02.022
Mo, A., Mukamel, E. A., Davis, F. P., Luo, C., Henry, G. L., Picard, S., et al. (2015). Epigenomic signatures of neuronal diversity in the mammalian brain. Neuron 86, 1369–1384. doi: 10.1016/j.neuron.2015.05.018
Muramatsu, M., Sankaranand, V. S., Anant, S., Sugai, M., Kinoshita, K., Davidson, N. O., et al. (1999). Specific expression of activation-induced cytidine deaminase (AID), a novel member of the RNA-editing deaminase family in germinal center B cells. J. Biol. Chem. 274, 18470–18476. doi: 10.1074/jbc.274.26.18470
Nabel, C. S., Jia, H., Ye, Y., Shen, L., Goldschmidt, H. L., Stivers, J. T., et al. (2012). AID/APOBEC deaminases disfavor modified cytosines implicated in DNA demethylation. Nat. Chem. Biol. 8, 751–758. doi: 10.1038/nchembio.1042
Nott, A., Watson, P. M., Robinson, J. D., Crepaldi, L., and Riccio, A. (2008). S-Nitrosylation of histone deacetylase 2 induces chromatin remodelling in neurons. Nature 455, 411–415. doi: 10.1038/nature07238
Oliveira, A. M. (2016). DNA methylation: a permissive mark in memory formation and maintenance. Learn. Mem. 23, 587–593. doi: 10.1101/lm.042739.116
Ooi, S. K., and Bestor, T. H. (2008). The colorful history of active DNA demethylation. Cell 133, 1145–1148. doi: 10.1016/j.cell.2008.06.009
Penn, N. W., Suwalski, R., O’Riley, C., Bojanowski, K., and Yura, R. (1972). The presence of 5-hydroxymethylcytosine in animal deoxyribonucleic acid. Biochem. J. 126, 781–790. doi: 10.1042/bj1260781
Rai, K., Huggins, I. J., James, S. R., Karpf, A. R., Jones, D. A., and Cairns, B. R. (2008). DNA demethylation in zebrafish involves the coupling of a deaminase, a glycosylase and gadd45. Cell 135, 1201–1212. doi: 10.1016/j.cell.2008.11.042
Raiber, E. A., Beraldi, D., Ficz, G., Burgess, H. E., Branco, M. R., Murat, P., et al. (2012). Genome-wide distribution of 5-formylcytosine in embryonic stem cells is associated with transcription and depends on thymine DNA glycosylase. Genome Biol. 13:R69. doi: 10.1186/gb-2012-13-8-r69
Rangam, G., Schmitz, K. M., Cobb, A. J., and Petersen-Mahrt, S. K. (2012). AID enzymatic activity is inversely proportional to the size of cytosine C5 orbital cloud. PLoS One 7:e43279. doi: 10.1371/journal.pone.0043279
Ratnu, V. S., Wei, W., and Bredy, T. W. (2014). Activation-induced cytidine deaminase regulates activity-dependent BDNF expression in post-mitotic cortical neurons. Eur. J. Neurosci. 40, 3032–3039. doi: 10.1111/ejn.12678
Roubroeks, J. A. Y., Smith, R. G., van den Hove, D. L. A., and Lunnon, K. (2017). Epigenetics and DNA methylomic profiling in Alzheimer’s disease and other neurodegenerative diseases. J. Neurochem. 143, 158–170. doi: 10.1111/jnc.14148
Shen, L., Wu, H., Diep, D., Yamaguchi, S., D’Alessio, A. C., Fung, H. L., et al. (2013). Genome-wide analysis reveals TET- and TDG-dependent 5-methylcytosine oxidation dynamics. Cell 153, 692–706. doi: 10.1016/j.cell.2013.04.002
Singh, A., and Kar, S. K. (2017). How electroconvulsive therapy Works?: understanding the neurobiological mechanisms. Clin. Psychopharmacol. Neurosci. 15, 210–221. doi: 10.9758/cpn.2017.15.3.210
Sultan, F. A., Wang, J., Tront, J., Liebermann, D. A., and Sweatt, J. D. (2012). Genetic deletion of Gadd45b, a regulator of active DNA demethylation, enhances long-term memory and synaptic plasticity. J. Neurosci. 32, 17059–17066. doi: 10.1523/JNEUROSCI.1747-12.2012
Tahiliani, M., Koh, K. P., Shen, Y., Pastor, W. A., Bandukwala, H., Brudno, Y., et al. (2009). Conversion of 5-methylcytosine to 5-hydroxymethylcytosine in mammalian DNA by MLL partner TET1. Science 324, 930–935. doi: 10.1126/science.1170116
Varley, K. E., Gertz, J., Bowling, K. M., Parker, S. L., Reddy, T. E., Pauli-Behn, F., et al. (2013). Dynamic DNA methylation across diverse human cell lines and tissues. Genome Res. 23, 555–567. doi: 10.1101/gr.147942.112
Veldic, M., Caruncho, H. J., Liu, W. S., Davis, J., Satta, R., Grayson, D. R., et al. (2004). DNA-methyltransferase 1 mRNA is selectively overexpressed in telencephalic GABAergic interneurons of schizophrenia brains. Proc. Natl. Acad. Sci. U S A 101, 348–353. doi: 10.1073/pnas.2637013100
Wijesinghe, P., and Bhagwat, A. S. (2012). Efficient deamination of 5-methylcytosines in DNA by human APOBEC3A, but not by AID or APOBEC3G. Nucleic Acids Res. 40, 9206–9217. doi: 10.1093/nar/gks685
Xie, W., Barr, C. L., Kim, A., Yue, F., Lee, A. Y., Eubanks, J., et al. (2012). Base-resolution analysis of sequence and parent-of-origin dependent DNA methylation in the mouse genome. Cell 148, 816–831. doi: 10.1016/j.cell.2011.12.035
Yu, H., Su, Y., Shin, J., Zhong, C., Guo, J. U., Weng, Y. L., et al. (2015). Tet3 regulates synaptic transmission and homeostatic plasticity via DNA oxidation and repair. Nat. Neurosci. 18, 836–843. doi: 10.1038/nn.4008
Zhu, J. K. (2009). Active DNA demethylation mediated by DNA glycosylases. Annu. Rev. Genet. 43, 143–166. doi: 10.1146/annurev-genet-102108-134205
Zhu, B., Zheng, Y., Angliker, H., Schwarz, S., Thiry, S., Siegmann, M., et al. (2000a). 5-methylcytosine DNA glycosylase activity is also present in the human MBD4 (G/T mismatch glycosylase) and in a related avian sequence. Nucleic Acids Res. 28, 4157–4165. doi: 10.1093/nar/28.21.4157
Zhu, B., Zheng, Y., Hess, D., Angliker, H., Schwarz, S., Siegmann, M., et al. (2000b). 5-methylcytosine-DNA glycosylase activity is present in a cloned G/T mismatch DNA glycosylase associated with the chicken embryo DNA demethylation complex. Proc. Natl. Acad. Sci. U S A 97, 5135–5139. doi: 10.1073/pnas.100107597
Zhubi, A., Veldic, M., Puri, N. V., Kadriu, B., Caruncho, H., Loza, I., et al. (2009). An upregulation of DNA-methyltransferase 1 and 3a expressed in telencephalic GABAergic neurons of schizophrenia patients is also detected in peripheral blood lymphocytes. Schizophr. Res. 111, 115–122. doi: 10.1016/j.schres.2009.03.020
Keywords: DNA Methylation, GADD45B, gene expression, synaptic plasticity, TET enzymes, base excision repair (BER), neural disorders, neurons
Citation: Bayraktar G and Kreutz MR (2018) The Role of Activity-Dependent DNA Demethylation in the Adult Brain and in Neurological Disorders. Front. Mol. Neurosci. 11:169. doi: 10.3389/fnmol.2018.00169
Received: 08 March 2018; Accepted: 04 May 2018;
Published: 23 May 2018.
Edited by:
Daniel Ortuño-Sahagún, Universidad de Guadalajara, MexicoReviewed by:
Ana M. M. Oliveira, Universität Heidelberg, GermanyKatja Kobow, Universitätsklinikum Erlangen, Germany
Xiaolu Zhang, Northern Jiangsu People’s Hospital, China
Copyright © 2018 Bayraktar and Kreutz. This is an open-access article distributed under the terms of the Creative Commons Attribution License (CC BY). The use, distribution or reproduction in other forums is permitted, provided the original author(s) and the copyright owner are credited and that the original publication in this journal is cited, in accordance with accepted academic practice. No use, distribution or reproduction is permitted which does not comply with these terms.
*Correspondence: Gonca Bayraktar, gb581@medschl.cam.ac.uk
†Present address: Gonca Bayraktar, UK Dementia Research Institute at the University of Cambridge, Clifford Allbutt Building, Cambridge Biomedical Campus, University of Cambridge, Cambridge, United Kingdom