- 1Department of Medicine, Li Ka Shing Faculty of Medicine, University of Hong Kong, Hong Kong, Hong Kong
- 2Department of Pharmacology, Tongji Medical College, Huazhong University of Science and Technology, Wuhan, China
- 3Xiamen Cardiovascular Hospital, Xiamen University, Xiamen, China
The natural flavone acacetin inhibits several voltage-gated potassium currents in atrial myocytes, and has anti-atrial fibrillation (AF) effect in experimental AF models. The present study investigates whether acacetin inhibits the Ca2+-activated potassium (KCa) currents, including small conductance (SKCa1, SKCa2, and SKCa3), intermediate conductance (IKCa), and large-conductance (BKCa) channels stably expressed in HEK 293 cells. The effects of acacetin on these KCa channels were determined with a whole-cell patch voltage-clamp technique. The results showed that acacetin inhibited the three subtype SKCa channel currents in concentration-dependent manner with IC50 of 12.4 μM for SKCa1, 10.8 μM for SKCa2, and 11.6 μM for SKCa3. Site-directed mutagenesis of SKCa3 channels generated the mutants H490N, S512T, H521N, and A537V. Acacetin inhibited the mutants with IC50 of 118.5 μM for H490N, 275.2 μM for S512T, 15.3 μM for H521N, and 10.6 μM for A537V, suggesting that acacetin interacts with the P-loop helix of SKCa3 channel. However, acacetin at 3–10 μM did not decrease, but induced a slight increase of BKCa (+70 mV) by 8% at 30 μM. These results demonstrate the novel information that acacetin remarkably inhibits SKCa channels, but not IKCa or BKCa channels, which suggests that blockade of SKCa by acacetin likely contributes to its anti-AF property previously observed in experimental AF.
Introduction
Potassium channels are the largest and the most diverse super-family of ion channels in living organisms from bacteria and insects to animals including humans. Among them, Ca2+-activated potassium channels (KCa) comprise many members. They are divided into three subfamilies: big (or large) conductance (BKCa, Slo, or KCa1.1, encoded by KCNMA1), intermediate conductance (IKCa or KCa3.1, encoded by KCNN4), and small conductance channels (SKCa1, SKCa2, and SKCa3 or KCa2.1, KCa2.2, and KCa2.3, encoded by KCNN1, KCNN2, and KCNN3, respectively) (Girault et al., 2012; Gueguinou et al., 2014). The three SKCa channels are expressed in excitable tissues (e.g., neurons, skeletal muscle, adrenal gland, and heart) and also in some non-excitable tissues (e.g., liver, vascular endothelium, cancers, etc.) (Wei et al., 2005). In neurons, apamin-sensitive SKCa current is responsible for afterhyperpolarization (Weatherall et al., 2010) and regulates firing frequency as well as learning and memory (Adelman et al., 2012). In the cardiovascular system, SKCa channels contribute to cardiac repolarization (Xu et al., 2003; Li et al., 2009; Zhang et al., 2014), endothelium-derived hyperpolarization-type arterial dilation in response to increased hemodynamics (Wulff and Kohler, 2013), and also provide negative feedback on sympathetic tone (Taylor et al., 2003). Results from recent studies suggest that SKCa channels play a role in atrial fibrillation (AF) (Diness et al., 2010; Ellinor et al., 2010; Qi et al., 2014; Haugaard et al., 2015), tumor cell migration and metastasis (Chantome et al., 2013), and overactive bladder (Soder et al., 2013). A recent report demonstrated that the SKCa inhibitor apamin may cause ventricular arrhythmias in failing rabbit hearts (Chang et al., 2013); however, blockade of SKCa channels is very effective in anti-AF (Diness et al., 2010; Qi et al., 2014; Haugaard et al., 2015). The development of SK channel blockers has been considered as a new therapeutic strategy in the treatment of AF (Zhang et al., 2015).
We have previously reported that the natural flavone acacetin from the traditional Chinese medicinal herb Xuelianhua (Saussurea involucrata) prolongs the atrial effective refractory period and prevents or terminates the experimentally induced AF in anesthetized dogs without increasing the QT interval (Li et al., 2008; Liu et al., 2016) by inhibiting atrial IKur (ultra-rapidly activating delayed rectifier potassium current) or Kv1.5, IK.ACh (acetylcholine-activated potassium current), and Ito (transient outward potassium current) (Wu et al., 2011, 2013a). The present study investigated the effects of acacetin on SKCa1, SKCa2, SKCa3, IKCa, and BKCa currents in HEK 293 cells stably expressing corresponding channel genes with a conventional whole-cell patch voltage-clamp technique. Our results showed that acacetin inhibited the three subtypes of SKCa channels, but not IKCa and BKCa channels, suggesting that the blockade of SKCa channels may also participate in the anti-AF previously observed in experimental canine models.
Materials and Methods
Cell Line Culture and Gene Transfection
The pCDNA3/rSKCa2 (KCNN2), pCDNA3/hSKCa3 (KCNN3), and pCDNA3/hIKCa (KCNN4) plasmids obtained as generous gifts from Dr. Nicole Schmitt (Department of Biomedical Sciences, University of Copenhagen, Copenhagen, Denmark) were transfected into HEK 293 cells (ATCC, Manassas, VA, United States) using Lipofectamine 2000TM. The HEK 293 cell lines stably expressing the SKCa1, SKCa2, and SKCa3 channels were established as described previously (Wu et al., 2012). The cell lines were maintained in Dulbecco’s modified Eagle’s medium (Invitrogen, Hong Kong, China) supplemented with 10% fetal bovine serum and G418 (400 μg/ml). HEK 293 cell line (Wu et al., 2013c) stably expressing human BKCa (KCNMA1) was also maintained in the same culture conditions. Cells were seeded on glass cover slips for electrophysiological recording.
The primers of SKCa3 mutants were synthesized by the Genome Research Center, the University of Hong Kong (Hong Kong), and the mutants were generated using a QuikChange kit (Stratagene, La Jolla, CA, United States). After confirmed by DNA sequencing, the mutants were transiently expressed in HEK 293 cells in a 35 mm culture dish using Lipofectamine 2000TM (10 μl) with SKCa3 mutant cDNA plasmid (4 μg).
Drugs and Solutions
Acacetin (5,7-dihydroxy-4′-methoxyflavone) was synthesized in the laboratory as described previously in the US patent (Li et al., 2010). The stock solution (100 mM) of acacetin was prepared with dimethyl sulfoxide, aliquoted, and stored at -20°C. Tyrode’s solution used in this study contained (in mM): 140 NaCl, 5.4 KCl, 1 MgCl2, 1.8 CaCl2, 10 HEPES, and 10 glucose (pH was adjusted to 7.3 with NaOH). The pipette solution contained (in mM): 20 KCl, 110 potassium aspartate, 1.0 MgCl2, 10 HEPES, 5 EGTA, 0.1 GTP, 5 sodium phosphocreatine, and 5 Mg-ATP, pH adjusted to 7.2 with KOH (Wu et al., 2013b), in which 300 nM free Ca2+ (calculated using the Cabuf software provided by Dr. G. Droogmans, Department of Physiology, KU Leuven, Leuven, Belgium) was included.
Electrophysiology
The HEK 293 cells on a coverslip were placed into a cell chamber mounted on the stage of an inverted microscope (Olympus, IX70, Japan), and superfused with Tyrode’s solution (2 ml/min). Whole-cell current was recorded with a patch clamp amplifier (EPC-10, HEKA Elektronik, Lambrecht, Germany) as described previously (Wu et al., 2011, 2012, 2013c; Sun et al., 2014). Briefly, glass electrodes (1.2 mm OD) were pulled with a Brown–Flaming puller (Model P-97, Sutter Instrument Co., Novato, CA, United States). Resistance of the glass pipettes was 2–3 MΩ when filled with the pipette solution. Whole-cell configuration was established by a gentle suction after a gigaohm-seal was obtained. Electrical signal was stored on the hard disk of a PC computer. All experiments were performed at room temperature (22–23°C).
Statistical Analysis
The data were expressed as means ± SEM. Unpaired Student’s t-tests were used as appropriate to evaluate the differences between two group means, and ANOVA was used for multiple groups. A value of P < 0.05 was considered to indicate statistical significance.
Results
Effect of Acacetin on SKCa1 Current
The effect of acacetin on SKCa1 current was determined in HEK 293 cells stably expressing human KCNN1. Figure 1A displays the voltage-dependent SKCa1 current recorded with 200-ms voltage steps between -70 and +80 mV from a holding potential of -80 mV in a representative cell. The current was inhibited by 10 μM acacetin (10 min exposure), and the inhibition was partially reversed by washout. Figure 1B displays the current–voltage (I–V) relationships of SKCa1 determined in another typical experiment with a voltage ramp in the absence and presence of acacetin. The I–V relationships of SKCa1 current showed a reversal potential around -70 mV and an inward rectification property, typical SKCa current as described previously (Girault et al., 2011; Wu et al., 2013b). The current was significantly descreased by 10 μM acacetin in bath solution, and the inhibition was partially reversed on washout. Figure 1C illustrates the concentration-dependent inhibition of SKCa1 current (at +80 mV) by acacetin. The concentration–response curve was fitted to a Hill equation to obtain IC50 (the concentration of inhibiting 50% current) value. The IC50 of acacetin for inhibiting SKCa1 at +80 mV was 12.4 μM (Hill co-efficient, 0.8).
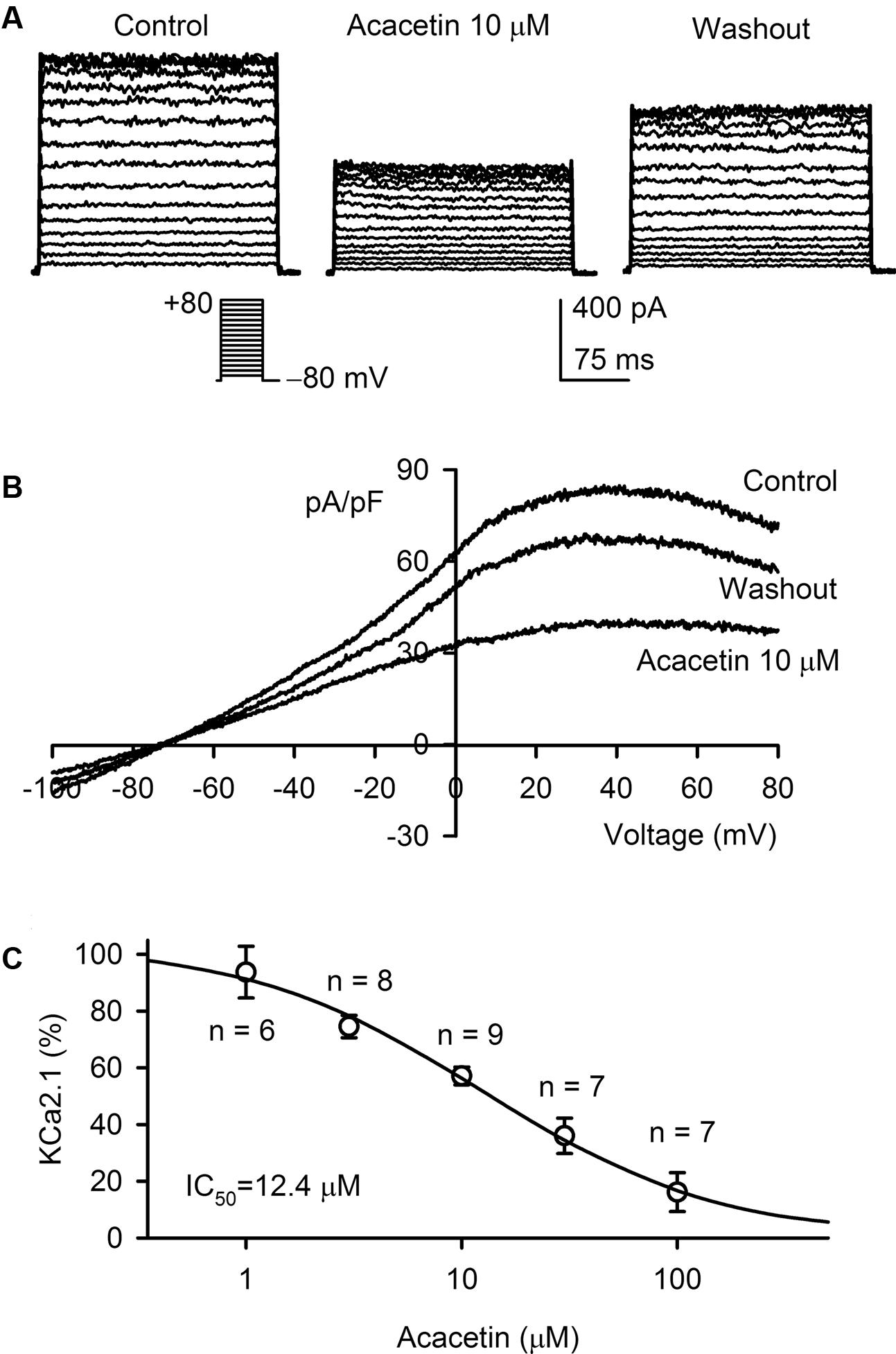
FIGURE 1. Effect of acacetin on SKCa1 channel stably expressed in HEK 293 cells. (A) SKCa1 current was activated in a representative cell expressing human KCNN1 by 200-ms step voltages between –70 and +80 mV from a holding potential of –80 mV in the absence and presence of 10 μM acacetin. (B) Current–voltage (I–V) relationships of SKCa1 current were recorded in a typical experiment with a 3-s voltage ramp from –100 to +80 mV in the absence and presence of 10 μM acacetin. (C) Concentration–response relationship of acacetin for inhibiting SKCa1 current (+80 mV) was fitted to a Hill equation to obtain IC50 value of acacetin.
Effect of Acacetin on SKCa2 Current
The effect of acacetin on SKCa2 current was determined in HEK 293 cell line expressing rat KCNN2. Figure 2A shows the voltage-dependent SKCa2 current in a typical experiment with the voltage protocol as shown in the inset. The current was significantly decreased by 10 μM acacetin (10 min exposure) at all testing potentials, and the inhibition was partially reversed by washout. I–V relationships of SKCa2 current determined by a ramp voltage protocol also showed an inward rectification. Inward and outward components of the current were decreased by 10 μM acacetin, and the inhibition was partially recovered on drug washout (Figure 2B). Figure 2C illustrates the concentration–response relationship of acacetin for inhibiting SKCa2 current (at +80 mV). The curve was fitted to a Hill equation. The IC50 of acacetin for inhibiting SKCa2 current was 10.8 μM (Hill coefficient, 0.8).
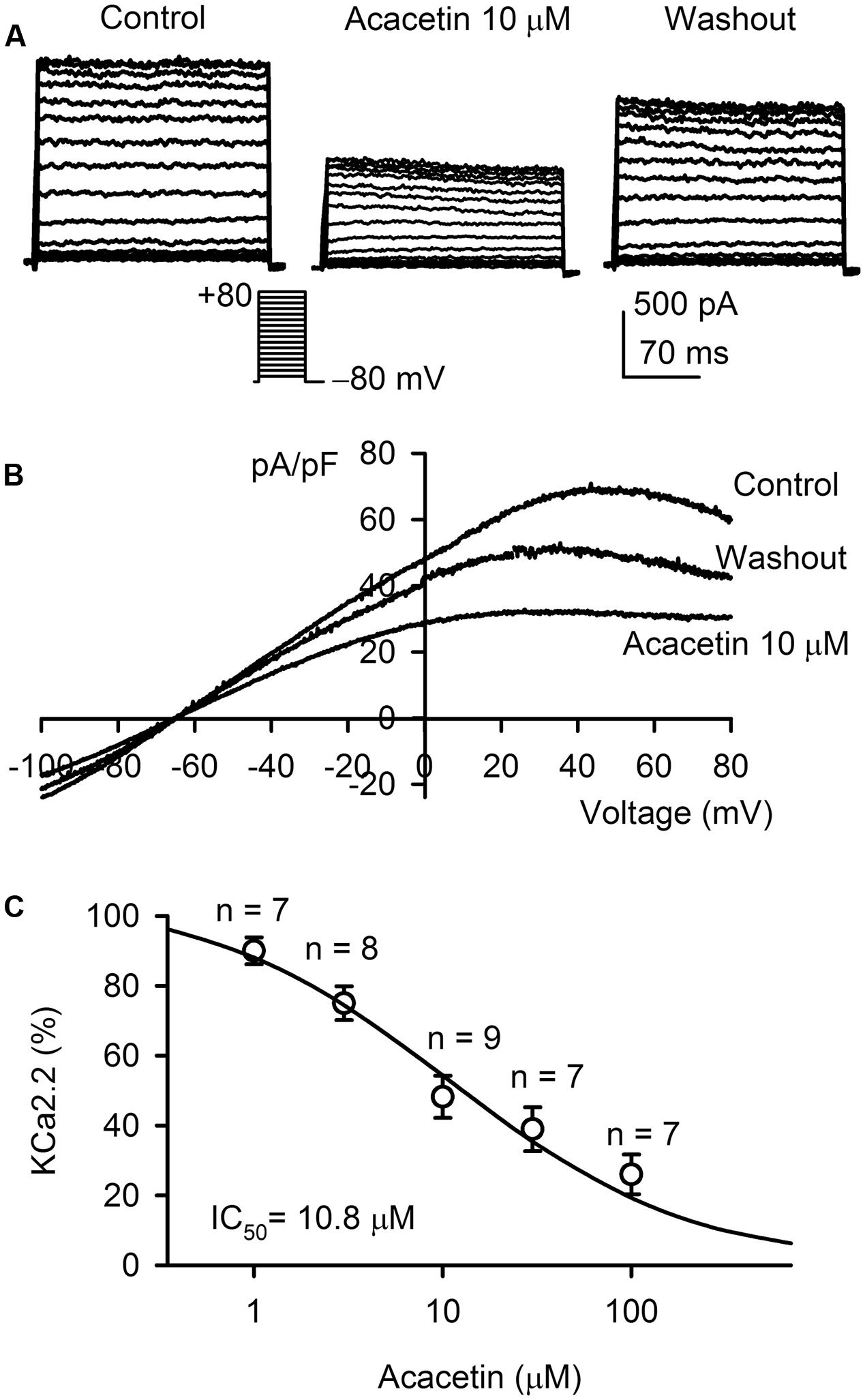
FIGURE 2. Effect of acacetin on SKCa2 channel stably expressed in HEK 293 cells. (A) SKCa2 current was activated in a representative cell expressing rat KCNN2 by 200-ms step voltages between –70 and +80 mV from a holding potential of –80 mV in the absence and presence of 10 μM acacetin. (B) Current–voltage (I–V) relationships of SKCa2 current were recorded in a typical experiment with a 3-s voltage ramp from –100 to +80 mV in the absence and presence of 10 μM acacetin. (C) Concentration–response relationship of acacetin for inhibiting SKCa2 current (+80 mV) was fitted to a Hill equation to obtain IC50 value of acacetin.
Inhibition of SKCa3 Current by Acacetin
The inhibitory effect of acacetin on SKCa3 was determined in HEK 293 cell line expressing human KCNN3 gene. The voltage-dependent step SKCa3 current was determined with the voltage protocol as shown in the inset in a typical experiment (Figure 3A). SKCa3 current at all test potentials was inhibited by 10 μM acacetin with 10 min incubation, and the inhibition partially recovered on washout for 10 min. I–V relationships of SKCa3 current was determined with a ramp voltage protocol in another cell (Figure 3B) before and after application of acacetin. The current also displays an inward rectification and was reversibly decreased by 10 μM acacetin. The concentration–response curve of acacetin for inhibiting SKCa 3 current was fitted to a Hill equation (Figure 3C). The IC50 of acacetin for inhibiting SKCa3 was 11.6 μM (with a Hill coefficient of 0.8).
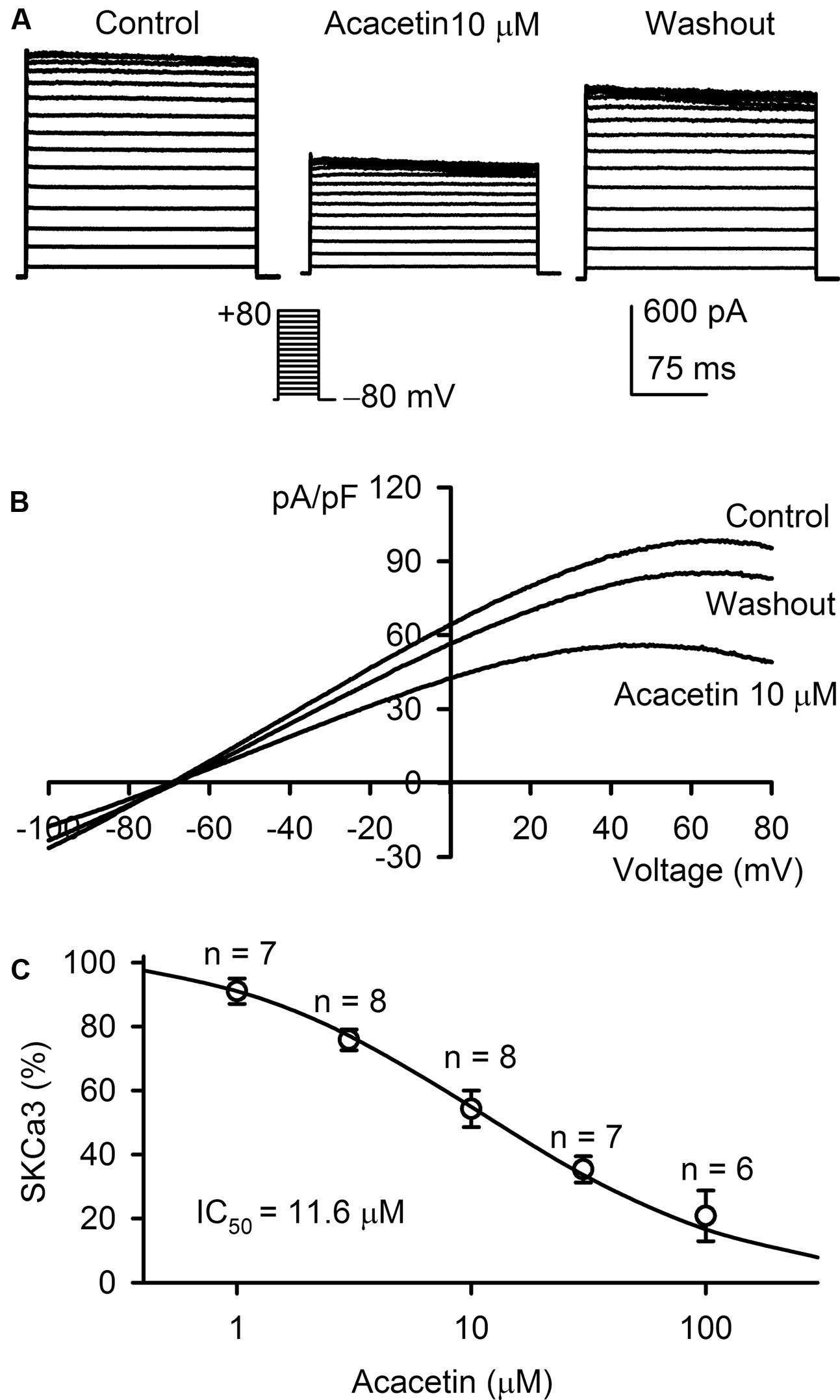
FIGURE 3. Effect of acacetin on SKCa3 channel stably expressed in HEK 293 cells. (A) SKCa3 current was activated in a representative cell expressing human KCNN3 by 200-ms step voltages between –70 and +80 mV from a holding potential of –80 mV in the absence and presence of 10 μM acacetin. (B) Current–voltage (I–V) relationships of SKCa3 current were recorded in a typical experiment with a 3-s voltage ramp from –100 to +80 mV in the absence and presence of 10 μM acacetin. (C) Concentration–response relationship of acacetin for inhibiting SKCa3 current (+80 mV) was fitted to a Hill equation to obtain IC50 value of acacetin.
Molecular Determinant of Acacetin for Inhibiting SKCa Channels
The potential molecular determinant of acacetin for inhibiting SKCa channels was investigated using SKCa3 mutants, H490N, S512T, H521N, and A537V in P-loop helix and S6, generated by site-directed mutagenesis as described previously (Wu et al., 2013a,b). Figure 4A illustrates the I–V relationships of wild type (WT) SKCa current and mutant currents recorded in representative cells expressing WT SKCa3 or the mutant H490N, S512T, or H521N with a voltage ramp protocol before (control) and after 10 μM acacetin. The inhibitory effect of acacetin for the mutant H490N and S512T currents was clearly reduced, compared with WT SKCa3 current. Figure 4B illustrates the percent values of current inhibition by 10 μM acacetin for WT SKCa3, and the mutants H490N, S512T, H521N, and A537V currents at +80 mV. Acacetin at 10 μM decreased the current by 45.7 ± 4.1% for WT SKCa1 current (n = 11), 21.9 ± 4.5% for H490N current (n = 7, P < 0.01 vs. WT), 17.9 ± 3.9% for S512T current (n = 7, P < 0.01 vs. WT), 48.8 ± 3.5% for H521N current (n = 7, P > 0.05 vs. WT), and 40.8 ± 7.6% for A537V current (n = 6, P > 0.05 vs. WT), respectively.
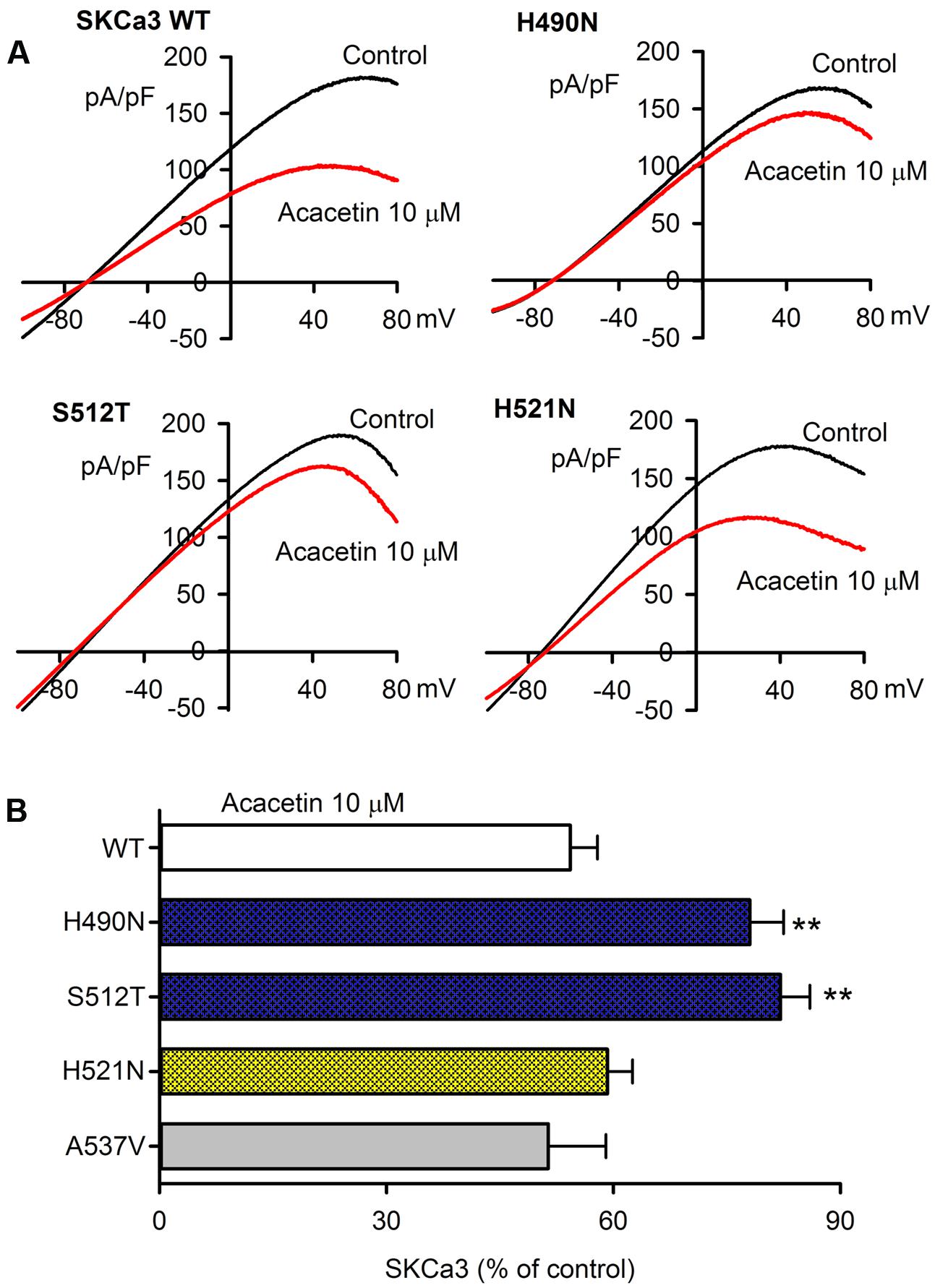
FIGURE 4. Effect of acacetin on WT and mutant SKCa3 channel currents expressed in HEK 293 cells. (A) Current–voltage (I–V) relationships of SKCa3 WT current, H490N, S512T, H521N were recorded in typical experiments with a 3-s voltage ramp from –100 to +80 mV in the absence and presence of 10 μM acacetin. (B) Percent values of 10 μM acacetin for inhibiting SKCa3 WT current (n = 11, H490N current (n = 7, ∗∗P < 0.01 vs. WT), S512T current (n = 7, ∗∗P < 0.01 vs. WT), H521N current (n = 7, P > 0.05 vs. WT), or A537V current (n = 6) at +80 mV.
Figure 5A displays the concentration–response relationships of acacetin for inhibiting WT SKCa3 current, H490N current, S512T current, H521N current, and A537V current at +80 mV. The concentration-dependent inhibition curves were fitted to a Hill equation. The IC50 of acacetin was 11.6 μM for WT SKCa3 current, 118.5 μM for H490N current, 275.2 μM for S512T current, 15.3 μM for H521N current, and 10.6 μM for A537V current, respectively. The efficacy of acacetin for inhibiting H490N current and S512T current was dramatically reduced, which suggests that acacetin blocks SKCa3 channel by interacting with H490 and S512 in the P-loop helix of the channel (Figure 5B).
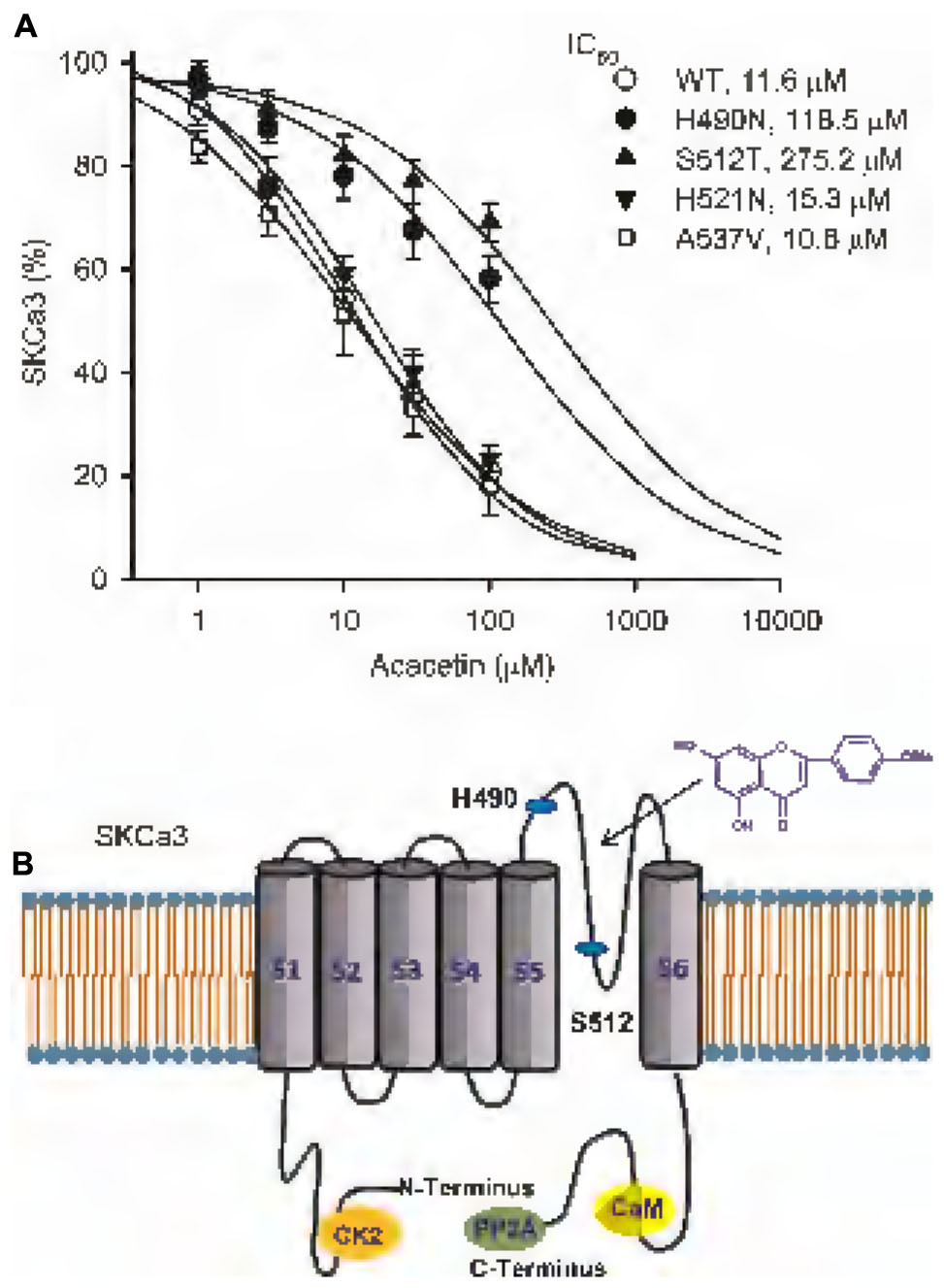
FIGURE 5. IC50 values of acacetin for inhibiting SKCa3 WT, H490N, S512T, H521N, or 537V current in HEK 293 cells. (A) Concentration–response relationship curves of acacetin for inhibiting SKCa3 WT current (+80 mV), H490N, S512T, H521N, or 537V (n = 6–9 for each concentration) was fitted to a Hill equation to obtain IC50 values of acacetin. (B) Schematic graph showing the putative binding sites of acacetin at H490, S512, and also H521 in the P-loop helix of human SKCa3 channels.
Effect of Acacetin on IKCa Current
The potential effect of acacetin on IKCa was determined in HEK 293 cell line expressing human KCNN4. The voltage-dependent IKCa current (Figure 6A) was recorded with the step voltages as shown in the inset in a typical experiment before and after application of acacetin. Acacetin (10 and 30 μM) slightly decreased the current, and the inhibition was partially recovered on washout. Similar results were observed for the I–V relationships of the current recorded with a voltage ramp in another representative cell (Figure 6B). IKCa shows a linear I–V relationship, similar to those previously recorded in HEK 293 cell line expressing IKCa (Girault et al., 2011). Figure 6C shows that acacetin (10 and 30 μM) decreased IKCa (+70 mV) to 95.0 ± 4.5% (n = 7, P > 0.05) and 89.3 ± 5.5% of control (n = 7, P < 0.05 vs. control, 0 μM), respectively. These results suggest that acacetin has a slight inhibition of IKCa current.
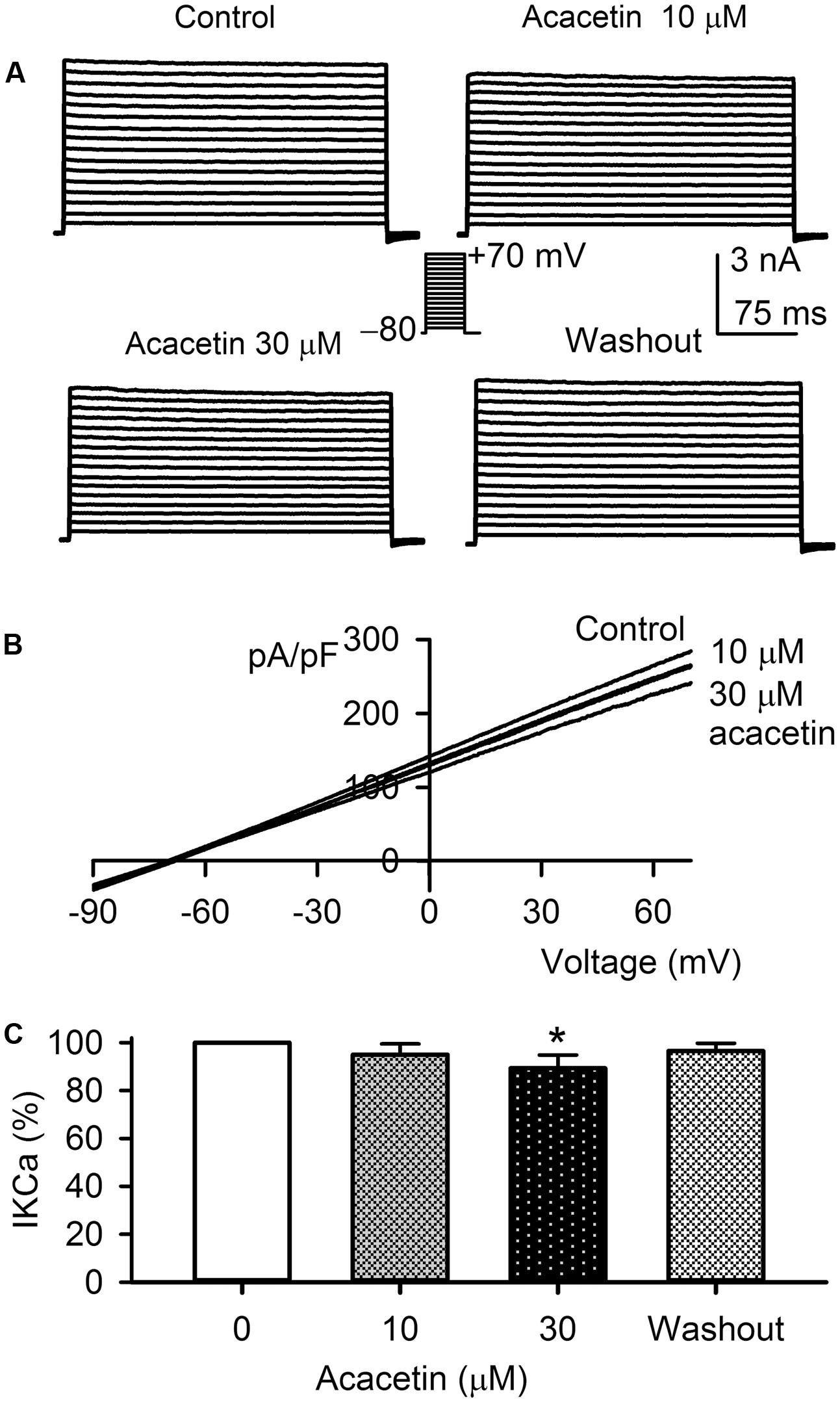
FIGURE 6. Effect of acacetin on IKCa channel stably expressed in HEK 293 cells. (A) IKCa current was activated in a representative cell expressing human KCNN4 by 200-ms step voltages between –70 and +70 mV from a holding potential of –80 mV in the absence and presence of 10 or 30 μM acacetin. (B) Current–voltage (I–V) relationships of IKCa current were recorded in a typical experiment with a 3-s voltage ramp from –90 to +70 mV in the absence and presence of 10 and 30 μM acacetin. (C) Percent values of acacetin (10 or 30 μM) for inhibiting IKCa current (+70 mV, n = 7, ∗P < 0.05 vs. 0 μM acacetin).
Effect of Acacetin on BKCa Current
The effect of acacetin on BKCa current was examined in HEK 293 cell line expressing human KCNMA1 gene. Voltage-dependent BKCa current was recorded with the step voltage protocol as shown in the inset in a representative cell before and after application of acacetin (Figure 7A). Acacetin had no effect on BKCa at 3 and 10 μM, whereas it slightly increased the current at 30 μM. I–V relationships (Figure 7B) of BKCa current determined with a voltage ramp showed a similar response to acacetin. Acacetin did not affect the current at 10 μM, but slightly increased the outward component of BKCa current at 30 μM. The BKCa inhibitor paxilline (1 μM) almost fully suppressed the current. The percent values of BKCa current at +70 mV illustrated in Figure 7C show that no significant effect of acacetin was observed at 3 and 10 μM, whereas 30 μM acacetin increased the current to 108.1 ± 5.7% of control (n = 7, P < 0.05 vs. control). These results suggest that acacetin may stimulate BKCa channel at high concentration of 30 μM.
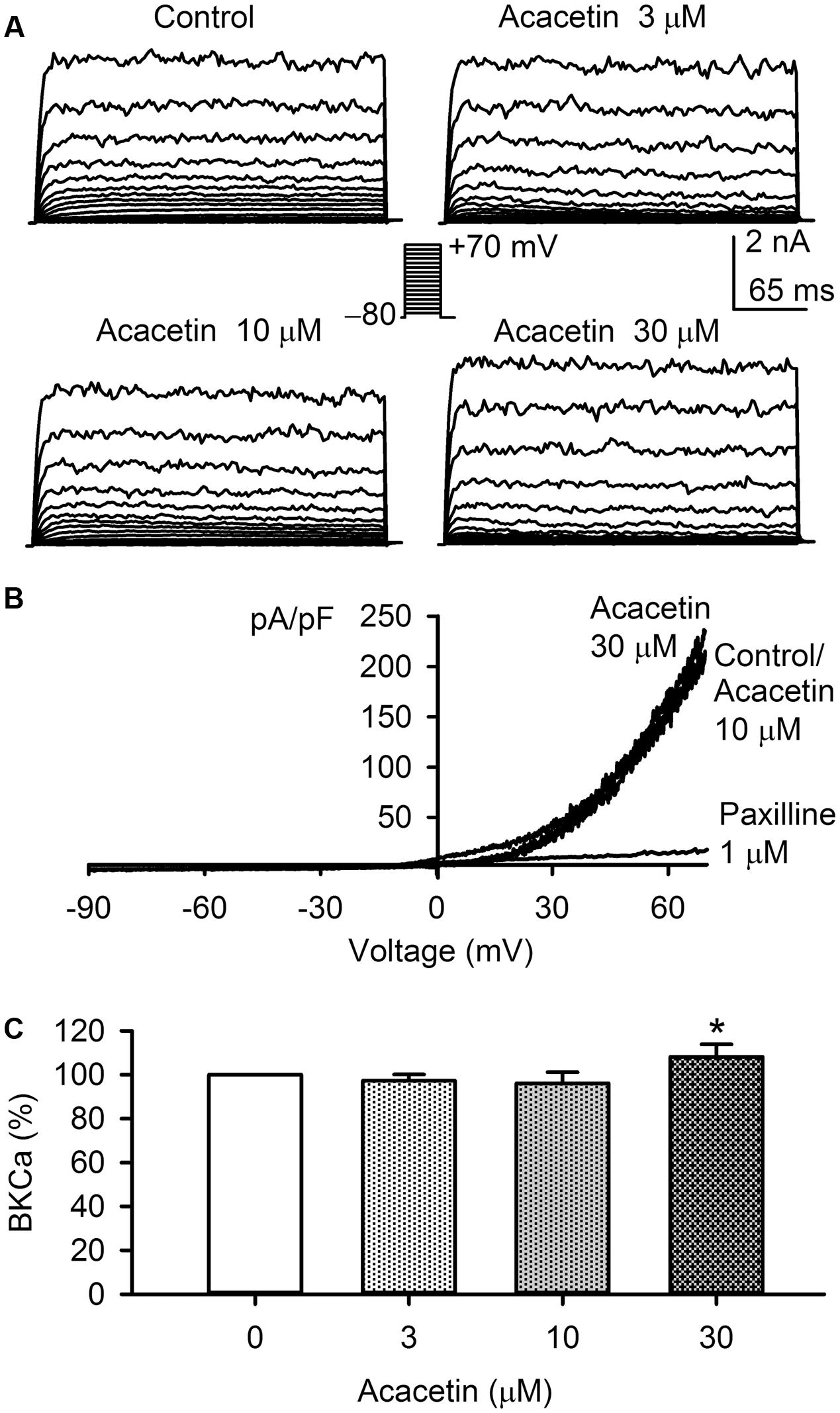
FIGURE 7. Effect of acacetin on BKCa channel stably expressed in HEK 293 cells. (A) BKCa current was activated in a representative cell expressing human KCNMA1 by 200-ms step voltages between –70 and +70 mV from a holding potential of –80 mV in the absence and presence of 3, 10, or 30 μM acacetin. (B) Current–voltage (I–V) relationships of SKCa3 current were recorded in a typical experiment with a 3-s voltage ramp from –90 to +70 mV in the absence and presence of 10 and 30 μM acacetin. (C) Percent values of acacetin (3, 10, or 30 μM) for increasing BKCa current (+70 mV, n = 7, ∗P < 0.05 vs. 0 μM acacetin).
Discussion
The present study provides the novel information that the natural flavone acacetin blocks the three SKCa channel subtypes: SKCa1, SKCa2, and SKCa3, stably expressed in HEK 293 cells with similar efficacy. The IC50 values of acacetin for inhibiting SKCa1, SKCa2, and SKCa3 are 12.4, 10.8, and 11.6 μM, respectively. Point mutagenesis of SKCa3 channel reveals that acacetin mainly interacts with H490 and S512 in the P-loop helix of the channel. However, acacetin at a high concentration of 30 μM induces only a small decrease in IKCa channel and a small increase in BKCa channel stably expressed in HEK 293 cells. The very limited effect of acacetin on IKCa channel is similar to that reported previously for other SKCa channel blockers (Girault et al., 2011).
An earlier study demonstrated that SKCa channels were expressed in rat skeletal muscles, and sensitive to blocking by apamin (Blatz and Magleby, 1986). Then, the sequence of the transmembrane segments of SKCa1, SKCa2, and SKCa3 are found 80–90% identical (Kohler et al., 1996). However, the three subunits have different sensitivity to blocking by apamin (SKCa2 > SKCa1 > SKCa3), and are highly conserved among mammalian species, and are identified in many organisms from Drosophila to humans (Adelman et al., 2012). SKCa subunits assemble to form homomeric (Kohler et al., 1996) or heteromeric (Tuteja et al., 2010) tetramers. SKCa channels are identified in human and mouse atrial myocytes (Tuteja et al., 2005; Skibsbye et al., 2014), neurons (Church et al., 2015), and tumor cells (Girault et al., 2011, 2012; Gueguinou et al., 2014).
In the heart, activation of SKCa channel may be antiarrhythmic or proarrhythmic, depending on the myocardial pathophysiological conditions (Chang and Chen, 2015). Chua et al. (2011) reported that the SKCa channel current was heterogeneously upregulated in failing rabbit ventricles and SKCa blocker apamin suppressed post-shock shortening of action potential duration in the failing hearts with ventricular fibrillation. On the other hand, apamin induced ventricular arrhythmias in slowly paced failing rabbit ventricles (Chang et al., 2013). The proarrhythmic effect was also observed with apamin in isolated normal canine left atrium (Hsueh et al., 2013).
However, the results from other groups demonstrated that blockade of SKCa channels prolongs atrial effective refractory period, and SKCa channels are therefore considered as a promising therapeutic target in the treatment of AF (Diness et al., 2010, 2011; Qi et al., 2014; Haugaard et al., 2015). Several SKCa channel blockers, e.g., NS8593, UCL1684, N-(pyridin-2-yl)-4-(pyridin-2-yl)thiazol-2-amine (ICA) and apamin, have been used for anti-AF studies (Diness et al., 2010, 2011, 2015). In perfused guinea pig hearts, NS8593, UCL1684, and ICA effectively terminated AF induced with a combination of acetylcholine with electric stimulation (Diness et al., 2010). Injection of NS8593, UCL1684 or apamin reduced the duration of pacing-induced AF in vivo rat model (Skibsbye et al., 2011). UCL1684 and NS8593 had significant anti-AF effect in a rat paroxysmal AF with hypertension-induced atrial remodeling (Diness et al., 2011). Interestingly, in large animals such as dogs (Qi et al., 2014) and horses (Haugaard et al., 2015), intravenous administration of NS8593 terminated all induced AF episodes, increased atrial effective refractory period, and decreased AF duration and vulnerability without QTc interval prolongation, suggesting that SKCa channel blockers can be considered as promising anti-AF drugs. Moreover, recent studies showed that acute myocardial infarction might activate SKCa channels, and apamin, UCL-1684 or ICA reduced ventricular burden arrhythmia by prolonging ventricular action potential duration and effective refractory period in rats with acute myocardial infarction (Gui et al., 2013; Hundahl et al., 2017).
In this study, we demonstrated that acacetin inhibited SKCa1, SKCa2, and SKCa3 channels in HEK 293 cell line expressing the corresponding genes. The blockade of SKCa channels by acacetin likely also contributes to the anti-AF effect observed in canine models in addition to blocking IKur/Kv1.5, Ito/Kv4.3, and IK.ACh (Li et al., 2008; Wu et al., 2011, 2013a). These studies suggest that acacetin blocks multiple atrial-selective channels, and would be more effective in anti-AF than the blocker that specifically inhibits one type of atrial channel. However, whether acacetin, as apamin and other SKCa blockers, is effective in improving learning and memory (Adelman et al., 2012) remains to be studied in the future. Moreover, additional studies are required for clarifying whether the SKCa blocking effect of acacetin is related to its anti-cancer effect (Salimi et al., 2016; Zeng et al., 2017).
In our previous reports, we demonstrated that acacetin blocked Kv1.5 channel by binding to both its resting and open states by interacting with V505, I508, and V512 within the S6 domain (Wu et al., 2011), and inhibited the closed channel and blocked the open state of Kv4.3 by binding to both P-loop selectivity filter and S6 domain (Wu et al., 2013a). In the present study, we found that H490 and S512, but not H521, of P-loop helix are the binding sites of acacetin for blocking SKCa3 channel. The pore blocking of SKCa3 by acacetin is applicable to SKCa1 and SKCa2, because SKCa1, SKCa2, and SKCa3 share the same sequence in the range of pore helix1. This differs from the molecular determinants of acacetin for blocking Kv1.5 or Kv4.3 channel. On the other hand, the acacetin blockade of SKCa3 channel is different from the organic SKCa blocker NS8593 and the archetypical peptide SKCa blocker apamin. NS8593 interacts with S507 of P-loop helix and A532 of S6 domain (Jenkins et al., 2011), while apamin binds to a residue of S3–S4 extracellular loop of outside pore of the channel to produce a high-sensitivity block without selectivity filter contact (Weatherall et al., 2011). While the binding sites of various SKCa blockers differ, it is important to develop these potential blockers into feasible drug candidates for future clinical application. A water soluble prodrug of acacetin has been developed, which can be intravenously administered for future clinical application (Liu et al., 2016).
Acacetin showed increased BKCa current at concentration of 30 μM. Although the concentration for activating BKCa channel is greater than those of blocking IKur/Kv1.5, IK.ACh, Ito/Kv4.3, and also SKCa channels; this effect may account in part for the vascular dilation reported in a previous study (Calderone et al., 2004).
A limitation of the present study was that all the experiments were conducted only in HEK 293 line expressing SKCa1, SKCa2, or SKCa3 channels and lack of data from native cardiomyocytes. However, this does not affect the conclusion that acacetin blocks SKCa channels. Future effort is required to obtain the data for the effect of acacetin on SKCa current in native cardiomyocytes from an animal species whose heart has no or less expression of IKur/Kv1.5 and Ito/Kv4.3, because acacetin also inhibits these currents in native human atrial myocytes (Li et al., 2008).
Collectively, the present study demonstrates for the first time that acacetin is a SKCa channel blocker and inhibits three subtypes of the SKCa channels stably expressed in HEK 293 cells. The SKCa channel blocking effect may be involved in its anti-AF property previously observed in experimentally induced AF in dogs.
Author Contributions
K-HC, M-WJ, G-SX, YW, and G-RL conceived and designed the project. K-HC, HL, and H-YS conducted the experiments. K-HC, HL, H-YS, and G-RL analyzed the data. K-HC and G-RL prepared the manuscript. All authors read and approved the manuscript.
Conflict of Interest Statement
The authors declare that the research was conducted in the absence of any commercial or financial relationships that could be construed as a potential conflict of interest.
Acknowledgments
This work was supported in part by a grant (ITS/339/09) from Innovation and Technology Commission of the Hong Kong SAR Government, China, a Seeding Fund from the University of Hong Kong, and a Key Cardiovascular Laboratory Fund (3502Z20150050) from Department of Xiamen Science and Technology, Xiamen, China
Abbreviations
AF, atrial fibrillation; BKCa, big/large conductance Ca2+-activated potassium channels; IKCa, intermediate conductance Ca2+-activated potassium channels; KCa, Ca2+-activated potassium channel; SKCa, small conductance Ca2+-activated potassium channels.
Footnotes
References
Adelman, J. P., Maylie, J., and Sah, P. (2012). Small-conductance Ca2+-activated K+ channels: form and function. Ann. Rev. Physiol. 74, 245–269. doi: 10.1146/annurev-physiol-020911-153336
Blatz, A. L., and Magleby, K. L. (1986). Single apamin-blocked Ca-activated K+ channels of small conductance in cultured rat skeletal muscle. Nature 323, 718–720. doi: 10.1038/323718a0
Calderone, V., Chericoni, S., Martinelli, C., Testai, L., Nardi, A., Morelli, I., et al. (2004). Vasorelaxing effects of flavonoids: investigation on the possible involvement of potassium channels. Naunyn Schmiedebergs Arch. Pharmacol. 370, 290–298.
Chang, P. C., and Chen, P. S. (2015). SK channels and ventricular arrhythmias in heart failure. Trends Cardiovasc. Med. 25, 508–514. doi: 10.1016/j.tcm.2015.01.010
Chang, P. C., Hsieh, Y. C., Hsueh, C. H., Weiss, J. N., Lin, S. F., and Chen, P. S. (2013). Apamin induces early afterdepolarizations and torsades de pointes ventricular arrhythmia from failing rabbit ventricles exhibiting secondary rises in intracellular calcium. Heart Rhythm. 10, 1516–1524. doi: 10.1016/j.hrthm.2013.07.003
Chantome, A., Potier-Cartereau, M., Clarysse, L., Fromont, G., Marionneau-Lambot, S., Gueguinou, M., et al. (2013). Pivotal role of the lipid Raft SK3-Orai1 complex in human cancer cell migration and bone metastases. Cancer Res. 73, 4852–4861. doi: 10.1158/0008-5472.CAN-12-4572
Chua, S. K., Chang, P. C., Maruyama, M., Turker, I., Shinohara, T., Shen, M. J., et al. (2011). Small-conductance calcium-activated potassium channel and recurrent ventricular fibrillation in failing rabbit ventricles. Circ. Res. 108, 971–979. doi: 10.1161/CIRCRESAHA.110.238386
Church, T. W., Weatherall, K. L., Correa, S. A., Prole, D. L., Brown, J. T., and Marrion, N. V. (2015). Preferential assembly of heteromeric small conductance calcium-activated potassium channels. Eur. J. Neurosci. 41, 305–315. doi: 10.1111/ejn.12789
Diness, J. G., Bentzen, B. H., Sorensen, U. S., and Grunnet, M. (2015). Role of calcium-activated potassium channels in atrial fibrillation pathophysiology and therapy. J. Cardiovasc. Pharmacol. 66, 441–448. doi: 10.1097/FJC.0000000000000249
Diness, J. G., Skibsbye, L., Jespersen, T., Bartels, E. D., Sorensen, U. S., Hansen, R. S., et al. (2011). Effects on atrial fibrillation in aged hypertensive rats by Ca2+-activated K+ channel inhibition. Hypertension 57, 1129–1135. doi: 10.1161/HYPERTENSIONAHA.111.170613
Diness, J. G., Sorensen, U. S., Nissen, J. D., Al-Shahib, B., Jespersen, T., Grunnet, M., et al. (2010). Inhibition of small-conductance Ca2+-activated K+ channels terminates and protects against atrial fibrillation. Circ. Arrhythm. Electrophysiol. 3, 380–390. doi: 10.1161/CIRCEP.110.957407
Ellinor, P. T., Lunetta, K. L., Glazer, N. L., Pfeufer, A., Alonso, A., Chung, M. K., et al. (2010). Common variants in KCNN3 are associated with lone atrial fibrillation. Nat. Genet. 42, 240–244. doi: 10.1038/ng.537
Girault, A., Haelters, J. P., Potier-Cartereau, M., Chantome, A., Jaffres, P. A., Bougnoux, P., et al. (2012). Targeting SKCa channels in cancer: potential new therapeutic approaches. Curr. Med. Chem. 19, 697–713. doi: 10.2174/092986712798992039
Girault, A., Haelters, J. P., Potier-Cartereau, M., Chantome, A., Pinault, M., Marionneau-Lambot, S., et al. (2011). New alkyl-lipid blockers of SK3 channels reduce cancer cell migration and occurrence of metastasis. Curr. Cancer Drug Targets 11, 1111–1125. doi: 10.2174/156800911798073069
Gueguinou, M., Chantome, A., Fromont, G., Bougnoux, P., Vandier, C., and Potier-Cartereau, M. (2014). KCa and Ca2+ channels: the complex thought. Biochim. Biophys. Acta 1843, 2322–2333. doi: 10.1016/j.bbamcr.2014.02.019
Gui, L., Bao, Z., Jia, Y., Qin, X., Cheng, Z. J., Zhu, J., et al. (2013). Ventricular tachyarrhythmias in rats with acute myocardial infarction involves activation of small-conductance Ca2+-activated K+ channels. Am. J. Physiol. Heart Circ. Physiol. 304, H118–H130. doi: 10.1152/ajpheart.00820.2011
Haugaard, M. M., Hesselkilde, E. Z., Pehrson, S., Carstensen, H., Flethoj, M., Praestegaard, K. F., et al. (2015). Pharmacologic inhibition of small-conductance calcium-activated potassium (SK) channels by NS8593 reveals atrial antiarrhythmic potential in horses. Heart Rhythm 12, 825–835. doi: 10.1016/j.hrthm.2014.12.028
Hsueh, C. H., Chang, P. C., Hsieh, Y. C., Reher, T., Chen, P. S., and Lin, S. F. (2013). Proarrhythmic effect of blocking the small conductance calcium activated potassium channel in isolated canine left atrium. Heart Rhythm 10, 891–898. doi: 10.1016/j.hrthm.2013.01.033
Hundahl, L. A., Sattler, S. M., Skibsbye, L., Diness, J. G., Tfelt-Hansen, J., and Jespersen, T. (2017). Pharmacological blockade of small conductance Ca2+-activated K+ channels by ICA reduces arrhythmic load in rats with acute myocardial infarction. Pflugers Arch. 469, 739–750. doi: 10.1007/s00424-017-1962-6
Jenkins, D. P., Strobaek, D., Hougaard, C., Jensen, M. L., Hummel, R., Sorensen, U. S., et al. (2011). Negative gating modulation by (R)-N-(benzimidazol-2-yl)-1,2,3,4- tetrahydro-1-naphthylamine (NS8593) depends on residues in the inner pore vestibule: pharmacological evidence of deep-pore gating of KCa2 channels. Mol. Pharmacol. 79, 899–909. doi: 10.1124/mol.110.069807
Kohler, M., Hirschberg, B., Bond, C. T., Kinzie, J. M., Marrion, N. V., Maylie, J., et al. (1996). Small-conductance, calcium-activated potassium channels from mammalian brain. Science 273, 1709–1714. doi: 10.1126/science.273.5282.1709
Li, G. R., Lau, C. P., Qin, G. W., and Wang, H. B. (2010). Use of Acacetin and Related Compounds as Potassium Channel Inhibitors. Available at: http://www.freepatentsonline.com/y2010/0331271.html
Li, G. R., Wang, H. B., Qin, G. W., Jin, M. W., Tang, Q., Sun, H. Y., et al. (2008). Acacetin, a natural flavone, selectively inhibits human atrial repolarization potassium currents and prevents atrial fibrillation in dogs. Circulation 117, 2449–2457. doi: 10.1161/CIRCULATIONAHA.108.769554
Li, N., Timofeyev, V., Tuteja, D., Xu, D., Lu, L., Zhang, Q., et al. (2009). Ablation of a Ca2+-activated K+ channel (SK2 channel) results in action potential prolongation in atrial myocytes and atrial fibrillation. J. Physiol. 587, 1087–1100. doi: 10.1113/jphysiol.2008.167718
Liu, H., Wang, Y. J., Yang, L., Zhou, M., Jin, M. W., Xiao, G. S., et al. (2016). Synthesis of a highly water-soluble acacetin prodrug for treating experimental atrial fibrillation in beagle dogs. Sci. Rep. 6:25743. doi: 10.1038/srep25743
Qi, X. Y., Diness, J. G., Brundel, B. J., Zhou, X. B., Naud, P., Wu, C. T., et al. (2014). Role of small-conductance calcium-activated potassium channels in atrial electrophysiology and fibrillation in the dog. Circulation 129, 430–440. doi: 10.1161/CIRCULATIONAHA.113.003019
Salimi, A., Roudkenar, M. H., Sadeghi, L., Mohseni, A., Seydi, E., Pirahmadi, N., et al. (2016). Selective anticancer activity of acacetin against chronic lymphocytic leukemia using both in vivo and in vitro methods: key role of oxidative stress and cancerous mitochondria. Nutr. Cancer 68, 1404–1416. doi: 10.1080/01635581.2016.1235717
Skibsbye, L., Diness, J. G., Sorensen, U. S., Hansen, R. S., and Grunnet, M. (2011). The duration of pacing-induced atrial fibrillation is reduced in vivo by inhibition of small conductance Ca2+-activated K+ channels. J. Cardiovasc. Pharmacol. 57, 672–681. doi: 10.1097/FJC.0b013e318217943d
Skibsbye, L., Poulet, C., Diness, J. G., Bentzen, B. H., Yuan, L., Kappert, U., et al. (2014). Small-conductance calcium-activated potassium (SK) channels contribute to action potential repolarization in human atria. Cardiovasc. Res. 103, 156–167. doi: 10.1093/cvr/cvu121
Soder, R. P., Parajuli, S. P., Hristov, K. L., Rovner, E. S., and Petkov, G. V. (2013). SK channel-selective opening by SKA-31 induces hyperpolarization and decreases contractility in human urinary bladder smooth muscle. Am. J. Physiol. Regul. Integr. Comp. Physiol. 304, R155–R163. doi: 10.1152/ajpregu.00363.2012
Sun, H. Y., Xiao, G. S., Wang, Y., and Li, G. R. (2014). Ionic mechanism underlying distinctive excitability in atrium and ventricle of the heart. Sheng Li Xue Bao 66, 85–95.
Taylor, M. S., Bonev, A. D., Gross, T. P., Eckman, D. M., Brayden, J. E., Bond, C. T., et al. (2003). Altered expression of small-conductance Ca2+-activated K+ (SK3) channels modulates arterial tone and blood pressure. Circ. Res. 93, 124–131. doi: 10.1161/01.RES.0000081980.63146.69
Tuteja, D., Rafizadeh, S., Timofeyev, V., Wang, S., Zhang, Z., Li, N., et al. (2010). Cardiac small conductance Ca2+-activated K+ channel subunits form heteromultimers via the coiled-coil domains in the C termini of the channels. Circ. Res. 107, 851–859. doi: 10.1161/CIRCRESAHA.109.215269
Tuteja, D., Xu, D., Timofeyev, V., Lu, L., Sharma, D., Zhang, Z., et al. (2005). Differential expression of small-conductance Ca2+-activated K+ channels SK1, SK2, and SK3 in mouse atrial and ventricular myocytes. Am. J. Physiol. Heart Circ. Physiol. 289, H2714–H2723. doi: 10.1152/ajpheart.00534.2005
Weatherall, K. L., Goodchild, S. J., Jane, D. E., and Marrion, N. V. (2010). Small conductance calcium-activated potassium channels: from structure to function. Prog. Neurobiol. 91, 242–255. doi: 10.1016/j.pneurobio.2010.03.002
Weatherall, K. L., Seutin, V., Liegeois, J. F., and Marrion, N. V. (2011). Crucial role of a shared extracellular loop in apamin sensitivity and maintenance of pore shape of small-conductance calcium-activated potassium (SK) channels. Proc. Natl. Acad. Sci. U.S.A. 108, 18494–18499. doi: 10.1073/pnas.1110724108
Wei, A. D., Gutman, G. A., Aldrich, R., Chandy, K. G., Grissmer, S., and Wulff, H. (2005). International Union of Pharmacology. LII. Nomenclature and molecular relationships of calcium-activated potassium channels. Pharmacol. Rev. 57, 463–472. doi: 10.1124/pr.57.4.9
Wu, H. J., Sun, H. Y., Wu, W., Zhang, Y. H., Qin, G. W., and Li, G. R. (2013a). Properties and molecular determinants of the natural flavone acacetin for blocking hKv4.3 channels. PLOS ONE 8:e57864. doi: 10.1371/journal.pone.0057864
Wu, H. J., Wu, W., Sun, H. Y., Qin, G. W., Wang, H. B., Wang, P., et al. (2011). Acacetin causes a frequency- and use-dependent blockade of hKv1.5 channels by binding to the S6 domain. J. Mol. Cell Cardiol. 51, 966–973. doi: 10.1016/j.yjmcc.2011.08.022
Wu, W., Sun, H. Y., Deng, X. L., and Li, G. R. (2013b). EGFR tyrosine kinase regulates human small-conductance Ca2+-activated K+ (hSKCa1) channels expressed in HEK-293 cells. Biochem. J. 452, 121–129. doi: 10.1042/BJ20121324
Wu, W., Wang, Y., Deng, X. L., Sun, H. Y., and Li, G. R. (2013c). Cholesterol down-regulates BK channels stably expressed in HEK 293 cells. PLOS ONE 8:e79952. doi: 10.1371/journal.pone.0079952
Wu, W., Dong, M. Q., Wu, X. G., Sun, H. Y., Tse, H. F., Lau, C. P., et al. (2012). Human ether-a-go-go gene potassium channels are regulated by EGFR tyrosine kinase. Biochim. Biophys. Acta 1823, 282–289. doi: 10.1016/j.bbamcr.2011.10.010
Wulff, H., and Kohler, R. (2013). Endothelial small-conductance and intermediate-conductance KCa channels: an update on their pharmacology and usefulness as cardiovascular targets. J. Cardiovasc. Pharmacol. 61, 102–112. doi: 10.1097/FJC.0b013e318279ba20
Xu, Y., Tuteja, D., Zhang, Z., Xu, D., Zhang, Y., Rodriguez, J., et al. (2003). Molecular identification and functional roles of a Ca2+-activated K+ channel in human and mouse hearts. J. Biol. Chem. 278, 49085–49094. doi: 10.1074/jbc.M307508200
Zeng, W., Zhang, C., Cheng, H., Wu, Y. L., Liu, J., Chen, Z., et al. (2017). Targeting to the non-genomic activity of retinoic acid receptor-gamma by acacetin in hepatocellular carcinoma. Sci. Rep. 7:348. doi: 10.1038/s41598-017-00233-5
Zhang, X. D., Lieu, D. K., and Chiamvimonvat, N. (2015). Small-conductance Ca2+ -activated K+ channels and cardiac arrhythmias. Heart Rhythm 12, 1845–1851. doi: 10.1016/j.hrthm.2015.04.046
Keywords: acacetin, ion channels, potassium channels, small conductance Ca2+-activated potassium channels
Citation: Chen K-H, Liu H, Sun H-Y, Jin M-W, Xiao G-S, Wang Y and Li G-R (2017) The Natural Flavone Acacetin Blocks Small Conductance Ca2+-Activated K+ Channels Stably Expressed in HEK 293 Cells. Front. Pharmacol. 8:716. doi: 10.3389/fphar.2017.00716
Received: 30 July 2017; Accepted: 25 September 2017;
Published: 10 October 2017.
Edited by:
Marco Leonti, Università degli Studi di Cagliari, ItalyReviewed by:
Zhilin Qu, University of California, Los Angeles, United StatesMarcia Hiriart, National Autonomous University of Mexico, Mexico
Copyright © 2017 Chen, Liu, Sun, Jin, Xiao, Wang and Li. This is an open-access article distributed under the terms of the Creative Commons Attribution License (CC BY). The use, distribution or reproduction in other forums is permitted, provided the original author(s) or licensor are credited and that the original publication in this journal is cited, in accordance with accepted academic practice. No use, distribution or reproduction is permitted which does not comply with these terms.
*Correspondence: Gui-Rong Li, grli8@outlook.com