A novel two-dimensional transition metal dichalcogenide as water splitting photocatalyst with excellent performances
- 1School of Mechanical Engineering, Neutron Scattering Technical Engineering Research Center, Dongguan University of Technology, Dongguan, China
- 2School of Optoelectronic Science and Engineering, University of Electronic Science and Technology of China, Chengdu, China
- 3School of Materials Science and Engineering, Hebei University of Technology, Tianjin, China
- 4Guangdong-Taiwan College of Industrial Science & Technology, Dongguan University of Technology, Dongguan, China
With the rising demand for renewable energy, photocatalysts are considered the most promising solution to harness solar energy, and the search for photocatalysts with excellent performances remains an urgent task. Here, based on density functional theory (DFT), the photocatalytic properties of MoWS4 are systematically investigated. The MoWS4 monolayer and bilayer are demonstrated as semiconductors with indirect band gaps of 2.01 and 1.48 eV. Moreover, they exhibit high and anisotropic light absorption coefficients of up to ∼105 cm−1 in the visible-ultraviolet region. The intrinsic band edge positions could fully satisfy the redox potentials of water without any external adjustment. The electron mobility of MoWS4 monolayer is 557 cm2 V−1s−1, which is seven times higher than MoS2 monolayer. Hence, MoWS4 can be regarded as a promising 2D photocatalyst candidate for water splitting.
Introduction
With the depletion of fossil energy and the increasing pollution of the natural environment, the demands for renewable energy become critical and urgent for sustainable development of global economy. In 1972, Fujishima and Honda discovered that TiO2 can split water to produce hydrogen and oxygen in the presence of sunlight, making photocatalysis one of the most noteworthy solutions to harness solar energy (Fujishima and Honda, 1972). Afterwards, great efforts have been made to develop effective photocatalysts, including transition metal oxides, sulfides, nitrides, and so forth (Tsuji et al., 2005; Suntivich et al., 2011; Han et al., 2018). However, the low quantum efficiency derived from charge recombination on the surface and in the bulk of these photocatalysts could not meet the criteria of favorable photocatalyst for sunlight driven water splitting (Fujishima et al., 2000).
Due to the interesting structures and corresponding electronic properties, two-dimensional (2D) materials have been widely used in various fields and also provide new research directions for efficient photocatalysis (Singh et al., 2015). In recent years, 2D photocatalysts showed the greater advantages over their bulk phase counterparts in terms of photocatalytic performance. For example, Both SnS2 monolayer and ZnSe nanosheet exhibited higher photocurrent density than their bulk materials (Sun et al., 2012a; Sun et al., 2012b). In addition, various 2D materials have also been theoretically and experimentally demonstrated to be used as photocatalysts for water splitting, such as 2D transition metal dichalcogenides, g-C3N4, phosphorene, and so on (Wang et al., 2009; Zhuang and Hennig, 2013a; Rahman et al., 2016; Phuc et al., 2018; Zhang et al., 2022). However, few of these photocatalysts can simultaneously satisfy high visible light absorption, high carrier mobility and perfect band edge positions. For instance, MoS2 monolayer showed lower carrier mobility, and MoTe2 monolayer can’t perfectly meet the redox potential of water (Wang et al., 2009; Zhuang and Hennig, 2013a; Cai et al., 2014; Rahman et al., 2016; Zhang et al., 2022). Both GaS and GaSe monolayers demonstrated low visible light absorption due to the large band gaps (Zhuang and Hennig, 2013b). Therefore, it is still a challenge to develop water splitting photocatalysts with excellent performances.
In this work, based on the first-principles calculations, we propose a novel 2D transition metal dichalcogenide namely MoWS4 and systemically investigate its photocatalytic properties. Firstly, the stability of MoWS4 monolayer is confirmed by calculating its phonon spectra and ab initio molecular dynamics (AIMD) simulations. Secondly, we calculate the band structures of MoWS4 monolayer and bilayer, and show their semiconductive characteristic. Then, relevant photocatalytic properties are systematically investigated. It is found that MoWS4 monolayer and bilayer can nicely meet the redox potentials without strain engineering, and their light absorption coefficients reach ∼105 cm−1 in the visible-ultraviolet region. Moreover, the electronic mobility of MoWS4 monolayer is as high as 557 cm2 V−1s−1.
Computational details
For geometric and electronic structures, all calculations are performed by using the Vienna ab into simulation package (VASP) based on density functional theory (DFT) (Kresse and Furthmuller, 1996). We choose the generalized gradient approximation (GGA) of the Perdew–Burke–Ernzerhof (PBE) functional as the exchange-correlation functional to perform these calculations (Perdew et al., 1996). For the 2D monolayer structure, the vacuum layer thickness is set as about 20 Å to avoid layer-to-layer effects. The kinetic energy cutoff is set as 500 eV. The Brillouin zone is regulated with 10 × 6 × 1. During the calculations, the DFT-D2 method with Grimme correction is used to describe the long-range van der Waals interactions (Grimme, 2006). Besides, all atoms are fully relaxed, and the energy and force convergence criteria are set as 10–6 eV and 0.01 eV Å−1, respectively. Except for PBE functional, to obtain the more accurate results, the HSE06 functional is also adopted to calculate the band structures and the band edge positions (Deák et al., 2010). To identify structural stability of MoWS4 monolayer, its phonon spectra are calculated by using the PHONOPY code (Gonze and Lee, 1997). A 6 × 3 supercell structure of MoWS4 monolayer is used in ab initio molecular dynamics (AIMD) simulations (NVT ensemble), which is carried out for 3 ps with a time step of 1 fs at 500 K (Cimas et al., 2014).
The optical properties can be defined by the complex dielectric function (frequency) for characterization:
where
where
where
where
The carrier mobility is calculated by the following formula (Qiao et al., 2014):
where
Results and discussions
Crystal structures, stability, and electronic properties
Figure 1A shows the top and side views of 4 × 2 supercell of MoWS4 monolayer, which has a space group of Pmm2. The light green area is the unit cell of MoWS4 monolayer, which contains four atoms. The pink, blue, and yellow balls represent Mo, W, and S atoms, respectively. MoWS4 monolayer is a honeycomb structure from the top view, while sandwich structure from the side view, which is very similar to the structure of MoS2 monolayer (Kan et al., 2014). After fully geometric optimization, the lattice constants are a = 3.188 Å and b = 5.526 Å with a layer thickness of 3.119 Å.
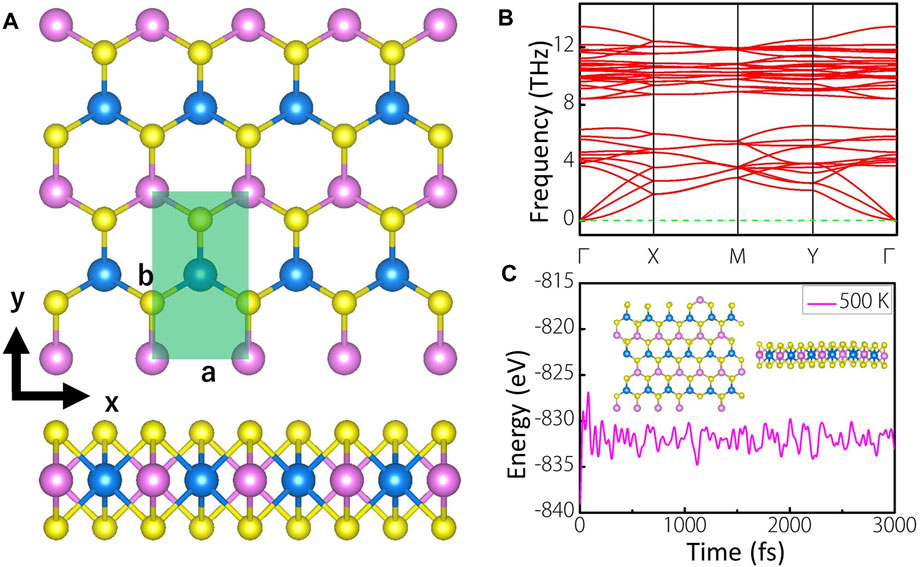
FIGURE 1. (A) Top and side views of MoWS4 monolayer. The pink, blue, and yellow balls represent Mo, W, and S atoms, respectively. The light green area is the unit cell of MoWS4 monolayer (B) Phonon spectra of MoWS4 monolayer (C) Evolution of the total energy and a snapshot of MoWS4 monolayer after a 3,000 fs AIMD simulations in vacuum at 500K
To confirm the stability of MoWS4 monolayer, the formation energy (
Figure 2 shows the top and side views of MoWS4 bilayer. Three stacking patterns (AA, AB, and AC) are considered in the bilayer structures of MoWS4. The results of the calculated total energy are −94.2727 eV, −94.4175 eV, and −94.2726 eV for AA, AB, and AC stacking patterns, respectively. AA and AC stacking structures have the same layer spacing of 6.76 Å, and AB stacking has a spacing of 6.13 Å. Obviously, the AB stacking structure is most stable energetically.
Figures 3A,B shows the electronic band structures of MoWS4 monolayer and bilayer obtained by using the PBE functional (blue) and the HSE06 functional (red). The Fermi level and the high symmetry path are set as 0 eV and Γ-X-M-Y-Γ, respectively. The MoWS4 monolayer and bilayer are indirect band gap semiconductors and their band gaps calculated by HSE06/PBE functional are 2.01/1.67 eV and 1.48/1.14 eV, respectively. The band gaps obtained by the HSE06 functional are larger than that obtained by the PBE functional since the PBE functional tends to underestimate the band gap. Figures 3C,D shows the maps of charge density for MoW4 monolayer at the conduction band minimum (CBM) and the valence band maximum (VBM), and it can be concluded that the CBM is mainly contributed by the Mo atoms, whereas the VBM comes from a combination of Mo, W, and S atoms.
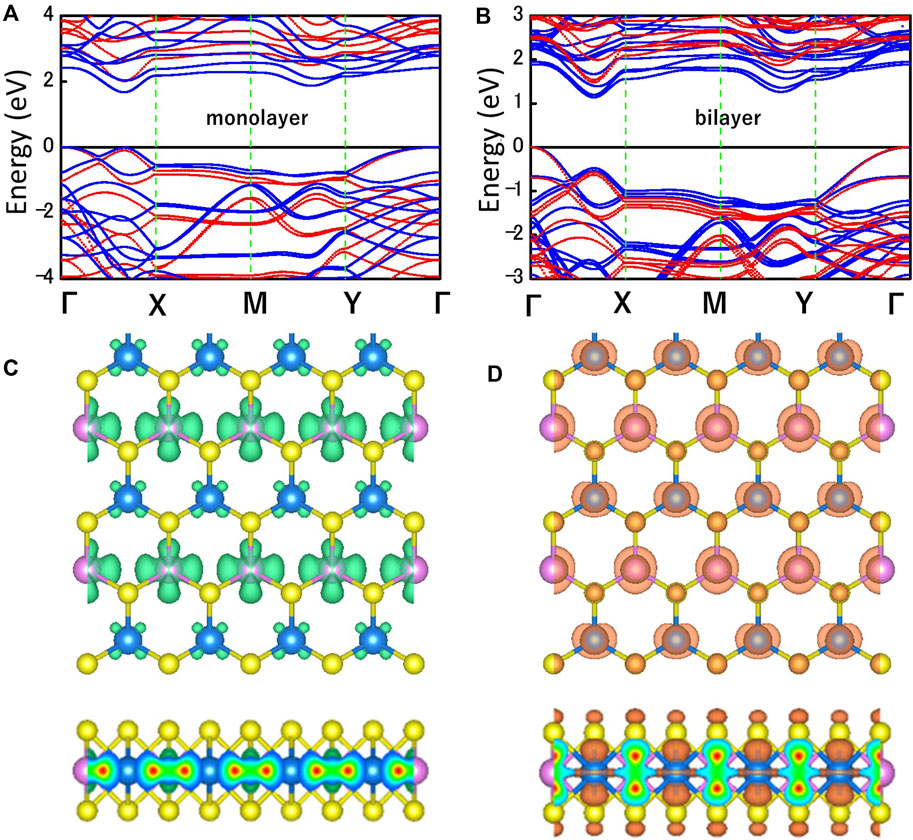
FIGURE 3. Band structures of (A) MoWS4 monolayer and (B) MoWS4 bilayer. The red and blue represent the HSE06 functional and PBE functional, respectively. Top and side views of the charge density at the (C) CBM, and (D) VBM. The isosurface value is set as 0.012 e Å−3.
Photocatalytic water splitting and optical properties
Considering the excellent semiconductor properties of MoWS4 monolayer and bilayer, we systematically study their feasibility as photocatalysts for water splitting. It is well known that a photocatalytic candidate should meet the following conditions: Firstly, its band gap should exceed the free energy of water splitting (1.23 eV). Obviously, the band gaps of MoWS4 monolayer and bilayer both exceed 1.23 eV; Secondly, its band edges must cross the redox potentials of water. The CBM energy should be higher than the reduction potential of H+/H2 (−4.44 eV), and the VBM energy should be lower than the oxidation potential of O2/H2O (−5.67 eV) (Abe, 2010; Sun et al., 2019; Cheng et al., 2022b). For 2D materials, the band edges with respect to the vacuum level (
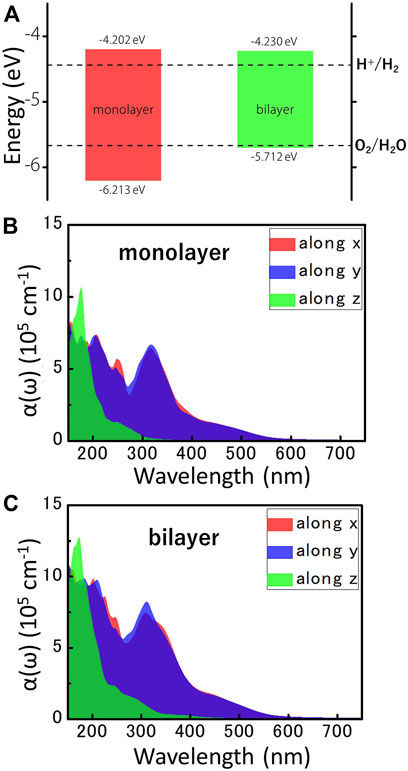
FIGURE 4. (A) The locations of VBM and CBM of MoWS4 monolayer and bilayer, which are obtained by the HSE06 functional. The redox potentials of water splitting at pH = 0 are shown for comparison. All the energy levels are based on the vacuum level, and the vacuum level is set as 0 eV. The optical absorption coefficients for the different directions of (B) MoWS4 monolayer and (C) MoWS4 bilayer.
Besides, efficient light absorption is also an important feature for water splitting photocatalysts. Figures 4B,C shows the light absorption spectra of the MoWS4 monolayer and bilayer within the visible-ultraviolet light range. The result can be summarized as follows: 1) Their light absorption coefficients in the ultraviolet light range are higher than visible light region (up to ∼106 cm−1); 2) Their light absorption coefficients of MoWS4 bilayer overall are higher than that of MoWS4 monolayer; 3) Their light absorption coefficients are significantly anisotropic: that is higher in the z direction, but have a wider range of light absorption spectra in the x and y directions. It is known that the high light absorption coefficients can guarantee the effective use of solar energy, which is very favorable for water splitting photocatalysts (Zhao et al., 2018; Fan et al., 2021). Therefore, both MoWS4 monolayer and bilayer can be promising potential candidates as photocatalysts.
Strain engineering and high carrier mobility
We further investigate the effect of strain engineering on the photocatalytic performance of MoWS4 monolayer. Figure 5 shows the band structures of MoWS4 monolayer under the in-plane biaxial strain from −2 to 2%. Its band gap increases when compressive strain increases or tensile strain decreases, and its band gap varies in the range of 1.508–2.378 eV. In addition, when it is subjected to compressive strain, the indirect bandgap changes to be a direct bandgap; while when it is subjected to tensile strain, it remains an indirect bandgap semiconductor. As illustrated in Figure 6, we study the changes of its band edge positions under the applied in-plane biaxial strain. All of VBM positions are lower than the oxidation potential of O2/H2O under the −2∼2% in-plane biaxial strain, which indicates that MoWS4 monolayer always serves as a potential photocatalyst to generate oxygen. Besides, when the applied tensile strain reaches 2%, its CBM positions become lower than the reduction potential of H+/H2. Thus, excessive tensile strain will lead to its inability to produce hydrogen. In general, compressive strain does not cause MoWS4 monolayer to deviate from the basic requirements for water splitting photocatalyst, but tensile strain can easily affect its hydrogen production performance.
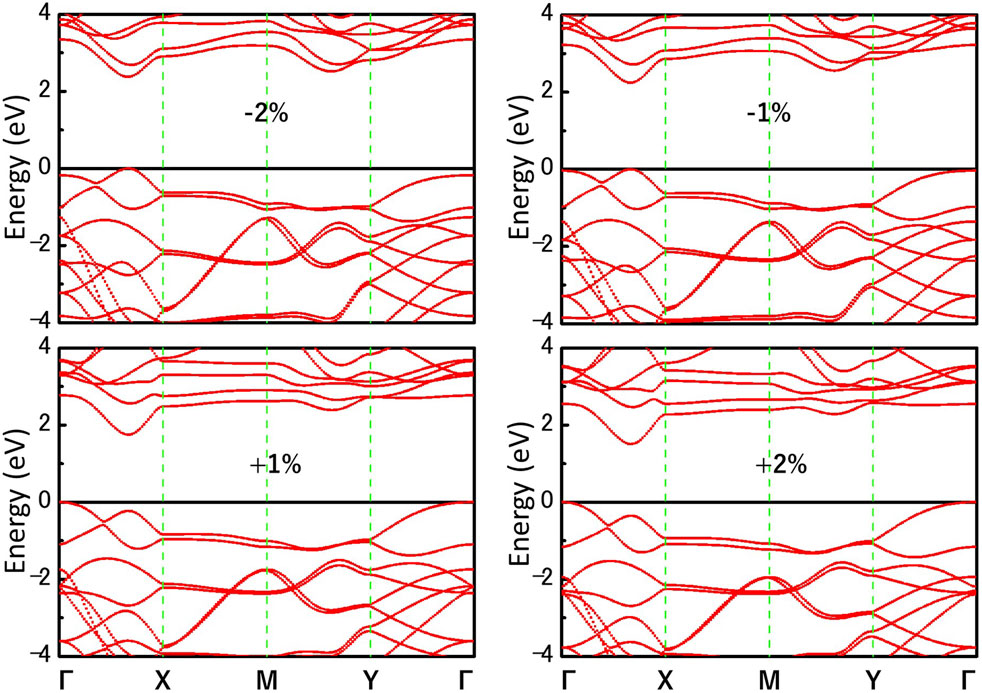
FIGURE 5. Band structures of MoWS4 monolayer under the biaxial strain from −2 to 2% are calculated by HSE06 functional.
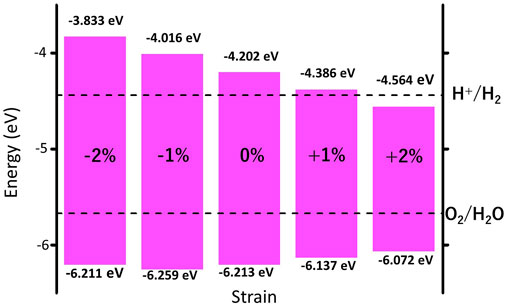
FIGURE 6. Strain effects on band edge positions of MoWS4 monolayer with respect to the vacuum level (0 eV). The redox potentials of water splitting at pH = 0 are shown for comparison.
As we know, the fast carrier migration capability is necessary for high performance photocatalysts. Thus, the PBE functional is used to calculate the carrier mobility of MoWS4 monolayer according to Eq. 5. Subsequently, we calculate its carrier effective masses
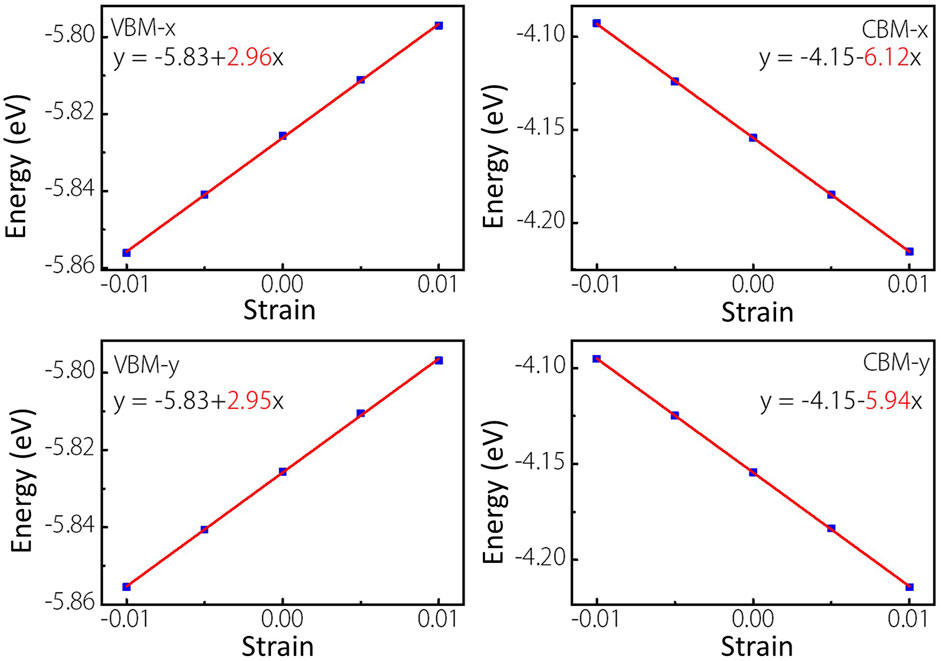
FIGURE 7. Linear fitting maps of VBM and CBM locations of MoWS4 monolayer under the strain along x and y directions.

TABLE 1. Carrier effective masses
Conclusion
Based on the first-principles calculations, we systematically investigate photocatalytic properties of two-dimensional MoWS4. It is found that both the MoWS4 monolayer and bilayer are semiconductors with indirect band gaps and show high and anisotropic light absorption coefficients in the visible-ultraviolet range. The band edge positions of the materials can satisfy the redox potentials perfectly and the electron mobility of MoWS4 monolayer is up to 557 cm2 V−1 s−1, which outperforms many other 2D photocatalytic materials, such as MoS2 monolayer, Penta-PdS2, Penta-PdSe2, and SnP2S6 monolayer. These results indicate that MoWS4 can be a promising photocatalyst for water splitting with outstanding performances.
Data availability statement
The original contributions presented in the study are included in the article/Supplementary Material, further inquiries can be directed to the corresponding authors.
Author contributions
FW: Methodology, Investigation, Writing-original draft. ZC: Conceptualization, Investigation, Methodology, Writing—original draft. XZ: Investigation, Writing-original draft. CX: Validation, Resources. FL: Data curation, Validation. CC: Conceptualization, Writing—original draft, Supervision, Funding acquisition. GL: Validation, Data curation, Supervision.
Funding
The authors are grateful for support from the National Natural Science Foundation of China (52101192 and 51871056), Foundation for Basic and Applied Basic Research-Joint Foundation of Dongguan-Guangdong Province (2020A1515111109), and Department of Education of Guangdong in China (2018KZDXM069 and 2021 KCXTD050).
Conflict of interest
The authors declare that the research was conducted in the absence of any commercial or financial relationships that could be construed as a potential conflict of interest.
Publisher’s note
All claims expressed in this article are solely those of the authors and do not necessarily represent those of their affiliated organizations, or those of the publisher, the editors and the reviewers. Any product that may be evaluated in this article, or claim that may be made by its manufacturer, is not guaranteed or endorsed by the publisher.
References
Abe, R. (2010). Recent progress on photocatalytic and photoelectrochemical water splitting under visible light irradiation. J. Photochem. Photobiol. C Photochem. Rev. 11, 179–209. doi:10.1016/j.jphotochemrev.2011.02.003
Bardeen, J., and Shockley, W. (1950). Deformation potentials and mobilities in non-polar crystals. Phys. Rev. 80, 72–80. doi:10.1103/physrev.80.72
Cai, Y. Q., Zhang, G., and Zhang, Y. W. (2014). Polarity-reversed robust carrier mobility in monolayer MoS2 nanoribbons. J. Am. Chem. Soc. 136, 6269–6275. doi:10.1021/ja4109787
Cheng, Z. S., Zhang, X. M., Zhang, H., Liu, H. Y., Dai, X. F., Liu, G. D., et al. (2022). Binary pentagonal auxetic materials for photocatalysis and energy storage with outstanding performances. Nanoscale 14, 2041–2051. doi:10.1039/d1nr08368f
Cheng, Z. S., Zhang, X. M., Zhang, H., Liu, H. Y., Dai, X. F., Liu, G. D., et al. (2022). Two-dimensional auxetic pentagonal materials as water splitting photocatalysts with excellent performances. J. Mat. Sci. 57, 7667–7679. doi:10.1007/s10853-022-07130-x
Cimas, Á., Tielens, F., Sulpizi, M., Gaigeot, M. P., and Costa, D. (2014). The amorphous silica-liquid water interface studied by ab initio molecular dynamics (AIMD): Local organization in global disorder. J. Phys. Condens. Matter 26, 244106. doi:10.1088/0953-8984/26/24/244106
Deák, P., Aradi, B., Frauenheim, T., Janzén, E., and Gali, A. (2010). Accurate defect levels obtained from the HSE06 range-separated hybrid functional. Phys. Rev. B 81, 153203. doi:10.1103/physrevb.81.153203
Fan, Y. S., Xi, X. L., Liu, Y. S., Nie, Z. R., Zhao, L. Y., and Zhang, Q. H. (2021). Regulation of morphology and visible light-driven photocatalysis of WO3 nanostructures by changing pH. Rare Met. 40, 1738–1745. doi:10.1007/s12598-020-01490-6
Fujishima, A., and Honda, K. (1972). Electrochemical photolysis of water at a semiconductor electrode. Nature 238, 37–38. doi:10.1038/238037a0
Fujishima, A., Rao, T. N., and Tryk, D. A. (2000). Titanium dioxide photocatalysis. J. Photochem. Photobiol. C Photochem. Rev. 1, 1–21. doi:10.1016/s1389-5567(00)00002-2
Gonze, X., and Lee, C. Y. (1997). Dynamical matrices, born effective charges, dielectric permittivity tensors, and interatomic force constants from density-functional perturbation theory. Phys. Rev. B 55, 10355–10368. doi:10.1103/physrevb.55.10355
Grimme, S. (2006). Semiempirical GGA-type density functional constructed with a long-range dispersion correction. J. Comput. Chem. 27, 1787–1799. doi:10.1002/jcc.20495
Han, N., Liu, P. Y., Jiang, J., Ai, L. H., Shao, Z. P., and Liu, S. M. (2018). Recent advances in nanostructured metal nitrides for water splitting. J. Mat. Chem. A 6, 19912–19933. doi:10.1039/c8ta06529b
Jing, Y., Zhou, Z. P., Zhang, J., Huang, C. B., Li, Y. F., and Wang, F. (2019). SnP2S6 monolayer: A promising 2D semiconductor for photocatalytic water splitting. Phys. Chem. Chem. Phys. 21, 21064–21069. doi:10.1039/c9cp04143e
Kan, M., Wang, J. Y., Li, X. W., Zhang, S. H., Li, Y. W., Kawazoe, Y., et al. (2014). Structures and phase transition of a MoS2 monolayer. J. Phys. Chem. C 118, 1515–1522. doi:10.1021/jp4076355
Kresse, G., and Furthmuller, J. (1996). Efficient iterative schemes for ab initio total-energy calculations using a plane-wave basis set. Phys. Rev. B 54, 11169–11186. doi:10.1103/physrevb.54.11169
Kuzmenko, A. B. (2005). Kramers-Kronig constrained variational analysis of optical spectra. Rev. Sci. Instrum. 76, 083108. doi:10.1063/1.1979470
Liu, X. F., Zhang, Z. F., Ding, Z., Lv, B., Luo, Z. J., Wang, J. S., et al. (2021). Highly anisotropic electronic and mechanical properties of monolayer and bilayer As2S3. Appl. Surf. Sci. 542, 148665. doi:10.1016/j.apsusc.2020.148665
Long, C., Liang, Y., Jin, H., Huang, B. B., and Dai, Y. (2018). PdSe2: Flexible two-dimensional transition metal dichalcogenides monolayer for water splitting photocatalyst with extremely low recombination rate. ACS Appl. Energy Mat. 2, 513–520. doi:10.1021/acsaem.8b01521
Perdew, J. P., Burke, K., and Ernzerhof, M. (1996). Generalized gradient approximation made simple. Phys. Rev. Lett. 77, 3865–3868. doi:10.1103/physrevlett.77.3865
Phuc, H. V., Hieu, N. N., Hoi, B. D., Hieu, N. V., Thu, T. V., Hung, N. M., et al. (2018). Tuning the electronic properties, effective mass and carrier mobility of MoS2 monolayer by strain engineering: First-principle calculations. J. Electron. Mat. 47, 730–736. doi:10.1007/s11664-017-5843-8
Qiao, J. S., Kong, X. H., Hu, Z. X., Yang, F., and Ji, W. (2014). High-mobility transport anisotropy and linear dichroism in few-layer black phosphorus. Nat. Commun. 5, 4475–4477. doi:10.1038/ncomms5475
Rahman, M. Z., Kwong, C. W., Davey, K., and Qiao, S. Z. (2016). 2D phosphorene as a water splitting photocatalyst: Fundamentals to applications. Energy Environ. Sci. 9, 709–728. doi:10.1039/c5ee03732h
Saha, S., Sinha, T. P., and Mookerjee, A. (2000). Electronic structure, chemical bonding, and optical properties of paraelectric BaTiO3. Phys. Rev. B 62, 8828–8834. doi:10.1103/physrevb.62.8828
Singh, A. K., Mathew, K., Zhuang, H. L., and Hennig, R. G. (2015). Computational screening of 2D materials for photocatalysis. J. Phys. Chem. Lett. 6, 1087–1098. doi:10.1021/jz502646d
Sun, Y. F., Cheng, H., Gao, S., Sun, Z. H., Li, Q. H., Liu, Q., et al. (2012). Freestanding tin disulfide single‐layers realizing efficient visible‐light water splitting. Angew. Chem. Int. Ed. Engl. 51, 8857–8861. doi:10.1002/ange.201204675
Sun, Y. F., Sun, Z. H., Gao, S., Cheng, H., Liu, Q. H., Piao, J. Y., et al. (2012). Fabrication of flexible and freestanding zinc chalcogenide single layers. Nat. Commun. 3, 1057–7. doi:10.1038/ncomms2066
Sun, S. S., Meng, F. C., Wang, H. Y., Wang, H., and Ni, Y. X. (2018). Novel two-dimensional semiconductor SnP3: High stability, tunable bandgaps and high carrier mobility explored using first-principles calculations. J. Mat. Chem. A 6, 11890–11897. doi:10.1039/c8ta02494d
Sun, S. S., Meng, F. C., Xu, Y. F., He, J., Ni, Y. X., and Wang, H. Y. (2019). Flexible, auxetic and strain-tunable two dimensional penta-X2C family as water splitting photocatalysts with high carrier mobility. J. Mat. Chem. A 7, 7791–7799. doi:10.1039/c8ta12405a
Suntivich, J., May, K. J., Gasteiger, H. A., Goodenough, J. B., and Yang, S. H. (2011). A perovskite oxide optimized for oxygen evolution catalysis from molecular orbital principles. Science 334, 1383–1385. doi:10.1126/science.1212858
Tsuji, I., Kato, H., and Kudo, A. (2005). Visible‐light‐induced H2 evolution from an aqueous solution containing sulfide and sulfite over a ZnS-CuInS2-AgInS2 solid‐solution photocatalyst. Angew. Chem. Int. Ed. Engl. 117, 3631–3634. doi:10.1002/ange.200500314
Wang, X. C., Maeda, K., Thomas, A., Takanabe, K., Xin, G., Carlsson, J. M., et al. (2009). A metal-free polymeric photocatalyst for hydrogen production from water under visible light. Nat. Mat. 8, 76–80. doi:10.1038/nmat2317
Wang, Y., Li, Y., and Chen, Z. F. (2015). Not your familiar two dimensional transition metal disulfide: Structural and electronic properties of the PdS2 monolayer. J. Mat. Chem. C 3, 9603–9608. doi:10.1039/c5tc01345c
Xiao, F., Lei, W., Wang, W., Xu, L. L., Zhang, S. L., and Ming, X. (2021). Pentagonal two-dimensional noble-metal dichalcogenide PdSSe for photocatalytic water splitting with pronounced optical absorption and ultrahigh anisotropic carrier mobility. J. Mat. Chem. C 9, 7753–7764. doi:10.1039/d1tc01245b
Zhang, W., Chai, C. C., Fan, Q. Y., Sun, M. L., Song, Y. X., Yang, Y. T., et al. (2021). Two-dimensional tetrahex-GeC2: A material with tunable electronic and optical properties combined with ultrahigh carrier mobility. ACS Appl. Mat. Interfaces 13, 14489–14496. doi:10.1021/acsami.0c23017
Zhang, Q., Wang, X., and Yang, S. L. (2021). δ-SnS: An emerging bidirectional auxetic direct semiconductor with desirable carrier mobility and high-performance catalytic behavior toward the water-splitting reaction. ACS Appl. Mat. Interfaces 13, 31934–31946. doi:10.1021/acsami.1c03650
Zhang, Y. F., Wei, J. M., Liu, T. Y., Zhong, Z., Luo, Z. J., Xiao, W. J., et al. (2022). Tunable properties of ZnSe/graphene heterostructure as a promising candidate for photo/electro-catalyst applications. Appl. Surf. Sci. 574, 151679. doi:10.1016/j.apsusc.2021.151679
Zhao, P., Ma, Y. D., Lv, X. S., Li, M. M., Huang, B. B., and Dai, Y. (2018). Two-dimensional III2-VI3 materials: Promising photocatalysts for overall water splitting under infrared light spectrum. Nano Energy 51, 533–538. doi:10.1016/j.nanoen.2018.07.010
Zhuang, H. L., and Hennig, R. G. (2013). Computational search for single-layer transition-metal dichalcogenide photocatalysts. J. Phys. Chem. C 117, 20440–20445. doi:10.1021/jp405808a
Keywords: two-dimensional materials, transition metal dichalcogenides, water splitting photocatalyst, high mobility, density functional theory
Citation: Wang F, Cheng Z, Zhang X, Xie C, Liu F, Chang C and Liu G (2022) A novel two-dimensional transition metal dichalcogenide as water splitting photocatalyst with excellent performances. Front. Chem. 10:1003027. doi: 10.3389/fchem.2022.1003027
Received: 25 July 2022; Accepted: 03 August 2022;
Published: 25 August 2022.
Edited by:
Guangzhao Wang, Yangtze Normal University, ChinaCopyright © 2022 Wang, Cheng, Zhang, Xie, Liu, Chang and Liu. This is an open-access article distributed under the terms of the Creative Commons Attribution License (CC BY). The use, distribution or reproduction in other forums is permitted, provided the original author(s) and the copyright owner(s) are credited and that the original publication in this journal is cited, in accordance with accepted academic practice. No use, distribution or reproduction is permitted which does not comply with these terms.
*Correspondence: Zishuang Cheng, czs19950627@163.com; Chuntao Chang, changct@dgut.edu.cn