- National Clinical Research Center for Metabolic Diseases, Key Laboratory of Diabetes Immunology (Central South University), Ministry of Education, Metabolic Syndrome Research Center, and Department of Metabolism and Endocrinology, The Second Xiangya Hospital of Central South University, Changsha, China
The rapid increase of obesity and associated diseases has become a major global health problem. Adipose tissues are critical for whole-body homeostasis. The gut microbiota has been recognized as a significant environmental factor in the maintenance of energy homeostasis and host immunity. A growing body of evidence suggests that the gut microbiota regulates host metabolism through a close cross-talk with adipose tissues. It modulates energy expenditure and alleviates obesity by promoting energy expenditure, but it also produces specific metabolites and structural components that may act as the central factors in the pathogenesis of inflammation, insulin resistance, and obesity. Understanding the relationship between gut microbiota and adipose tissues may provide potential intervention strategies to treat obesity and associated diseases. In this review, we focus on recent advances in the gut microbiota and its actions on adipose tissues and highlight the joint actions of the gut microbiota and adipose tissue with each other in the regulation of energy metabolism.
1 Introduction
Obesity, a chronic disease characterized by the excessive expansion of adipose tissues and associated low-grade inflammation, is the risk factor for various disorders, including insulin resistance (IR), metabolic syndrome, hypertension, type 2 diabetes mellitus (T2D), cardiovascular diseases, nonalcoholic fatty liver disease (NAFLD), cancers and mental diseases (1, 2). Genome-wide association (GWAS) studies have found that fat mass and obesity-associated (FTO) gene and its single nucleotide polymorphisms (SNPs) are associated with obesity (3). Such as SNP rs 1558902 was proved to be associated with BMI in a study including 247,796 Europeans (3). Besides genetic deficiency, such as leptin or leptin receptor deficiency (4, 5), obesity is mainly caused by a disrupted balance of energy homeostasis with increased nutrition intake and decreased energy expenditure (6). New therapeutic strategies through reductions in energy intake, absorption, or storage are crucial to fight against the worldwide epidemic of obesity.
Although obesity is characterized by excessive accumulation of adipose tissues, under physiological conditions, adipose tissues are critical for whole-body homeostasis, including lipid storage, thermoregulation, and secretion of adipokines to regulate energy balance, metabolism, and immune responses (7). According to different phenotypes, distribution, and physiological functions, adipose tissues are classified into white and brown adipose tissues. White adipose tissue (WAT), as the majority form of the adipose tissue mass in our body, is mainly responsible for energy storage in the form of triglycerides (TG) (7). It also secretes many adipokines, such as leptin and adiponectin, to regulate whole-body homeostasis (7). Brown adipose tissue (BAT) represents a small part of the total fat mass and is located predominantly in the interscapular and supraclavicular regions of adult humans (8). BAT expresses unique uncoupling protein 1 (UCP1), which eliminates the difference in transmembrane proton concentration between the two sides of the mitochondrial inner membrane and blocks the adenosine triphosphate (ATP) synthesis, resulting in the dissipation of energy as heat (9). Thus, BAT is involved in thermoregulatory, energy expenditure, and insulin sensitivity. An inducible form of brown-like adipocytes, namely beige adipocytes, can be found interspersed in WAT depots (10). Similar to brown adipocytes, beige adipocytes also express UCP1 and are involved in non-shivering thermogenesis and the dissipation of energy in response to cold exposure or β-adrenergic receptor (βAR) activators (10, 11). WAT, BAT, and beige adipocytes are important for energy balance, and their dysfunctions are closely associated with the pathogenesis of obesity and metabolic diseases.
Recently, gut microbiota has been viewed as an important regulator for the maintenance of host energy homeostasis and immunity (12). In human digestive system, there are around 10 trillion microbiota, carrying more than 9.9 million microbial genes (12, 13). Gut microbiota has lots of functions, including keeping in charge of metabolism and nutrition absorption, protection of the integrity of intestinal mucous, and regulation of the immune responses. In 2004, Gordon and colleagues first verified that gut microbiota is a crucial environmental factor that regulates energy homeostasis and fat accumulation (14). After that, with the development of sequencing techniques and bioinformatics (13), lots of studies have demonstrated a close relationship between gut microbiota and the host energy homeostasis. Studies in both mice (15) and humans (16) have shown that obesity is closely linked to the altered gut microbiota, including lower microbial richness and diversity and decreased levels of Bacteroidetes, one of the most abundant phyla in the gut (17, 18). Altered microbial composition is related to the alterations in the gut microbial metagenome, especially an enrichment of genes involved in energy harvest (14). Nutrients/different diets, medication, and basic metabolic state (energy balance, etc.) of the host can impact the abundance or composition of the gut microbial population, which, in turn, may affect not only the host’s energy expenditure (weight loss) but also energy storage (weight gain) (19).
A growing body of evidence suggests that the gut microbiota is a bridge to connect the external environment and the host homeostasis. On the one hand, the composition of gut microbiota can be affected by region (20), race/ethnicity (21), diet (22–24), and host genetics (6). For example, geographical location has been suggested to be closely associated with gut microbiota variation (20), and race/ethnicity (22) or its correlates such as diet and socioeconomic status have important influences on gut microbiota. Western diet with high fat and low fiber is one of the most significant drivers of metabolic diseases (21, 25). Besides, host genetics can have a fundamental influence on the composition and function of gut microbiota. Interactions between host genetics and diet modulate gut microbiota and susceptibility to obesity and metabolic diseases (25, 26).
On the other hand, gut microbiota modulates host homeostasis by the distal organs such as liver (27) and adipose tissues (28) via metabolites and cytokines. The liver communicates with the intestine by bile acids (BAs) and many bioactive mediators such as hepatokine FGF21 (27). Gut microbiota regulates energy expenditure and alleviates obesity by promoting browning of WAT, the activity of BAT, and lipid metabolism. Gut microbiota also produces specific microbial-derived metabolites and structural components, such as short-chain fatty acids (SCFA), lipopolysaccharide (LPS), and peptidoglycans, which may act as the central factors in the pathogenesis of inflammation, insulin resistance, and obesity (18, 29). Thus, understanding the relationship between gut microbiota and adipose tissues and the potential mechanism may provide new approaches to treat obesity and related metabolic diseases. In this review, we will focus on recent advances in the connection between gut microbiota and adipose tissues and highlight the mutual actions of gut microbiota and adipose tissue with each other in the regulation of energy metabolism.
2 Gut Microbiota and Adipose Tissues - Two Edges of One Sword
A growing body of evidence suggests that the relationship between gut microbiota and adipose tissue is complicated. It may influence the development of metabolic alterations of white adipose tissues associated with inflammation or the activity of brown and beige adipose tissues for energy expenditure and weight loss.
2.1 Gut Microbiota Regulates Adipose Tissue Expansion and Lipid Metabolism
Earlier studies have shown that gut microbiota participates in the development of adipose tissues in both normal and pathological conditions. Gut microbiota’s ability to harvest energy has been proposed as a major contributor of conventional mice (harbored a microbiota beginning at birth) in fat storage. Conventional mice have 42% more total body fat mass than germ-free (GF) mice (raised in the absence of any detectable microorganisms) (14). Adult GF mice transplanted with the gut microbiota, which is harvested from the distal intestine (cecum) of conventionally raised mice, produce a 60% increase in body fat content within 2 weeks (14). Obesity-associated gut microbiome can increase the capacity for energy harvest from the diet, thus contributing to increased adipose tissue storage of the host, especially the WAT (30). Transplantation of gut microbiota isolated from obese mice to GF mice resulted in a greater increase in total body weight and fat mass compared with colonization with gut microbiota from lean donors (30). And GF mice have a significant reduction of energy harvest that is accompanied with thinner intestinal villi, larger cecum, and decreased inflammatory responses (30, 31). Mechanistically, GF animals are protected from diet-induced obesity by inducing fatty acid metabolism through elevated levels of fasting-induced adipocyte factor (Fiaf)/angiopoietin-like protein 4 (Angptl4). Angptl14 is a circulating lipoprotein lipase (LPL) inhibitor, which can be selectively suppressed in the gut epithelium by the microbiota, and/or through increased AMP-activated protein kinase (AMPK) activity (14, 32).
The dynamic linkage between adiposity and gut microbial ecology has also been observed in human subjects. People with obesity tend to have higher levels of the phylum Firmicutes and fewer Bacteroidetes counts than normal-weight individuals (33). A study including more than 800 individuals indicates that gut microbiota may explain 2.74% variation in BMI, 2.46% of HDL in plasma, and 3.8% of TG in plasma (34). A crossover clinical trial with 21 individuals observed that increases of 20% in Firmicutes and decreases of a corresponding in Bacteroidetes were linked to an increased energy harvest (35). Similar phenomena were observed in obese children (36) and adult female twin pairs discordant for obesity (37). Using fecal microbiota transplantation from one in twin pairs to mouse respectively, researchers found that the increased fat mass of mice who had received an obese twin’s fecal microbiota was notably higher than the mice that received a lean twin’s fecal microbiota (37).
Omics analysis revealed significant differences between conventional and GF mice in their global adipose lipid (38, 39). The gut microbiota significantly increases triglyceride (TG) levels in the WAT, which is correlated with increased adipose tissue mass in conventional mice, compared with GF counterparts. Interestingly, TG levels were also higher in the liver of conventional mice, but lower in their serum, indicating increased lipid clearance by microbiota (39). Roy et al. found that compared with GF mice, conventional Apoe-/-mice had higher plasma cholesterol levels, hepatic cholesterol, and HDL cholesterol levels. Moreover, transplant of gut microbiota from humans elevated plasma cholesterol levels in mice, which might correlate with Betaproteobacteria, Alistipes, Bacteroides, and Barnesiella tax (40). These lipidomic results are in agreement with previous discoveries showing that microbiota increases LPL and promotes lipid clearance through suppression of Angptl4/Fiaf expression (32). LPL helps TG enter the circulation system through the liver and then TG is absorbed and stored by fat cells (41).
Certain strains of bacteria play an important role in nutrient absorption. Tazi et.al, using two models of commensal microorganisms, Lactobacillus paracasei and Escherichia coli, demonstrated the role of gut microbiota in regulating lipid absorption and metabolism through the epithelium. It has been reported that under homeostatic conditions, L. paracasei boosts lipid accumulation in enterocytes, while E. coli raises lipid catabolism and suppresses chylomicron circulating levels through the inhibition of mTOR pathways (42). This is consistent with the results in human studies (16, 35). However, L. paracasei-colonized mice but not E. coli-colonized mice resist high-fat-diet (HFD)-induced hypercholesterolemia and an increase in body weight (43). Conversely, Aronsson et al. demonstrated that the probiotic bacteria L. paracasei ssp paracasei F19 (F19) decreases lipid accumulation in mice fed with HFD by increasing Angptl4 and AMPK activity (32, 44). These two contradictory observations may be a result of the complex interplay between host genetic factors, environmental factors, or the biological properties of gut microbiota.
Although currently available data suggest that gut microbiota is closely associated with increased fat mass by promoting lipid absorption, lipogenesis, and adipogenesis, the underlying molecular mechanisms are still unclear and deserve further investigation.
2.2 Gut Microbiota Promotes Browning of WAT and Activity of BAT
2.2.1 Cold Exposure and Microbiota Depletion
It has been reported that beige adipocytes can be developed from white adipocytes through a process known as browning. Various stimulus, including cold temperature, β3-AR agonists, and circulating hormones, such as fibroblast growth factor 21(FGF21), can turn white adipocytes into beige adipocytes (11). Several studies have indicated that gut microbiota is a vital endogenous factor that regulates the browning of WAT and activation of BAT to adapt to external environment changes (38, 45)
Mestdagh et al. first showed that gut microbiota modulates the lipid metabolism in BAT. The absence of gut microbiota enhances BAT lipolysis and suppresses lipogenesis in GF mice, suggesting activated lipid catabolism in their BAT (38). In line with this study, another study has demonstrated that microbiota depletion, either by antibiotic treatment or in GF mice, promotes the functional beige adipocytes development in subcutaneous and perigonadal visceral adipose tissues. It increases the expression of brown fat markers, such as PPAR-γ coactivator 1a (Ppargc1a), Ucp1, and acyl-CoA synthetase long-chain family member 1 (Acsl1), and improves glucose tolerance and insulin sensitivity, while decreasing white adipose tissue mass in both genetically and diet-induced obese mice (46, 47). It has been proposed that eosinophil infiltration and enhancements of type 2 cytokine signaling and M2 macrophage polarization in the WAT, which are known to drive the process of browning, may contribute to the above metabolic improvement of the mice (46).
Previous research has indicated that cold exposure can notably change the gut microbiota composition by decreasing the richness of most gut microbiota phylum, especially Bacteroidetes, Proteobacteria, Tenericutes, Actinobacteria, Verrucomicrobia, and Cyanobacteria, while increasing Firmicutes, Deferribacteres, and Firmicutes versus Bacteroidetes ratios (47–49). Transplantation of the “cold microbiota” to GF mice can increases insulin sensitivity, cold tolerance as well as fat loss in the host. The expression of thermogenic genes is markedly elevated, especially Ucp1, Prdm16, Pgc-1α, and PPARγ in the BAT, as well as in the subcutaneous WAT (47), indicating WAT browning effects caused by “cold microbiota” transferring. In line with this, Zietak et al. observed a notable change in the gut microbiota composition at the family and phylum levels upon acute and long-term cold exposure. There were increased levels of Mogibacteriaceae, Ruminococcaceae, Adlercreutzia, and Desulfovibrio and reduced levels of Erysipelotrichaceae, Bacilli, and the genus rc4-4. Respectively, these genera are linked to leanness and obesity (49). GF mice that were fed a HFD at room temperature gained improved glucose tolerance and less adipose tissue, which is associated with increased BAT thermogenesis and a plasma bile acid profile (49). When rats were exposed to cold for 4h/day for 21 days, both subpopulations of Clostridiale and concentrations of butyric and isovaleric acid increased whereas Bifidobacteria decreased in the caecum (45). The Clostridiale subpopulation is the main producer of butyrate, particularly in Clostridial clusters IV and XIVa (45). These studies have further suggested that reducing ambient temperature can fight obesity by altering gut microbiota composition and promoting thermogenesis.
Cold exposure not only alters the gut microbiome, but also modulates bile acid synthesis pathway by up-regulating the expression of crucial enzyme cytochrome P450, polypeptide 1 (CYP7B1), family 7, and subfamily b in the liver. This sets off a metabolic process that integrates lipoprotein clarification in BAT and hepatic conversion of cholesterol to bile acids synthesis pathway, leading to increased hepatic synthesis and more fecal excretion of bile acids, which distinctly changes the gut microbiota composition and increases heat production (50). This study has identified a novel BAT-liver-gut axis in the regulation of energy metabolism. Furthermore, in a study of 12 healthy female subjects, oral supplementation of the chenodeoxycholic acid (CDCA) could increase BAT activity and whole-body energy expenditure (51).
AMPK, an essential nutrient sensor for maintaining cellular energy status, plays important role in regulating glucose metabolism in various tissues. Very recently, Huang et al. identified that intestinal AMPKα1 stimulates thermogenesis by modulating anti-microbial peptide that manipulates gut microbiota and metabolites. These intestine-BAT communications through AMPKα- related signaling might partially be the underlying mechanism of beneficiary action of metformin on the intestine (52).
However, a recent study by Li et al. has reported different results by showing that microbiota depletion via antibiotic treatment or in GF mice impairs the browning of WAT (53). They have reported that the Ucp1-dependent thermogenesis is blunted with the depletion of gut microbiota when exposed to cold stress, which effect can be partly rescued by butyrate indicating that this metabolite may play an important role in the normal thermogenic responses to cold temperature. The study has also shown that alternative macrophages, eosinophil, and type 2 cytokine signaling have no influence on thermogenesis and energy expenditure in GF mice (53, 54). The opposed results among different studies may be related to different backgrounds of gut microbiota compositions caused by different housing conditions, dietary nutrients, sex and age of rodents, antibiotic administration methods (drinking water or gavage), or other unknown factors that deserve further investigation in the future.
Although recent studies suggest that the gut microbiota is the key player in the regulation of energy balance and glucose homeostasis via thermogenesis, several studies have also shown that the ability of gut microbiota the maintenance of glucose homeostasis is independent of the manipulation of energy expenditure. By using commensal depleted (CD) and GF mice models, Krisko and colleagues have demonstrated that the gut microbiota seems disposable for both cold- and diet-induced thermogenesis and not required for the recruitment and activation of thermogenic tissues. The gut microbiota maintains blood glucose by regulating hepatic gluconeogenesis rather than adipose tissue in cold (55). However, in the absence of changes in energy expenditure, the gut microbiota seems to promote hepatic gluconeogenesis to maintain glucose homeostasis through the production of amino acid metabolites to facilitate hepatic tricarboxylic acid (TCA) cycle fluxes for gluconeogenesis (55), whose effect is independent of adaptive thermogenesis. A very recent study has found that gut microbiota depletion through antibiotic cocktail treatment promotes glucose uptake and clearance in BAT and cecum, whose effect is dissociated from adaptive thermogenesis (28).
Taken together, current studies have demonstrated that cold temperature and microbiome deletion or transplantation can decrease adiposity through the induction of WAT browning and BAT activity. Further studies on the mechanisms underlying these actions are needed to explore the relationship between gut microbiota and browning of WAT and activity of BAT.
2.2.2 Intermittent Fasting and Caloric Restriction
Intermittent fasting (IF) is known as an effective and useful strategy for weight loss and has multiple benefits for metabolism and health (56). Recent studies have shown that IF can induce WAT browning by altering gut microbiota composition. Li et al. reported that an every-other-day fasting (EODF) stimulates the browning of WAT, and greatly ameliorates obesity and associated hepatic steatosis and insulin resistance (57). These effects are linked to a change of the gut microbiota composition by increasing the Operational Taxonomic Unit (OTU) abundance of Firmicutes while decreasing most other phyla and, as a consequence, elevating serum levels of lactate and acetate (57). Both lactate and acetate have been reported to induce WAT browning, via the proton-linked monocarboxylate transporter 1 (MCT1) in WAT and BAT (58, 59). Consistently, transplantation of microbiota from IF mice to GF mice significantly upregulates inguinal WAT Ucp1 expression and increases small intestine length (57). Kim et al. thought IF promoted adipose thermogenesis via vascular endothelia growth factor (VEGF) expression in WAT and the expression of Adrb3, Cidea and Ucp1 were significantly elevated in HFD-IF mice, which might due to β-AR-dependent thermogenesis (60).
Similar to IF, caloric restriction (CR) also induces metabolic improvements and stimulates the browning within the subcutaneous and visceral adipose tissues (61). Change of microbiota not only contributes to increased activity of BAT and browning of WAT during CR, but also promotes metabolic improvements, including improved glucose tolerance, insulin sensitivity, and decreased fat gain (62). During CR, the most dramatic changes in the composition of gut microbiome are the increases in Erysipelotichaceae and Lactobacillaceae and decreases in other Firmicutes families, as well as increased levels of Bacteroidaceae and Verrucomicrobiaceae (62). In a study with 49 overweight and obese adults, increased Akkermansia muciniphila during CR has been shown to reduce adiposity and increase insulin sensitivity (63), which may contribute to the metabolic benefits of CR. Very recently, Li and colleagues discovered that CR could reshape the gut microbiota by elevating the ratio of Firmicutes to Bacteroidetes. In particular, Parabacteroides distasonis was significantly reduced (64). Parabacteroides distasonis is able to produce secondary bile acids (BAs) and is known to ameliorate weight regain (64).
In line with those observations from murine studies, data collected from patients also suggests a connection between microbiota and adipose tissue physiology in humans. By analyzing gut microbiota and browning gene markers in WAT from 34 morbidly obese subjects, Moreno et al. have found that, in people with obesity and insulin resistance, relative abundance (RA) of Firmicutes decreases, whereas compared with insulin sensitive subjects, Bacteroidetes and Proteobacteria RA increase (65). Interestingly, Firmicutes RA is positively linked to browning markers (Ucp1, Prdm16, and Dio2) in subcutaneous but not in visceral adipose tissue. Specifically, those bacteria belonging to the Ruminococcaceae family, are positively connected with plasma level of acetate, which is also associated with increased beige fat development and insulin sensitivity (65). A clinical trial based on 24 patients with metabolic syndrome demonstrated that 10-hour time-restricted eating decreased body weight, waist circumference, blood pressure, LDL cholesterol, hemoglobin A1C, and the risk of cardiovascular disease in 12 weeks (66). Very recently, a random clinical study with 139 patients found that CR and time-restricted eating have similar effects on reducing body weight, body fat, or metabolic risk factors (67). However, pharmacological means of inducing beiging and thermogenesis in humans by environmental (e.g., cold) or nutritional (e.g., IF, CR) manipulation and the roles of microbiota are still unknown and deserve further investigation.
In summary, the gut microbiota plays an essential role in whole-body energy metabolism by regulating thermogenic programs in different fat depots. Some environmental and nutrition situations (e.g., cold stress, fasting, or CR) could induce a shift of gut microbiota composition that stimulates browning of WAT and activity of BAT. However, the underlying mechanisms and pathways have not yet been fully identified.
2.3 Gut Microbiota and Inflammation of Adipose Tissues
Chronic low-grade inflammation is a trademark of many metabolic diseases, such as obesity, T2D, and NAFLD (68, 69). During obesity, the expanded adipose tissues develop hypoxia and release pro-inflammatory cytokines and chemokines, leading to the infiltration of immune cells (including macrophages, natural killer cells, neutrophils, and T cells) and secretion of pro-inflammatory factors such as tumor necrosis factor (TNF), interleukin 1beta (IL-1b), and IL-6 (70–72). A growing number of evidence suggests that gut microbiota play essential roles in adipose tissue inflammation in obesity and related disorders.
Changes in the abundance and diversity of microbiota are associated with inflammation in obesity. Using metagenomic approaches, Cotillard et al. demonstrated that people with 40% reduced microbial gene richness have more noticeable dysmetabolism and low-grade inflammation (73). An increase of gene richness and diversity is linked to a significant decrease in adipose tissues, circulating cholesterol, and inflammation. In addition, it is suggested that certain ‘pro-inflammatory’ bacterial strains, such as Ruminococcus gnavus or Bacteroides species, might prevail and certain ‘anti-inflammatory’ strains, such as Faecalibacterium prausnitzii, are less prevalent in obesity (14, 73, 74).
Not only does HFD feeding have profound impacts on gut microbiota composition, but it can also cause anatomical and functional changes of the intestinal barrier. Disruption of the intestinal barrier can cause low-grade inflammation in the small bowel and color locally. And some bacteria products such as LPS could leak into plasma inducing systemic inflammation (75). Studies in animals and humans have demonstrated that circulating LPS levels are one of the key elements linking the gut microbiota and inflammation of adipose tissues. Cani et al. found that HFD increases the fraction of an LPS containing microbiota in the gut accompanied with increased F4/80-positive cells and markers genes of inflammation in WAT. LPSCD14 system participates in insulin resistance and the onset of diabetes and obesity (76).
It has been hypothesized that LPS engages in the inflammatory process in adipose tissue during obesity (77). On the one hand, LPS is involved in the transition of the M2 to the M1 macrophages, the latter which causes inflammation responses of adipose tissues; on the other hand, LPS may activate caspase-4/5/11signaling, which induces pyroptosis, an inflammatory kind of programmed cell death of adipocytes. The dead adipocyte remnants and macrophages compose a morphological inflammation marker called crown-like structure in adipose tissue (78). In addition, LPS can activate receptor TLR4 on adipocytes and stimulate pro-inflammatory pathways. The infiltration of macrophages and the up-regulation of TNF-α, NF-κB, and IL-6in turn promote adipose tissue inflammation (79, 80). Also, the LPS-activated TLR leads to the downstream assembly of a complex of LPS-binding proteins such as CD14, LBP, and myeloid differentiation factor-2 (MD-2), leading to the formation of the activated (TLR4–MD-2–LPS) complex (74, 81). Orret al. demonstrated that global TRL4 deficiency could reduce body fat gain slightly, reduce liver TG and promote M2 polarization of macrophages in adipose tissues after HFD (82). Additionally, it has also been demonstrated that CD36 knockout mice fed with HFD showed reduced adipose tissue inflammation and suppressed pro-inflammatory cytokine response when exposed ex vivo to LPS, indicating that CD36 has an important impact on TLR signaling pathway (83). And gut microbiota can also produce some metabolites to reduce LPS-associated inflammation, such as SCFA, polyamines, and aryl hydrocarbon receptor (AHR) ligands (84). In many animal studies, an SCFA butyrate has been thought to act as an anti-inflammation metabolite (83) to block the translocation of LPS in the intestines, thus ameliorating LPS-induced pro-inflammatory effects (85, 86).
Previous studies have shown that probiotic strains can suppress adipose low-grade chronic inflammation by modulating the host’s immune responses (87). Lactic acid bacteria (LAB) strains can suppress chronic inflammation in adipose tissues by regulating the secretion of adipokines (73). Lactobacillus casei CRL431 administration decreased inflammatory cytokines, includingIL-6, TNF-α, and MCP-1, in adipocytes and macrophages in diet-induced obesity (74, 88). Additionally, evidence collected from in vitro (89) and in vivo (90, 91) studies have shown that lactic acid bacteria, especially strains of Lactobacillus and Bifidobacterium genera present anti-inflammatory properties in adipose tissue (91). The mucin-degrading bacterium Akkermansia muciniphila has anti-obesogenic effects in mice and humans (63) and treatment of HFD-fed mice with A. muciniphila attenuated visceral WAT inflammation by reducing the levels of pro-inflammatory cytokines, including IL-6 and IL-1β, and increasing the number of regulatory T cells (Tregs) (92). Faecalibacterium prausnitziiare is an important butyrate producing bacteria and plays critical roles in gut homeostasis. Xu et al. have demonstrated that, under diabetic condition, F. prausnitzii produced anti-inflammatory molecules and restored the intestinal barrier structure via increasing the expression of tight conjunction protein ZO-1in humans (93). Oral administration of F. prausnitzii markedly reduced the inflammation of colon by blocking NF-κB activation and IL-8 production (94). F. prausnitzii may therefore serve as a biomarker to diagnose gut related diseases and metabolic diseases. Due to their potential benefits on health, probiotics have been used in food and health care medicines (95). Parabacteroides distasonis has been identified to play a protective role in obesity (96). Xu et al. found that with the treatment of Panax notoginseng saponins (PNS), a traditional Chinese medicine, the obese mice had a higher AR of P. distasonis and A.muciniphila and lower body weight and fat mass (97).
Interestingly, dietary lipids can also influence adipose tissue inflammation. Unsaturated lipids were reported to decrease body weight gain, increase Firmicutes to Bacteroidetes ratio and promote a healthier metabolic phenotype, while saturated lipids aggravate WAT inflammation and promote a higher degree of obesity, which is partly attributed to distinct gut microbiota richness and diversity (24, 98). Mechanistically, gut microbial-derived factors increase chemokine CCL2 expression in adipocytes, which buildups macrophage accumulation and inflammation in WAT through TLR4, MyD88, and TRIF related pathways (98). In line with these studies, Tran et al. found that consumption of western-style diet (WSD), a low-fiber and high-fat diet, prompts adiposity and adipose inflammation characterized by increased M1to M2 macrophage ratio and proinflammatory cytokine expression. Microbiota ablation suppresses macrophage infiltration and M1 macrophage polarization, as a result, decreasing inflammation in epididymal WAT (24, 99). The strategies aimed to maintain gut microbiota balance, reduce LPS, or block TLR4 signaling, may be useful in reducing adipose tissue inflammation and insulin-resistance in obesity.
It has been aware that interactions between host genetics, diet, and gut microbiota contribute to the development of obesity and metabolic syndrome (25, 26). Ussar and colleagues found that depending on the different genetic backgrounds of the mouse strains, HFD and other environmental factors might produce different changes in gut microbiota, had different impacts on adipose tissue inflammation, and created different interactions between the microbiome and the mouse models, as a result, leading to different susceptibility to obesity, hepatosteatosis, insulin resistance and other components of metabolic syndrome (100).
3 Factors Mediate the Cross-Talk Between Gut Microbiota and Adipose Tissues
It is well aware that the human gut microbiota composition can be influenced by gender, age, ethnicity, and geography (20, 21). Recent studies suggest that, although gut microbiota has a profound influence on the homeostasis of adipose tissues, the latter in turn can also affect the composition of gut microbiota via metabolites and cytokines.
3.1 Adipokines
Adipose tissues not only serve as energy storage but also as important endocrine organs capable of secreting lots of biologically active compounds that modulate whole body energy balance. The adipose tissues secrete bioactive peptides, referred to as adipokines, including leptin, adiponectin, retinol-binding protein 4 (RBP4), bone morphogenetic protein (BMP)-4, BMP-7, dipeptidyl peptidase 4 (DPP-4), vaspin, apelin, and progranulin (101). At a systemic level, adipokines control multiple biological processes in target organs, including adipose tissues liver, muscle, vasculature, heart, brain and pancreas, immune system, and many others (101). Several studies have proposed that the gut microbiota is a critical environmental factor in the modulation of the adipokine profiles.
3.1.1 Leptin
Leptin plays an important role in the regulation of food intake, satiety, appetite, and energy expenditure (102, 103). Studies have shown that microbiota transplantation to GF mice increases fat mass with a higher production of leptin level, and this increase in leptin is proportional to the increase in body fat (104). Yao et al. found that leptin expression in GF mice increases with more CpG sites hypermethylated at the leptin promoter compared with conventional mice (103). High circulatory leptin could induce leptin resistance. Erik et al. found compared with GF mice, conventionally raised mice have reduced leptin sensitivity, as leptin treatment causes much less reduction in body weight and lower expression of the orexigenic peptides neuropeptide-Y (Npy) and agouti-related protein (Agrp). And leptin treatment increased leptin resistance-associated suppressors of cytokine signaling 3 (Socs-3) in the hypothalamus in conventional mice (105). These results indicate that the gut microbiota-associated increase of Socs3 might lead to leptin resistance and obesity in the conventional mice (105). Everard and colleagues have reported that prebiotic treatment is able to restore leptin sensitivity and improve metabolic parameters in HFD induced obese mice (106). Similar to those observations, prebiotic feeding decreases adiposity and increases plasma GLP-1 levels s in the ob/ob mice (106). Recently a randomized clinical trial suggested 12 weeks of probiotic administration significantly improved metabolic phenotypes and decreased leptin level compared with baseline values in obese women (107). However, whether and how gut microbiota mediate changes of leptin sensitivity/resistance in health and obesity are still unclear.
On the other hand, leptin may also have an impact on the gut microbiota. A daily supplement of leptin causes a higher proportion of Clostridium genus and a lower relative abundance of Sutterella, and enhances the expressions of interferon-α (TNF-α), mucin (MUC-2, MUC-3) during suckling period of rats, indicating a modulator role of leptin on the intestinal activation (108).
3.1.2 Adiponectin
As another abundant adipokine, adiponectin also has a bilateral relationship with gut microbiota. Li and his colleagues have found that changes in gut microbiota are closely associated with increased plasma adiponectin levels, adiponectin-receptor-gene (AdipoR1 and AdipoR2), activated AMPK signal, and upregulated anti-obesity effects (109). Yao et al. found that alteration of the gut microbiota via antibiotics not only inhibits body weight gain, increases the expression of lipid oxidation and thermogenesis genes (including Pparα, Pgc-1α, Atgl) in adipose tissues, but also increases mRNA level of adiponectin (110). In addition, antibiotics reduces DNA methylation fractions of adiponectin promoters and down-regulate the level of DNA methyltransferase 1 (DNMT1) and 3a(DNMT3a) in HFD mice (110). Also, Membrez et al. found that microbiota depletion with norfloxacin and ampicillin could induce a higher circulating level of adiponectin while a lower level of LPS (111).
Adiponectin can influence the species richness of gut microbiota. Animals with daily adiponectin supplementation led to a lower relative abundance of Proteobacteria phylum, Blautia and Roseburiagenus, and a higher proportion of the Enterococcus genus. Exogenous administration of adiponectin can increase the percentage of Tc TCRαβ+ and NKT cells and decrease the NK cells in intraepithelial lymphocytes (IEL) (108). Additionally, suckling period supplementation with adiponectin alters gut microbiota and enhances functions of the immune system (108).
3.1.3 Fibroblast Growth Factor 21 (FGF21)
FGF21 is a member of the FGF superfamily and an important metabolic regulator, which is produced by adipose tissue, liver, and skeletal muscle (112, 113). Liver is considered as the major source of the endocrine FGF21 that circulates in the blood and regulates energy expenditure and insulin sensitivity (114). Recent studies indicated that JNK in adipose tissues could regulate adiponectin and hepatic FGF21 expression thus promoting a feed-forward regulatory loop to increase the crosstalk in different organs (115). Martin et al. discovered that gut microbiota mediates the hepatic FGF21 response to protein-restriction. During long-term dietary protein-restriction, the gut microbiota undergoes metabolic adaptations that stimulate hepatic FGF21 adaptive metabolic pathways (113). In the absence of gut microbiota, FGF21 is de-sensitized to the effects of protein-restriction (116). Additionally, changes in gut microbiome increase hepatic FGF21 expression and circulatory levels, which might be triggered by phosphorylation activation of eIF2αvia GCN2 (116, 117).
Ketogenic diet (KD) or carbohydrate-restricted diet can shift the gut microbiota toward “favorable bacteria” (i.e., A. muciniphila, Lactobacillus) and regulate lipid metabolism through PPARα/FGF21 pathway (117). Though short time KD enhances hepatic fatty oxidation and gluconeogenesis, long-term KD impairs FGF21 signaling, leading to glucose intolerance, insulin resistance, and macrophage infiltration, and steatosis in the liver (117, 118). A short time of carbohydrate-restricted diet consumption has been considered as a healthier lifestyle, while the underline mechanisms and debatable efficacy of this diet need more studies (22).
3.1.4 Apelin
Apelin, a 36 amino-acid peptide, is the endogenous ligand of the G protein-coupled receptor APLNR. As one of the key hormones produced by adipose tissues, it has been demonstrated to modulate glucose homeostasis via AMPK and nitric oxide (NO)-dependent mechanisms (99). It contributes to regulate whole body well-being, such as blood pressure, cardiovascular and fluid homeostasis, lipid metabolism, food consumption, cellular proliferation, and angiogenesis (119). Studies have found that, compared with lean subjects, plasma apelin concentrations increase in obese subjects and in hyper-insulinemic obese mice (120). In type 2 diabetic mice, altered gut microbiota composition, e.g., higher abundance of Firmicutes, Proteobacteria, and Fibrobacteres phyla, regulates expression of apelin/APJ in adipose tissue through low-grade inflammatory tone and eCB system (121), indicating definite relationships between the gut microbiota and the apelin system. While the distinct roles of different bacteria in the development of diabetes are yet to be determined.
Taken together these findings suggest a close relationship between gut microbiota and the regulation of the adipokines in both physiological and pathophysiological conditions. However, the mechanisms behind these relationships require further studies.
3.2 Lipopolysaccharide
Gut microbiota-derived LPS has been defined as one of the key factors involved in the initiation and development of inflammation and obesity related metabolic disorders (122). In response to HFD feeding, the production and transportation of LPS from the gut lumen toward target tissues can induce increased plasma LPS levels, resulting in metabolic endotoxemia and low-grade inflammation. Specifically, HFD causes gut microbiota-dependent impairment of the tight junction proteins, such as occludin, occludens-1, and zonula, which are important for the gut barrier function. The antibiotic treatment abolishes diet-induced gut permeability, diminishes endotoxemia absorption, and lowers inflammation and glucose intolerance (123, 124). It has been established that, compared with controls, obese individuals have higher plasma levels of LPS, which were closely associated with intra-abdominal adipose tissue mass but only moderately correlated with subcutaneous fat mass (125). Consistently, both plasma LPS levels and the LPS-containing microbiota in the gut were dramatically induced by a 4-week HFD feeding. Continuous subcutaneous infusion of LPS for 4 weeks in mice presented a similar phenotype in HFD fed mice and increased the expression of inflammation markers in adipose tissues by the LPS/CD14/TLR4 system or TLR4/MD-2 complex (76, 81). Hersoug et al. has proposed that scavenger receptor class B type 1 (SR-BI) binds to both lipids and LPS and stimulates LPS transfer to other lipoproteins with the help of translocases. LPS can induce transcytosis of lipoproteins over the endothelial barrier and endocytosis in adipocytes by felicitating the SR-BI binding (126). Large sizes of adipocytes with higher metabolic activity absorb more LPS-rich lipoproteins, which may contribute to the M2 to M1macrophages polarization, and as a result, increase LPS delivery into the hypertrophic adipose tissue (126).
3.3 Short-Chain Fatty Acids
SCFA, including acetate, butyrate, and propionate (which make up more than 95% of the SCFA content), are extracted from gut microbial fermentation of foods that cannot be digested due to the lack of appropriate enzymes (127). SCFA can be largely absorbed by colonic epithelium, used as energy resources, and play a vital role in the maintenance of gut health and metabolism homeostasis.
SCFA produced through fiber fermentation in the colonic region is known to regulate glucose and lipid metabolism. On the one hand, SCFA might increase intestinal energy harvesting capabilities and induce obesity. Fecal SCFA concentration has been reported to increase in ob/ob mice or obese humans. In ob/ob mice, increased Firmicutes to Bacteroidetes ratio is connected to increased caecal concentrations of acetate and butyrate or acetate and propionate respectively (128). In HFD fed rats, by using 13C-acetate labeled method to measure tissue acetate concentrations, Perry and his colleagues found that increased production of acetate by gut microbiota could activate parasympathetic nervous system and increase glucose-stimulated insulin secretion, hyperphagia, and obesity (129). Individuals with obesity have a higher concentration of SCFA in fecal compared with lean controls, who have significantly higher fecal concentrations of the genus Bacteroidesbut and lower Ruminococcus flavefaciens and Bifidobacterium (130), suggesting that SCFA metabolism might act as an essential factor in obesity.
However, on the other hand, studies have also found that SCFA increases energy expenditure and can reduce or reverse body weight gain and adiposity (131, 132). Lu et al. has demonstrated that supplementation of SCFA admixtures could change the composition of gut microbiota and reduce body weight by enhancing triglyceride hydrolysis and FFA oxidation in the adipose tissue, stimulating mitochondrial biogenesis and beige adipogenesis, and suppressing chronic inflammation (132). Oral supplementation of sodium butyrate leads to body weight loss and improves fasting blood glucose and insulin tolerance of obese mice by increasing energy expenditure and fat oxidation (133). Butyrate administration increases mitochondrial function and biogenesis and elevates AMPK and p38 activities via a PPARγ-dependent pathway in the BAT (133, 134). Furthermore, supplementation of acetate can result in an increase in GLP-1 and PYY, appetite suppression, and hypothalamic neuronal activation patterning, thereby reducing body weight, food intake, and fat mass (135).
SCFA can directly act on adipose tissues via G protein coupled receptor 41 and 43 (GPR41 and GPR43) dependent manner. By inhibiting intracellular lipolysis (mainly through acetate and propionate) and increasing the lipid buffering capacity of adipose tissues (mainly by propionate), SCFA can lead to a reduction of lipid overflow and decrease of ectopic fat accumulation, thereby positively regulating insulin sensitivity (132, 136). SCFA might be also involved in adipogenesis via GPR43 in rodent adipocytes. Hong et al. found expression of GPR43 was up-regulated in adipose tissues of HFD mice and also in differentiated 3T3-L1 adipocytes (137, 138). And both propionate and acetate can elevate GPR43 expression with up-regulation of PPAR-γ2 and inhibit lipolysis in a dose-dependent manner (138).
SCFA might also mediate cold exposure induced adipose tissue thermogenesis. Cold exposure alters SCFA production while some SCFA including acetate and propionate were decreased in mice after antibiotics (ABX) treatment, however, butyrate was not altered and, under cold exposure, Lachnospiraceae, which belongs to butyrate producing bacteria Clostridia, was increased (53). Butyrate sodium administration to antibiotics treat mice can increase their body temperature and Ucp1 expression in BAT and subcutaneous WAT. Using the isotope labeling method to trace12C/13C butyrate, it has been reported that butyrate functions through the gut-brain axis instead of having a direct effect on adipose tissues (53, 63). Butyrate could reduce appetite and activate BAT via suppressing the activity of orexigenic neurons and the expression of NPY (139). Cold microbiota transplantation can also result in significant changes in SCFA, increasing the concentration of acetic acid, butyric acid, isobutyric acid, and isovaleric acid. Besides, cold microbiota transplantation can activate the β3-adrenoceptor-cAMP-PKA signaling pathway (55), indicating that gut microbiota can activate the NE system in vivo, probably through microbiota metabolite SCFA.
3.4 Bile Acids
Secreted in the liver from cholesterol, bile acids are metabolized in the intestine through the gut microbiota. Many researchers have proven that gut microbiota can regulate the composition of bile acids. In GF mice, the bile acid pool is primarily comprised of conjugated bile acids (140). The bile acids function via the nuclear Farnesoid X receptor (FXR) and the G protein-coupled membrane receptor 5 (GTR5), which are involved in numerous metabolic pathways in the host (140, 141).
Past research that has used hepatic and intestinal disruption of the FXR have demonstrated that the liver and the intestine both acts as central organs for FXR signaling-dependent glucose and lipid control. FXR deficiency can reduce body weight gain and adipose tissue mass, and improve glucose homeostasis and insulin sensitivity in HFD mice (142). Specific intestinal FXR activation protected against the development of obesity through increased thermogenesis and WAT browning (142, 143). Another bile acid-responsive receptor is TGR5, or, the G protein bile acid activated receptor (GPBAR)-1, regulates glucose homeostasis by bolstering energy expenditure in BAT and in muscle and enhancing GLP-1 release in intestinal L cells (144).
Cold exposure can change the composition of bile acid pool by inducing hepatic enzymes involved in the conversion of cholesterol to primary bile acids (49), which, in turn, may mediate the action of cold exposure on the shape of the gut microbiome and adaptive thermogenesis promotion. Genetic and pharmacological interventions that targeted CYP7B1 (cytochrome P450, family 7, subfamily b, polypeptide 1), the key enzyme of synthesis and biliary excretion of bile acids, have hindered the rise in fecal bile acid excretion, altered the bacterial composition of the gut and, more importantly, regulated thermogenic responses, implying that bile acids may play a role as crucial metabolic effectors under sustained BAT activation (50).
As mentioned above, the diet was closely associated with gut microbiota and bile acids pool (64, 142). Wang et al. found that HFD significantly increased the deoxycholic acid (DCA) content in faces and administration of DCA promoted M1 macrophage polarization via NF-κB/ERK/JNK signaling (145). Li and colleagues have reported that compared with normal diet, CR decreased the conjugated non-12α-hydroxylated (12OH) BAs, such as tauro-β-muricholic acid (TβMCA), tauro-ω-muricholic acid (TωMCA), tauroursodeoxycholic acid (TUDCA), and taurochenodeoxycholic acid (TCDCA) (64). Interestingly, the gut microbiota will shift towards favoring bacteria that can harvest energy more efficiently during CR. When coming back to a normal diet or high-fat diet, gut microbiota will be reshaped, and the abundance of parabacteroides distasonis and 12OH BAs for efficient fat adsorption increase significantly, leading to overweight again (64), suggesting that BAs are important mediators for gut microbiota regulated body weight.
3.5 Endocannabinoid System
The eCB system is comprised of endogenous lipids that trigger specific G protein-coupled receptors termed cannabinoid receptors 1 and 2 (CB1 and CB2). It has been demonstrated that the eCB system consists of more than 100 lipid mediators and 50 proteins, such as CB1, CB2, N-arachidonoyl-ethanolamine (AEA), 2-arachidonoyi-glycerol (2-AG), and the anabolic and catabolic enzymes for the endocannabinoids (146, 147). The eCB system has the ability to regulate gut-barrier function, gut permeability, and metabolic endotoxemia in obesity, and control energy balance by manipulating the metabolic process, energy intake and fat accumulation in adipocytes (148–150). The eCB also boosts fat storage in adipocytes by inducing adipogenesis and triacylglyceride production. The CB1 inhibits the activity of AMPK and reduces the AMPK-associated lipolysis (148). Geruts et al. has demonstrated that db/db mice displayed increased eCB signals in adipose tissues, and apelin and APJ expression were decreased by the eCB system under normal conditions (121). The activation of its specific receptor CB1R in mice stimulates the expression of PPARγ and LPL in WAT and increases the expression of genes involved in adipocyte differentiation, including PPARγ, C/EBPα, AP2, and genes involved in lipogenesis, such as SREBP-1c, ACC, FAS markedly (150, 151).
Chronic CB1 antagonism promotes body weight loss in diet-induced obese mice by increasing energy expenditure insulin-stimulated glucose application and significant stimulation of BAT activation (152). Paola et al. found chronic N-oleoyl-ethanolamine (OEA) administration to mice in normal diet could shift Firmicutes/Bacteroides ratio, decrease Lactobacillus, and reduce intestinal cytokines expression (153). In obese humans, supplementation of OEA decreased BMI and waist circumference, which might due to the activation of PPARα (147). And the administration of palmitoyl ethanolmaide (PEA), which is a member of N-acyl ethanolamide (NAE) similar to AEA, could decrease body weight, food intake, and fat mass in HFD rats (154). So the design and development of eCB system agonists, antagonists, and allosteric modulators may represent a therapeutic way for gut dysbiosis and related metabolism diseases. However, the mechanism behind this is largely unclear.
4 Conclusion Remarks and Future Perspective
Recent research has indicated that the gut microbiota can contribute to host metabolism by cross-talk with adipose tissues. On the one hand, the gut microbiota modulates adipogenesis and energy expenditure and alleviates obesity by promoting the browning of WAT and the activity of BAT. On the other hand, microbial-derived metabolites, such as SCFA and LPS, may act as the central factors in the pathogenesis of adipose inflammation, insulin resistance, and obesity. Additionally, the gut microbiota is a crucial environmental factor in adipokine profile regulation, which, in turn, may mediate the functional modulation of adipose tissues to the microbiota (Figure 1).
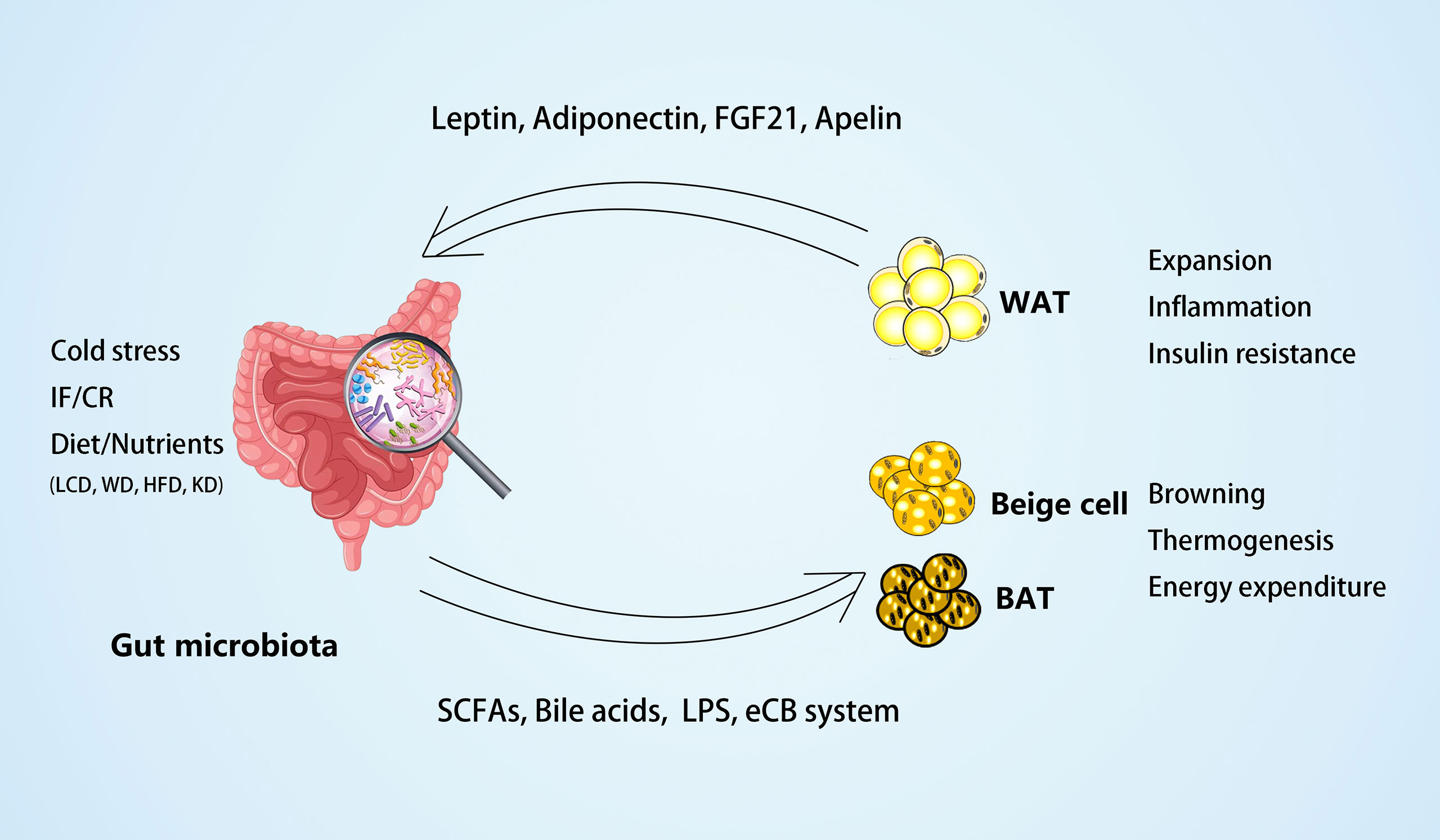
Figure 1 The crosstalk between gut microbiota and adipose tissues. On the one hand, the gut microbiota and microbial-derived metabolites may mediate the external factors, such as dietary foods, to regulate white adipose tissue (WAT) expansion, inflammation, and insulin resistance in obesity. Different diets may have different effects on the gut microbiota, such as low carbohydrate diet (LCD) (22) can increase Bacteroidaceae Bacteroides and reduce body weight effectively; whereas western diet (WD) (24) or high-fat diet (HFD) (43, 76) can increase dysbiosis of gut microbiota and inflammation of adipose tissues; and ketogenic diet (KD) (117) can increase A. muciniphila, Lactobacillus and regulate lipid metabolism through PPARα/FGF21 pathway. On the other hand, the gut microbiota and its metabolites may mediate environmental temperature, intermittent fasting (IF), or caloric restriction (CR) in the process of energy expenditure by promoting browning and thermogenesis of beige and brown adipose tissue (BAT). In the meantime, some adipokines, including leptin, adiponectin, FGF21, and apelin, produced by adipose tissues can act on the gut microbiota to modulate its composition.
Although a number of inconsistent findings have been reported, perhaps as a result of differences in mice strain, housing conditions, or diet, current studies have shown changes of gut microbiota composition, specific metabolites and some exogenous substances have impacts on modulating WAT browning, BAT activity, and thermogenesis. However, the mechanisms underlying the relationship between gut microbiota changes and browning of WAT or BAT activity were still unclear and, more importantly, evidence collected from studies in humans is needed to be verified. In addition, despite these associations, the causal pathways and the mechanisms underlying the relations between gut microbiota and adipose tissues in inflammation and risks of obesity associated diseases have yet to be fully classified.
Further characterization of the stimulators of a steady, low-grade, inflammatory response in people with obesity might provide different evidence for novel intervention methods to help to alleviate the risk of obesity and related disorders. These changes would help to guide the clinical application of LCD to overweight and obese patients (22). Additionally, due to their beneficial effects on the host, some probiotic bacteria have been widely used as a health care product. For example, Bifidobacterium genera has been added to many foods and medicines to regulate gut microbiota and metabolism. Some others, such as Lactobacillus paracasei (42, 44). Akkermansia muciniphila (63), Faecalibacterium prausnitziiare, Parabacteroides distasonis and Clostridium leptum (94–97) have been reported to improve insulin sensitivity and alleviate the inflammation of adipocytes. Also, some microbial-derived metabolites such as certain SCFA and bile acids, may provide enlightenments to treat obesity. And after 3-month treatment of berberine (BBR), the baseline of Alistipes and Blautia changed that was closely associated with altered serum lipids levels (155). And metformin treatment significantly altered microbiota composition and its metabolites, including decreased Roseburia faecis and Roseburia intestinalis and increased butyrate, acetate and valerate (156). These studies implied that monitoring gut microbiota might be a potential way to predict the pharmacotherapeutic efficacy of some medicine. In the future, targeting the gut microbiota and their metabolites may provide a promising approach for therapeutic interventions and treatment of obesity and related metabolic diseases.
Author Contributions
DW performed the literature search and prepared the original draft, HW & LX performed the literature search and edited the manuscript, and FH had the idea for the article and critically revised the work. All authors read and approved the final manuscript.
Funding
This work was supported by grants from National Nature Science Foundation of China (91957113& 31871180), National Key R&D Program of China (2020YFA0803604), and Natural Science Foundation of Hunan Province (2019JJ40410) to F.H.
Conflict of Interest
The authors declare that the research was conducted in the absence of any commercial or financial relationships that could be construed as a potential conflict of interest.
Publisher’s Note
All claims expressed in this article are solely those of the authors and do not necessarily represent those of their affiliated organizations, or those of the publisher, the editors and the reviewers. Any product that may be evaluated in this article, or claim that may be made by its manufacturer, is not guaranteed or endorsed by the publisher.
References
1. Gadde KM, Martin CK, Berthoud HR, Heymsfield SB. Obesity: Pathophysiology and Management. J Am Coll Cardiol (2018) 71(1):69–84. doi: 10.1016/j.jacc.2017.11.011
2. Rohm TV, Meier DT, Olefsky JM, Donath MY. Inflammation in Obesity, Diabetes, and Related Disorders. Immunity (2022) 55(1):31–55. doi: 10.1016/j.immuni.2021.12.013
3. Loos RJ, Yeo GS. The Bigger Picture of FTO: The First GWAS-Identified Obesity Gene. Nat Rev Endocrinol (2014) 10(1):51–61. doi: 10.1038/nrendo.2013.227
4. Yupanqui-Lozno H, Bastarrachea RA, Yupanqui-Velazco ME, Alvarez-Jaramillo M, Medina-Méndez E, Giraldo-Peña AP, et al. Congenital Leptin Deficiency and Leptin Gene Missense Mutation Found in Two Colombian Sisters With Severe Obesity. Genes (Basel) (2019) 10(5):342. doi: 10.3390/genes10050342
5. Kleinendorst L, Abawi O, van der Kamp HJ, Alders M, Meijers-Heijboer HEJ, van Rossum EFC, et al. Leptin Receptor Deficiency: A Systematic Literature Review and Prevalence Estimation Based on Population Genetics. Eur J Endocrinol (2020) 182(1):47–56. doi: 10.1530/EJE-19-0678
6. Qin Y, Havulinna AS, Liu Y, Jousilahti P, Ritchie SC, Tokolyi A, et al. Combined Effects of Host Genetics and Diet on Human Gut Microbiota and Incident Disease in a Single Population Cohort. Nat Genet (2022) 54(2):134–42. doi: 10.1038/s41588-021-00991-z
7. Bagchi DP, MacDougald OA. Identification and Dissection of Diverse Mouse Adipose Depots. J Vis Exp (2019) 11:(149). doi: 10.3791/59499
8. Scheele C, Wolfrum C. Brown Adipose Crosstalk in Tissue Plasticity and Human Metabolism. Endocr Rev (2020) 41(1):53–65. doi: 10.1210/endrev/bnz007
9. Cannon B, Nedergaard J. Brown Adipose Tissue: Function and Physiological Significance. Physiol Rev (2004) 84(1):277–359. doi: 10.1152/physrev.00015.2003
10. Wu J, Boström P, Sparks LM, Ye L, Choi JH, Giang AH, et al. Beige Adipocytes are a Distinct Type of Thermogenic Fat Cell in Mouse and Human. Cell (2012) 150(2):366–76. doi: 10.1016/j.cell.2012.05.016
11. Harms M, Seale P. Brown and Beige Fat: Development, Function and Therapeutic Potential. Nat Med (2013) 19(10):1252–63. doi: 10.1038/nm.3361
12. Sebastián-Domingo JJ, Sánchez-Sánchez C. From the Intestinal Flora to the Microbiome. Rev Esp Enferm Dig (2018) 110(1):51–6. doi: 10.17235/reed.2017.4947/2017
13. Adak A, Khan MR. An Insight Into Gut Microbiota and its Functionalities. Cell Mol Life Sci (2019) 76(3):473–93. doi: 10.1007/s00018-018-2943-4
14. Bäckhed F, Ding H, Wang T, Hooper LV, Koh GY, Nagy A, et al. The Gut Microbiota as an Environmental Factor That Regulates Fat Storage. Proc Natl Acad Sci U S A (2004) 101(44):15718–23. doi: 10.1073/pnas.0407076101
15. Ley RE, Bäckhed F, Turnbaugh P, Lozupone CA, Knight RD, Gordon JI. Obesity Alters Gut Microbial Ecology. Proc Natl Acad Sci U S A (2005) 102(31):11070–5. doi: 10.1073/pnas.0504978102
16. Ley RE, Turnbaugh PJ, Klein S, Gordon JI. Microbial Ecology: Human Gut Microbes Associated With Obesity. Nature (2006) 444(7122):1022–3. doi: 10.1038/4441022a
17. Cox AJ, West NP, Cripps AW. Obesity, Inflammation, and the Gut Microbiota. Lancet Diabetes Endocrinol (2015) 3(3):207–15. doi: 10.1016/S2213-8587(14)70134-2
18. Romaní-Pérez M, Bullich-Vilarrubias C, López-Almela I, Liébana-García R, Olivares M, Sanz Y. The Microbiota and the Gut-Brain Axis in Controlling Food Intake and Energy Homeostasis. Int J Mol Sci (2021) 22(11):5830. doi: 10.3390/ijms22115830
19. Rosenbaum M, Knight R, Leibel RL. The Gut Microbiota in Human Energy Homeostasis and Obesity. Trends Endocrinol Metab (2015) 26(9):493–501. doi: 10.1016/j.tem.2015.07.002
20. He Y, Wu W, Zheng HM, Li P, McDonald D, Sheng HF, et al. Regional Variation Limits Applications of Healthy Gut Microbiome Reference Ranges and Disease Models. Nat Med (2018) 24(10):1532–5. doi: 10.1038/s41591-018-0164-x
21. Stanislawski MA, Dabelea D, Lange LA, Wagner BD, Lozupone CA. Gut Microbiota Phenotypes of Obesity. NPJ Biofilms Microbiomes (2019) 5(1):18. doi: 10.1038/s41522-019-0091-8
22. Zhang S, Wu P, Tian Ye, Liu B, Huang L, Liu Z, et al. Gut Microbiota Serves a Predictable Outcome of Short-Term Low-Carbohydrate Diet (LCD) Intervention for Patients With Obesity. Microbiol Spectr (2021) 9(2):e0022321. doi: 10.1128/Spectrum.00223-21
23. Avirineni BS, Singh A, Zapata RC, Stevens RD, Phillips CD, Chelikani PK. Diets Containing Egg or Whey Protein and Inulin Fiber Improve Energy Balance and Modulate Gut Microbiota in Exercising Obese Rats. Mol Nutr Food Res (2022) 66(7):e2100653. doi: 10.1002/mnfr.202100653
24. Tran HQ, Bretin A, Adeshirlarijaney A, Yeoh BS, Vijay-Kumar M, Zou J, et al. “Western Diet”-Induced Adipose Inflammation Requires a Complex Gut Microbiota. Cell Mol Gastroenterol Hepatol (2020) 9(2):313–33. doi: 10.1016/j.jcmgh.2019.09.009
25. Ussar S, Fujisaka S, Kahn CR. Interactions Between Host Genetics and Gut Microbiome in Diabetes and Metabolic Syndrome. Mol Metab (2016) 5(9):795–803. doi: 10.1016/j.molmet.2016.07.004
26. Singh A, Zapata RC, Pezeshki A, Workentine ML, Chelikani PK. Host Genetics and Diet Composition Interact to Modulate Gut Microbiota and Predisposition to Metabolic Syndrome in Spontaneously Hypertensive Stroke-Prone Rats. FASEB J (2019) 33(6):6748–66. doi: 10.1096/fj.201801627RRR
27. Tripathi A, Debelius J, Brenner DA, Karin M, Loomba R, Schnabl B, et al. The Gut-Liver Axis and the Intersection With the Microbiome. Nat Rev Gastroenterol Hepatol (2018) 15(7):397–411. doi: 10.1038/s41575-018-0011-z
28. Li M, Li L, Li B, Hambly C, Wang G, Wu Y, et al. Brown Adipose Tissue is the Key Depot for Glucose Clearance in Microbiota Depleted Mice. Nat Commun (2021) 12(1):4725. doi: 10.1038/s41467-021-24659-8
29. Canfora EE, Meex RCR, Venema K, Blaak EE. Gut Microbial Metabolites in Obesity, NAFLD and T2DM. Nat Rev Endocrinol (2019) 15(5):261–73. doi: 10.1038/s41574-019-0156-z
30. Turnbaugh PJ, Ley RE, Mahowald MA, Magrini V, Mardis ER, Gordon JI. An Obesity-Associated Gut Microbiome With Increased Capacity for Energy Harvest. Nature (2006) 444(7122):1027–31. doi: 10.1038/nature05414
31. Kübeck R, Bonet-Ripoll C, Hoffmann C, Walker A, Muller VM, Schuppel VL, et al. Dietary Fat and Gut Microbiota Interactions Determine Diet-Induced Obesity in Mice. Mol Metab (2016) 5(12):1162–74. doi: 10.1016/j.molmet.2016.10.001
32. Bäckhed F, Manchester JK, Semenkovich CF, Gordon JI. Mechanisms Underlying the Resistance to Diet-Induced Obesity in Germ-Free Mice. Proc Natl Acad Sci U S A (2007) 104(3):979–84. doi: 10.1073/pnas.0605374104
33. Gomes AC, Hoffmann C, Mota JF. The Human Gut Microbiota: Metabolism and Perspective in Obesity. Gut Microbes (2018) 9(4):308–25. doi: 10.1080/19490976.2018.1465157
34. Fu J, Bonder MJ, Cenit MC, Tigchelaar EF, Maatman A, Dekens JA, et al. The Gut Microbiome Contributes to a Substantial Proportion of the Variation in Blood Lipids. Circ Res (2015) 117(9):817–24. doi: 10.1161/CIRCRESAHA.115.306807
35. Jumpertz R, Le DS, Turnbaugh PJ, Trinidad C, Bogardus C, Gordon JI, Krakoff J, et al. Energy-Balance Studies Reveal Associations Between Gut Microbes, Caloric Load, and Nutrient Absorption in Humans. Am J Clin Nutr (2011) 94(1):58–65. doi: 10.3945/ajcn.110.010132
36. Indiani CMDSP, Rizzardi KF, Castelo PM, Ferraz LFC, Darrieux M, Parisotto TM. Childhood Obesity and Firmicutes/Bacteroidetes Ratio in the Gut Microbiota: A Systematic Review. Child Obes (2018) 14(8):501–9. doi: 10.1089/chi.2018.0040
37. Ridaura VK, Faith JJ, Rey FE, Cheng J, Duncan AE, Kau AL, et al. Gut Microbiota From Twins Discordant for Obesity Modulate Metabolism in Mice. Science (2013) 341(6150):1241214. doi: 10.1126/science.1241214
38. Mestdagh R, Dumas ME, Rezzi S, Kochhar S, Holmes E, Claus SP, et al. Gut Microbiota Modulate the Metabolism of Brown Adipose Tissue in Mice. J Proteome Res (2012) 11(2):620–30. doi: 10.1021/pr200938v
39. Chuang HL, Huang YT, Chiu CC, Liao CD, Hsu FL, Huang CC, et al. Metabolomics Characterization of Energy Metabolism Reveals Glycogen Accumulation in Gut-Microbiota-Lacking Mice. J Nutr Biochem (2012) 23(7):752–8. doi: 10.1016/j.jnutbio.2011.03.019
40. Le Roy T, Lécuyer E, Chassaing B, Rhimi M, Lhomme M, Boudebbouze S, et al. The Intestinal Microbiota Regulates Host Cholesterol Homeostasis. BMC Biol (2019) 17(1):94. doi: 10.1186/s12915-019-0715-8
41. Liu BN, Liu XT, Liang ZH, Wang JH. Gut Microbiota in Obesity. World J Gastroenterol (2021) 27(25):3837–50. doi: 10.3748/wjg.v27.i25.3837
42. Tazi A, Araujo JR, Mulet C, Arena ET, Nigro G, Pédron T, et al. Disentangling Host-Microbiota Regulation of Lipid Secretion by Enterocytes: Insights From Commensals Lactobacillus Paracasei and Escherichia Coli. mBio (2018) 9(5):e01493–18. doi: 10.1128/mBio.01493-18
43. Araújo JR, Tazi A, Burlen-Defranoux O, Vichier-Guerre S, Nigro G, Licandro H, et al. Fermentation Products of Commensal Bacteria Alter Enterocyte Lipid Metabolism. Cell Host Microbe (2020) 27(3):358–75.e7. doi: 10.1016/j.chom.2020.01.028
44. Aronsson L, Huang Y, Parini P, Korach-André M, Håkansson J, Gustafsson JÅ, et al. Decreased Fat Storage by Lactobacillus Paracasei is Associated With Increased Levels of Angiopoietin-Like 4 Protein (ANGPTL4). PLoS One (2010) 5(9):e13087. doi: 10.1371/journal.pone.0013087
45. Ramos-Romero S, Santocildes G, Pinol-Pinol D, Roses C, Pages T, Hereu M, et al. Implication of Gut Microbiota in the Physiology of Rats Intermittently Exposed to Cold and Hypobaric Hypoxia. PLoS One (2020) 15(11):e0240686. doi: 10.1371/journal.pone.0240686
46. Suárez-Zamorano N, Fabbiano S, Chevalier C, Stojanović O, Colin DJ, Stevanović A, et al. Microbiota Depletion Promotes Browning of White Adipose Tissue and Reduces Obesity. Nat Med (2015) 21(12):1497–501. doi: 10.1038/nm.3994
47. Nguyen KD, Qiu Y, Cui X, Goh YP, Mwangi J, David T, et al. Alternatively Activated Macrophages Produce Catecholamines to Sustain Adaptive Thermogenesis. Nature (2011) 480(7375):104–8. doi: 10.1038/nature10653
48. Sommer F, Bäckhed F. The Gut Microbiota–Masters of Host Development and Physiology. Nat Rev Microbiol (2013) 11(4):227–38. doi: 10.1038/nrmicro2974
49. Zietak M, Kovatcheva-Datchary P, Markiewicz LH, Ståhlman M, Kozak LP, Bäckhed F. Altered Microbiota Contributes to Reduced Diet-Induced Obesity Upon Cold Exposure. Cell Metab (2016) 23(6):1216–23. doi: 10.1016/j.cmet.2016.05.001
50. Worthmann A, John C, Rühlemann MC, Baguhl M, Heinsen FA, Schaltenberg N, et al. Cold-Induced Conversion of Cholesterol to Bile Acids in Mice Shapes the Gut Microbiome and Promotes Adaptive Thermogenesis. Nat Med (2017) 23(7):839–49. doi: 10.1038/nm.4357
51. Broeders EP, Nascimento EB, Havekes B, Brans B, Roumans KH, Tailleux A, et al. The Bile Acid Chenodeoxycholic Acid Increases Human Brown Adipose Tissue Activity. Cell Metab (2015) 22(3):418–26. doi: 10.1016/j.cmet.2015.07.002
52. Zhang E, Jin L, Wang Y, Tu J, Zheng R, Ding L, et al. Intestinal AMPK Modulation of Microbiota Mediates Crosstalk With Brown Fat to Control Thermogenesis. Nat Commun (2022) 13(1):1135. doi: 10.1038/s41467-022-28743-5
53. Li B, Li L, Li M, Lam SM, Wang G, Wu Y, et al. Microbiota Depletion Impairs Thermogenesis of Brown Adipose Tissue and Browning of White Adipose Tissue. Cell Rep (2019) 26(10):2720–37.e5. doi: 10.1016/j.celrep.2019.02.015
54. Fischer K, Ruiz HH, Jhun K, Finan B, Oberlin DJ, van der Heide V, et al. Alternatively Activated Macrophages do Not Synthesize Catecholamines or Contribute to Adipose Tissue Adaptive Thermogenesis. Nat Med (2017) 23(5):623–30. doi: 10.1038/nm.4316
55. Krisko TI, Nicholls HT, Bare CJ, Holman CD, Putzel GG, Jansen RS, et al. Dissociation of Adaptive Thermogenesis From Glucose Homeostasis in Microbiome-Deficient Mice. Cell Metab (2020) 31(3):592–604.e9. doi: 10.1016/j.cmet.2020.01.012
56. de Cabo R, Mattson MP. Effects of Intermittent Fasting on Health, Aging, and Disease. N Engl J Med (2019) 381(26):2541–51. doi: 10.1056/NEJMra1905136
57. Li G, Xie C, Lu S, Nichols RG, Tian Y, Li L, et al. Intermittent Fasting Promotes White Adipose Browning and Decreases Obesity by Shaping the Gut Microbiota. Cell Metab (2017) 26(4):672–85.e4. doi: 10.1016/j.cmet.2017.08.019
58. Kim N, Nam M, Kang MS, Lee JO, Lee YW, Hwang GS, et al. Piperine Regulates UCP1 Through the AMPK Pathway by Generating Intracellular Lactate Production in Muscle Cells. Sci Rep (2017) 7:41066. doi: 10.1038/srep410666
59. Sahuri-Arisoylu M, Brody LP, Parkinson JR, Parkes H, Navaratnam N, Miller AD, et al. Reprogramming of Hepatic Fat Accumulation and 'Browning' of Adipose Tissue by the Short-Chain Fatty Acid Acetate. Int J Obes (Lond) (2016) 40(6):955–63. doi: 10.1038/ijo.2016.23
60. Kim KH, Kim YH, Son JE, Lee JH, Kim S, Choe MS, et al. Intermittent Fasting Promotes Adipose Thermogenesis and Metabolic Homeostasis via VEGF-Mediated Alternative Activation of Macrophage. Cell Res (2017) 27(11):1309–26. doi: 10.1038/cr.2017.126
61. Fabbiano S, Suárez-Zamorano N, Rigo D, Veyrat-Durebex C, Stevanovic Dokic A, Colin DJ, et al. Caloric Restriction Leads to Browning of White Adipose Tissue Through Type 2 Immune Signaling. Cell Metab (2016) 24(3):434–46. doi: 10.1016/j.cmet.2016.07.023
62. Fabbiano S, Suárez-Zamorano N, Chevalier C, Lazarević V, Kieser S, Rigo D, et al. Functional Gut Microbiota Remodeling Contributes to the Caloric Restriction-Induced Metabolic Improvements. Cell Metab (2018) 28(6):907–21.e7. doi: 10.1016/j.cmet.2018.08.005
63. Dao MC, Everard A, Aron-Wisnewsky J, Sokolovska N, Prifti E, Verger EO, et al. Akkermansia Muciniphila and Improved Metabolic Health During a Dietary Intervention in Obesity: Relationship With Gut Microbiome Richness and Ecology. Gut (2016) 65(3):426–36. doi: 10.1136/gutjnl-2014-308778
64. Li M, Wang S, Li Y, Zhao M, Kuang J, Liang D, et al. Gut Microbiota-Bile Acid Crosstalk Contributes to the Rebound Weight Gain After Calorie Restriction in Mice. Nat Commun (2022) 13(1):2060. doi: 10.1038/s41467-022-29589-7
65. Moreno-Navarrete JM, Serino M, Blasco-Baque V, Azalbert V, Barton RH, Cardellini M, et al. Gut Microbiota Interacts With Markers of Adipose Tissue Browning, Insulin Action and Plasma Acetate in Morbid Obesity. Mol Nutr Food Res (2018) 62(3):1700721. doi: 10.1002/mnfr.201700721
66. Wilkinson MJ, Manoogian ENC, Zadourian A, Lo H, Fakhouri S, Shoghi A, et al. Ten-Hour Time-Restricted Eating Reduces Weight, Blood Pressure, and Atherogenic Lipids in Patients With Metabolic Syndrome. Cell Metab (2020) 31(1):92–104.e5. doi: 10.1016/j.cmet.2019.11.004
67. Liu D, Huang Y, Huang C, Yang S, Wei X, Zhang P, et al. Calorie Restriction With or Without Time-Restricted Eating in Weight Loss. N Engl J Med (2022) 386(16):1495–504. doi: 10.1056/NEJMoa2114833
68. Saltiel AR, Olefsky JM. Inflammatory Mechanisms Linking Obesity and Metabolic Disease. J Clin Invest (2017) 127(1):1–4. doi: 10.1172/JCI9203
69. Brunner KT, Henneberg CJ, Wilechansky RM, Long MT. Nonalcoholic Fatty Liver Disease and Obesity Treatment. Curr Obes Rep (2019) 8(3):220–8. doi: 10.1007/s13679-019-00345-1
70. Netea MG, Balkwill F, Chonchol M, Cominelli F, Donath MY, Giamarellos-Bourboulis EJ, et al. A Guiding Map for Inflammation. Nat Immunol (2017) 18(8):826–31. doi: 10.1038/ni.3790
71. Kaddai V, Jager J, Gonzalez T, Najem-Lendom R, Bonnafous S, Tran A, et al. Involvement of TNF-Alpha in Abnormal Adipocyte and Muscle Sortilin Expression in Obese Mice and Humans. Diabetologia (2009) 52(5):932–40. doi: 10.1007/s00125-009-1273-3
72. Chawla A, Nguyen KD, Goh YP. Macrophage-Mediated Inflammation in Metabolic Disease. Nat Rev Immunol (2011) 11(11):738–49. doi: 10.1038/nri30719
73. Cotillard A, Kennedy SP, Kong LC, Prifti E, Pons N, Le Chatelier E, et al. Dietary Intervention Impact on Gut Microbial Gene Richness. Nature (2013) 500(7464):585–8. doi: 10.1038/nature12480
74. Novotny Núñez I, Maldonado Galdeano C, de Moreno de LeBlanc A, Perdigón G. Lactobacillus Casei CRL 431 Administration Decreases Inflammatory Cytokines in a Diet-Induced Obese Mouse Model. Nutrition (2015) 31(7-8):1000–7. doi: 10.1016/j.nut.2015.02.006
75. Luck H, Tsai S, Chung J, Clemente-Casares X, Ghazarian M, Revelo XS, et al. Regulation of Obesity-Related Insulin Resistance With Gut Anti-Inflammatory Agents. Cell Metab (2015) 21(4):527–42. doi: 10.1016/j.cmet.2015.03.001
76. Cani PD, Amar J, Iglesias MA, Poggi M, Knauf C, Bastelica D, et al. Metabolic Endotoxemia Initiates Obesity and Insulin Resistance. Diabetes (2007) 56(7):1761–72. doi: 10.2337/db06-1491
77. Hersoug LG, Møller P, Loft S. Role of Microbiota-Derived Lipopolysaccharide in Adipose Tissue Inflammation, Adipocyte Size and Pyroptosis During Obesity. Nutr Res Rev (2018) 31(2):153–63. doi: 10.1017/S0954422417000269
78. Tanaka M. Molecular Mechanism of Obesity-Induced Adipose Tissue Inflammation; the Role of Mincle in Adipose Tissue Fibrosis and Ectopic Lipid Accumulation. Endocr J (2020) 67(2):107–11. doi: 10.1507/endocrj.EJ19-0417
79. Al Bander Z, Nitert MD, Mousa A, Naderpoor N. The Gut Microbiota and Inflammation: An Overview. Int J Environ Res Public Health (2020) 17(20):7618. doi: 10.3390/ijerph17207618
80. Ghanim H, Abuaysheh S, Sia CL, Korzeniewski K, Chaudhuri A, Fernandez-Real JM, et al. Increase in Plasma Endotoxin Concentrations and the Expression of Toll-Like Receptors and Suppressor of Cytokine Signaling-3 in Mononuclear Cells After a High-Fat, High-Carbohydrate Meal: Implications for Insulin Resistance. Diabetes Care (2009) 32(12):2281–7. doi: 10.2337/dc09-0979
81. Park BS, Song DH, Kim HM, Choi BS, Lee H, Lee JO. The Structural Basis of Lipopolysaccharide Recognition by the TLR4-MD-2 Complex. Nature (2009) 458(7242):1191–5. doi: 10.1038/nature07830
82. Orr JS, Puglisi MJ, Ellacott KL, Lumeng CN, Wasserman DH, Hasty AH. Toll-Like Receptor 4 Deficiency Promotes the Alternative Activation of Adipose Tissue Macrophages. Diabetes (2012) 61(11):2718–27. doi: 10.2337/db11-1595
83. Cai L, Wang Z, Ji A, Meyer JM, van der Westhuyzen DR. Scavenger Receptor CD36 Expression Contributes to Adipose Tissue Inflammation and Cell Death in Diet-Induced Obesity. PLoS One (2012) 7(5):e36785. doi: 10.1371/journal.pone.0036785
84. Rooks MG, Garrett WS. Gut Microbiota, Metabolites and Host Immunity. Nat Rev Immunol (2016) 16(6):341–52. doi: 10.1038/nri.2016.42
85. den Besten G, van Eunen K, Groen AK, Venema K, Reijngoud DJ, Bakker BM. The Role of Short-Chain Fatty Acids in the Interplay Between Diet, Gut Microbiota, and Host Energy Metabolism. J Lipid Res (2013) 54(9):2325–40. doi: 10.1194/jlr.R036012
86. Tilg H, Zmora N, Adolph TE, Elinav E. The Intestinal Microbiota Fuelling Metabolic Inflammation. Nat Rev Immunol (2020) 20(1):40–54. doi: 10.1038/s41577-019-0198-4
87. Kemgang TS, Kapila S, Shanmugam VP, Kapila R. Cross-Talk Between Probiotic Lactobacilli and Host Immune System. J Appl Microbiol (2014) 117(2):303–19. doi: 10.1111/jam.12521
88. Fabersani E, Abeijon-Mukdsi MC, Ross R, Medina R, González S, Gauffin-Cano P. Specific Strains of Lactic Acid Bacteria Differentially Modulate the Profile of Adipokines In Vitro. Front Immunol (2017) 8. doi: 10.3389/fimmu.2017.00266
89. Kim SW, Park KY, Kim B, Kim E, Hyun CK. Lactobacillus Rhamnosus GG Improves Insulin Sensitivity and Reduces Adiposity in High-Fat Diet-Fed Mice Through Enhancement of Adiponectin Production. Biochem Biophys Res Commun (2013) 431(2):258–63. doi: 10.1016/j.bbrc.2012.12.121
90. Cheng YC, Liu JR. Effect of Lactobacillus Rhamnosus GG on Energy Metabolism, Leptin Resistance, and Gut Microbiota in Mice With Diet-Induced Obesity. Nutrients (2020) 12(9):2557. doi: 10.3390/nu12092557
91. Okubo T, Takemura N, Yoshida A, Sonoyama K. KK/Ta Mice Administered Lactobacillus Plantarum Strain No. 14 Have Lower Adiposity and Higher Insulin Sensitivity. Biosci Microbiota Food Health (2013) 32(3):93–100. doi: 10.12938/bmfh.32.93
92. Shin NR, Lee JC, Lee HY, Kim MS, Whon TW, Lee MS, et al. An Increase in the Akkermansia Spp. Population Induced by Metformin Treatment Improves Glucose Homeostasis in Diet-Induced Obese Mice. Gut (2014) 63(5):727–35. doi: 10.1136/gutjnl-2012-303839
93. Xu J, Liang R, Zhang W, Tian K, Li J, Chen X, et al. Faecalibacterium Prausnitzii-Derived Microbial Anti-Inflammatory Molecule Regulates Intestinal Integrity in Diabetes Mellitus Mice via Modulating Tight Junction Protein Expression. J Diabetes (2020) 12(3):224–36. doi: 10.1111/1753-0407.12986
94. Sokol H, Pigneur B, Watterlot L, Lakhdari O, Bermúdez-Humarán LG, Gratadoux JJ, et al. Faecalibacterium Prausnitzii is an Anti-Inflammatory Commensal Bacterium Identified by Gut Microbiota Analysis of Crohn Disease Patients. Proc Natl Acad Sci USA (2008) 105(43):16731–6. doi: 10.1073/pnas.0804812105
95. Abenavoli L, Scarpellini E, Colica C, Boccuto L, Salehi B, Sharifi-Rad J, et al. Gut Microbiota and Obesity: A Role for Probiotics. Nutrients (2019) 11(11):2690. doi: 10.3390/nu11112690
96. Ezeji JC, Sarikonda DK, Hopperton A, Erkkila HL, Cohen DE, Martinez SP, et al. Parabacteroides Distasonis: Intriguing Aerotolerant Gut Anaerobe With Emerging Antimicrobial Resistance and Pathogenic and Probiotic Roles in Human Health. Gut Microbes (2021) 13(1):1922241. doi: 10.1080/19490976.2021.1922241
97. Xu Y, Wang N, Tan HY, Li S, Zhang C, Zhang Z, et al. Panax Notoginseng Saponins Modulate the Gut Microbiota to Promote Thermogenesis and Beige Adipocyte Reconstruction via Leptin-Mediated Ampkα/STAT3 Signaling in Diet-Induced Obesity. Theranostics (2020) 10(24):11302–23. doi: 10.7150/thno.47746
98. Caesar R, Tremaroli V, Kovatcheva-Datchary P, Cani PD, Bäckhed F. Crosstalk Between Gut Microbiota and Dietary Lipids Aggravates WAT Inflammation Through TLR Signaling. Cell Metab (2015) 22(4):658–68. doi: 10.1016/j.cmet.2015.07.026
99. Harris K, Kassis A, Major G, Chou CJ. Is the Gut Microbiota a New Factor Contributing to Obesity and its Metabolic Disorders? J Obes (2012) 2012:879151. doi: 10.1155/2012/879151
100. Ussar S, Griffin NW, Bezy O, Fujisaka S, Vienberg S, Softic S, et al. Interactions Between Gut Microbiota, Host Genetics and Diet Modulate the Predisposition to Obesity and Metabolic Syndrome. Cell Metab (2015) 22(3):516–30. doi: 10.1016/j.cmet.2015.07.007
101. FFasshauer M, Blüher M. Adipokines in Health and Disease. Trends Pharmacol Sci (2015) 36(7):461–70. doi: 10.1016/j.tips.2015.04.014
102. Chan JL, Heist K, DePaoli AM, Veldhuis JD, Mantzoros CS. The Role of Falling Leptin Levels in the Neuroendocrine and Metabolic Adaptation to Short-Term Starvation in Healthy Men. J Clin Invest (2003) 111(9):1409–21. doi: 10.1172/JCI17490
103. Yao H, Fan C, Fan X, Lu Y, Wang Y, Wang R, et al. Effects of Gut Microbiota on Leptin Expression and Body Weight are Lessened by High-Fat Diet in Mice. Br J Nutr (2020) 124(4):396–406. doi: 10.1017/S0007114520001117
104. Aron-Wisnewsky J, Warmbrunn MV, Nieuwdorp M, Clément K. Metabolism and Metabolic Disorders and the Microbiome: The Intestinal Microbiota Associated With Obesity, Lipid Metabolism, and Metabolic Health-Pathophysiology and Therapeutic Strategies. Gastroenterology (2021) 160(2):573–99. doi: 10.1053/j.gastro.2020.10.057
105. Schéle E, Grahnemo L, Anesten F, Hallén A, Bäckhed F, Jansson JO. The Gut Microbiota Reduces Leptin Sensitivity and the Expression of the Obesity-Suppressing Neuropeptides Proglucagon (Gcg) and Brain-Derived Neurotrophic Factor (Bdnf) in the Central Nervous System. Endocrinology (2013) 154(10):3643–51. doi: 10.1210/en.2012-2151
106. Everard A, Lazarevic V, Derrien M, Girard M, Muccioli GG, Neyrinck AM, et al. Responses of Gut Microbiota and Glucose and Lipid Metabolism to Prebiotics in Genetic Obese and Diet-Induced Leptin-Resistant Mice. Diabetes (2011) 60(11):2775–86. doi: 10.2337/db11-0227
107. Narmaki E, Borazjani M, Ataie-Jafari A, Hariri N, Doost AH, Qorbani M, et al. The Combined Effects of Probiotics and Restricted Calorie Diet on the Anthropometric Indices, Eating Behavior, and Hormone Levels of Obese Women With Food Addiction: A Randomized Clinical Trial. Nutr Neurosci (2022) 25(5):963–75. doi: 10.1080/1028415X.2020.1826763
108. Grases-Pintó B, Abril-Gil M, Castell M, Rodríguez-Lagunas MJ, Burleigh S, Fåk Hållenius F, et al. Influence of Leptin and Adiponectin Supplementation on Intraepithelial Lymphocyte and Microbiota Composition in Suckling Rats. Front Immunol (2019) 10. doi: 10.3389/fimmu.2019.02369
109. Li X, Yang L, Li J, Lin L, Zheng G. A Flavonoid-Rich Smilax China L. Extract Prevents Obesity by Upregulating the Adiponectin-Receptor/AMPK Signalling Pathway and Modulating the Gut Microbiota in Mice. Food Funct (2021) 12(13):5862–75. doi: 10.1039/d1fo00282a
110. Yao H, Fan C, Lu Y, Fan X, Xia L, Li P, et al. Alteration of Gut Microbiota Affects Expression of Adiponectin and Resistin Through Modifying DNA Methylation in High-Fat Diet-Induced Obese Mice. Genes Nutr (2020) 15(1):12. doi: 10.1186/s12263-020-00671-3
111. Membrez M, Blancher F, Jaquet M, Jaquet M, Bibiloni R, Cani PD, et al. Gut Microbiota Modulation With Norfloxacin and Ampicillin Enhances Glucose Tolerance in Mice. FASEB J (2008) 22(7):2416–624. doi: 10.1096/fj.07-102723
112. Itoh N. FGF21 as a Hepatokine, Adipokine, and Myokine in Metabolism and Diseases. Front Endocrinol (Lausanne) (2014) 5. doi: 10.3389/fendo.2014.00107
113. Martin A, Ecklu-Mensah G, Ha CWY, Hendrick G, Layman DK, Gilbert J, et al. Gut Microbiota Mediate the FGF21 Adaptive Stress Response to Chronic Dietary Protein-Restriction in Mice. Nat Commun (2021) 12(1):3838. doi: 10.1038/s41467-021-24074-z
114. Markan KR, Naber MC, Ameka MK, Anderegg MD, Mangelsdorf DJ, Kliewer SA, et al. Circulating FGF21 is Liver Derived and Enhances Glucose Uptake During Refeeding and Overfeeding. Diabetes (2014) 63:4057–63. doi: 10.2337/db14-0595
115. Han MS, Perry RJ, Camporez JP, Scherer PE, Shulman GI, Gao G, et al. A Feed-Forward Regulatory Loop in Adipose Tissue Promotes Signaling by the Hepatokine FGF21. Genes Dev (2021) 35(1-2):133–46. doi: 10.1101/gad.344556.120
116. Laeger T, Albarado DC, Burke SJ, Trosclair L, Hedgepeth JW, Berthoud HR, et al. Metabolic Responses to Dietary Protein Restriction Require an Increase in FGF21 That Is Delayed by the Absence of GCN2. Cell Rep (2016) 16(3):707–16. doi: 10.1016/j.celrep.2016.06.044
117. Golonka RM, Xiao X, Abokor AA, Joe B, Vijay-Kumar M. Altered Nutrient Status Reprograms Host Inflammation and Metabolic Health via Gut Microbiota. J Nutr Biochem (2020) 80:108360. doi: 10.1016/j.jnutbio.2020
118. Asrih M, Altirriba J, Rohner-Jeanrenaud F, Jornayvaz FR. Ketogenic Diet Impairs FGF21 Signaling and Promotes Differential Inflammatory Responses in the Liver and White Adipose Tissue. PLoS One (2015) 10(5):e0126364. doi: 10.1371/journal.pone.0126364
119. Maenhaut N, Van de Voorde J. Regulation of Vascular Tone by Adipocytes. BMC Med (2011) 9:25. doi: 10.1186/1741-7015-9-25
120. Dray C, Knauf C, Daviaud D, Waget A, Boucher J, Buléon M, et al. Apelin Stimulates Glucose Utilization in Normal and Obese Insulin-Resistant Mice. Cell Metab (2008) 8(5):437–45. doi: 10.1016/j.cmet.2008.10.003
121. Geurts L, Lazarevic V, Derrien M, Everard A, Van Roye M, Knauf C, et al. Altered Gut Microbiota and Endocannabinoid System Tone in Obese and Diabetic Leptin-Resistant Mice: Impact on Apelin Regulation in Adipose Tissue. Front Microbiol (2011) 2. doi: 10.3389/fmicb.2011.00149
122. Bäckhed F, Normark S, Schweda EK, Oscarson S, Richter-Dahlfors . Structural Requirements for TLR4-Mediated LPS Signalling: A Biological Role for LPS Modifications. Microbes Infect (2003) 5(12):1057–563. doi: 10.1016/s1286-4579(03)00207-7
123. Cani PD, Bibiloni R, Knauf C, Waget A, Neyrinck AM, Delzenne NM, et al. Changes in Gut Microbiota Control Metabolic Endotoxemia-Induced Inflammation in High-Fat Diet-Induced Obesity and Diabetes in Mice. Diabetes (2008) 57(6):1470–81. doi: 10.2337/db07-1403
124. Cani PD, Osto M, Geurts L, Everard A. Involvement of Gut Microbiota in the Development of Low-Grade Inflammation and Type 2 Diabetes Associated With Obesity. Gut Microbes (2012) 3(4):279–88. doi: 10.4161/gmic.19625
125. Trøseid M, Nestvold TK, Rudi K, Thoresen H, Nielsen EW, Lappegård KT. Plasma Lipopolysaccharide is Closely Associated With Glycemic Control and Abdominal Obesity: Evidence From Bariatric Surgery. Diabetes Care (2013) 36(11):3627–32. doi: 10.2337/dc13-0451
126. Hersoug LG, Møller P, Loft S. Gut Microbiota-Derived Lipopolysaccharide Uptake and Trafficking to Adipose Tissue: Implications for Inflammation and Obesity. Obes Rev (2016) 17(4):297–312. doi: 10.1111/obr.12370
127. Blaak EE, Canfora EE, Theis S, Frost G, Groen AK, Mithieux G, et al. Short Chain Fatty Acids in Human Gut and Metabolic Health. Benef Microbes (2020) 11(5):411–55. doi: 10.3920/BM2020.0057
128. Murphy EF, Cotter PD, Healy S, Marques TM, O'Sullivan O, Fouhy F, et al. Composition and Energy Harvesting Capacity of the Gut Microbiota: Relationship to Diet, Obesity and Time in Mouse Models. Gut (2010) 59(12):1635–42. doi: 10.1136/gut.2010.215665
129. Perry RJ, Peng L, Barry NA, Cline GW, Zhang D, Cardone RL, et al. Acetate Mediates a Microbiome-Brain-β-Cell Axis to Promote Metabolic Syndrome. Nature (2016) 534(7606):213–7. doi: 10.1038/nature18309
130. Schwiertz A, Taras D, Schäfer K, Beijer S, Bos NA, Donus C, et al. Microbiota and SCFA in Lean and Overweight Healthy Subjects. Obes (Silver Spring) (2010) 18(1):190–5. doi: 10.1038/oby.2009.167
131. Canfora EE, Jocken JW, Blaak EE. Short-Chain Fatty Acids in Control of Body Weight and Insulin Sensitivity. Nat Rev Endocrinol (2015) 11(10):577–91. doi: 10.1038/nrendo.2015.128
132. Lu Y, Fan C, Li P, Lu Y, Chang X, Qi K. Short Chain Fatty Acids Prevent High-Fat-Diet-Induced Obesity in Mice by Regulating G Protein-Coupled Receptors and Gut Microbiota. Sci Rep (2016) 6:37589. doi: 10.1038/srep37589
133. Gao Z, Yin J, Zhang J, Ward RE, Martin RJ, Lefevre M, et al. Butyrate Improves Insulin Sensitivity and Increases Energy Expenditure in Mice. Diabetes (2009) 58(7):1509–17. doi: 10.2337/db08-1637
134. den Besten G, Bleeker A, Gerding A, van Eunen K, Havinga R, van Dijk TH, et al. Short-Chain Fatty Acids Protect Against High-Fat Diet-Induced Obesity via a Pparγ-Dependent Switch From Lipogenesis to Fat Oxidation. Diabetes (2015) 64(7):2398–408. doi: 10.2337/db14-1213
135. Frost G, Sleeth ML, Sahuri-Arisoylu M, Lizarbe B, Cerdan S, Brody L, et al. The Short-Chain Fatty Acid Acetate Reduces Appetite via a Central Homeostatic Mechanism. Nat Commun (2014) 5:3611. doi: 10.1038/ncomms4611
136. Kimura I, Ozawa K, Inoue D, Imamura T, Kimura K, Maeda T, et al. The Gut Microbiota Suppresses Insulin-Mediated Fat Accumulation via the Short-Chain Fatty Acid Receptor GPR43. Nat Commun (2013) 4:1829. doi: 10.1038/ncomms2852
137. Yu H, Li R, Huang H, Yao R, Shen S. Short-Chain Fatty Acids Enhance the Lipid Accumulation of 3T3-L1 Cells by Modulating the Expression of Enzymes of Fatty Acid Metabolism. Lipids (2018) 53(1):77–84. doi: 10.1002/lipd.12005
138. Hong YH, Nishimura Y, Hishikawa D, Tsuzuki H, Miyahara H, Gotoh C, et al. Acetate and Propionate Short Chain Fatty Acids Stimulate Adipogenesis via GPCR43. Endocrinology (2005) 146(12):5092–9. doi: 10.1210/en.2005-0545
139. Li Z, Yi C-X, Katiraei S, Kooijman S, Zhou E, Chung CK, et al. Butyrate Reduces Appetite and Activates Brown Adipose Tissue via the Gut-Brain Neural Circuit. Gut (2018) 67(7):1269–79. doi: 10.1136/gutjnl-2017-314050
140. Wahlström A, Sayin SI, Marschall HU, Bäckhed F. Intestinal Crosstalk Between Bile Acids and Microbiota and Its Impact on Host Metabolism. Cell Metab (2016) 24(1):41–50. doi: 10.1016/j.cmet.2016.05.005
141. Castellanos-Jankiewicz A, Guzmán-Quevedo O, Fénelon VS, Zizzari P, Quarta C, Bellocchio L, et al. Hypothalamic Bile Acid-TGR5 Signaling Protects From Obesity. Cell Metab (2021) 33(7):1483–92.e10. doi: 10.1016/j.cmet.2021.04.009
142. Prawitt J, Abdelkarim M, Stroeve JH, Popescu I, Duez H, Velagapudi VR, et al. Farnesoid X Receptor Deficiency Improves Glucose Homeostasis in Mouse Models of Obesity. Diabetes (2011) 60(7):1861–71. doi: 10.2337/db11-0030
143. Fang S, Suh JM, Reilly SM, Yu E, Osborn O, Lackey D, et al. Intestinal FXR Agonism Promotes Adipose Tissue Browning and Reduces Obesity and Insulin Resistance. Nat Med (2015) 21(2):159–65. doi: 10.1038/nm.3760
144. Thomas C, Gioiello A, Noriega L, Strehle A, Oury J, Rizzo G, et al. TGR5-Mediated Bile Acid Sensing Controls Glucose Homeostasis. Cell Metab (2009) 10(3):167–77. doi: 10.1016/j.cmet.2009.08.001
145. Wang L, Gong Z, Zhang X, Zhu F, Liu Y, Jin C, et al. Gut Microbial Bile Acid Metabolite Skews Macrophage Polarization and Contributes to High-Fat Diet-Induced Colonic Inflammation. Gut Microbes (2020) 12(1):1–20. doi: 10.1080/19490976.2020.1819155
146. Iannotti FA, Di Marzo V. The Gut Microbiome, Endocannabinoids and Metabolic Disorders. J Endocrinol (2021) 248(2):R83–97. doi: 10.1530/JOE-20-0444
147. Schiano Moriello A, Di Marzo V, Petrosino S. Mutual Links Between the Endocannabinoidome and the Gut Microbiome, With Special Reference to Companion Animals: A Nutritional Viewpoint. Anim (Basel) (2022) 12(3):348. doi: 10.3390/ani12030348
148. Cani PD, Plovier H, Van Hul M, Geurts L, Delzenne NM, Druart C, et al. Endocannabinoids–at the Crossroads Between the Gut Microbiota and Host Metabolism. Nat Rev Endocrinol (2016) 12(3):133–43. doi: 10.1038/nrendo.2015.211
149. Schulz P, Hryhorowicz S, Rychter AM, Zawada A, Słomski R, Dobrowolska A, et al. What Role Does the Endocannabinoid System Play in the Pathogenesis of Obesity? Nutrients (2021) 13(2):373. doi: 10.3390/nu13020373
150. Lambert DM, Muccioli GG. Endocannabinoids and Related N-Acylethanolamines in the Control of Appetite and Energy Metabolism: Emergence of New Molecular Players. Curr Opin Clin Nutr Metab Care (2007) 10(6):735–44. doi: 10.1097/MCO.0b013e3282f00061
151. Muccioli GG, Naslain D, Bäckhed F, Reigstad CS, Lambert DM, Delzenne NM, et al. The Endocannabinoid System Links Gut Microbiota to Adipogenesis. Mol Syst Biol (2010) 6:392. doi: 10.1038/msb.2010.46
152. Bajzer M, Olivieri M, Haas MK, Pfluger PT, Magrisso IJ, Foster MT, et al. Cannabinoid Receptor 1 (CB1) Antagonism Enhances Glucose Utilization and Activates Brown Adipose Tissue in Diet-Induced Obese Mice. Diabetologia (2011) 54(12):3121–31. doi: 10.1007/s00125-011-2302-6
153. Di Paola M, Bonechi E, Provensi G, Costa A, Clarke G, Ballerini C, et al. Oleoylethanolamide Treatment Affects Gut Microbiota Composition and the Expression of Intestinal Cytokines in Peyer's Patches of Mice. Sci Rep (2018) 8(1):14881. doi: 10.1038/s41598-018-32925-x
154. Impellizzeri D, Bruschetta G, Cordaro M, Crupi R, Siracusa R, Esposito E, et al. Erratum to: Micronized/ultramicronized Palmitoylethanolamide Displays Superior Oral Efficacy Compared to Nonmicronized Palmitoylethanolamide in a Rat Model of Inflammatory Pain. J Neuroinflammation (2016) 13(1):129. doi: 10.1186/s12974-016-0595-6
155. Wu C, Zhao Y, Zhang Y, Yang Y, Su W, Yang Y, et al. Gut Microbiota Specifically Mediates the Anti-Hypercholesterolemic Effect of Berberine (BBR) and Facilitates to Predict BBR's Cholesterol-Decreasing Efficacy in Patients. J Adv Res (2021) 37:197–208. doi: 10.1016/j.jare.2021.07.011
Keywords: obesity, gut microbiota, adipose tissues, energy metabolism, inflammation
Citation: Wu D, Wang H, Xie L and Hu F (2022) Cross-Talk Between Gut Microbiota and Adipose Tissues in Obesity and Related Metabolic Diseases. Front. Endocrinol. 13:908868. doi: 10.3389/fendo.2022.908868
Received: 31 March 2022; Accepted: 06 June 2022;
Published: 05 July 2022.
Edited by:
Nabil Rabhi, Boston University, United StatesReviewed by:
Arashdeep Singh, University of Florida, United StatesLiwei Xie, Guangdong Academy of Science, China
Copyright © 2022 Wu, Wang, Xie and Hu. This is an open-access article distributed under the terms of the Creative Commons Attribution License (CC BY). The use, distribution or reproduction in other forums is permitted, provided the original author(s) and the copyright owner(s) are credited and that the original publication in this journal is cited, in accordance with accepted academic practice. No use, distribution or reproduction is permitted which does not comply with these terms.
*Correspondence: Fang Hu, hu_fang98@csu.edu.cn