Fire and Its Interactions With Other Drivers Shape a Distinctive, Semi-Arid ‘Mallee’ Ecosystem
- 1Department of Ecology, Environment and Evolution, Centre for Future Landscapes, La Trobe University, Bundoora, VIC, Australia
- 2School of Ecosystem and Forest Sciences, University of Melbourne, Parkville, VIC, Australia
- 3Centre for Integrative Ecology, School of Life and Environmental Sciences, Deakin University, Burwood, VIC, Australia
- 4689 Stedman Crescent, Albury, NSW, Australia
- 5Arthur Rylah Institute for Environmental Research, Department of Environment, Land, Water and Planning, Heidelberg, VIC, Australia
- 6Conservation in Human Landscapes Lab, Institute for Land, Water and Society, Charles Sturt University, Albury, NSW, Australia
- 7Department of Environment, Land, Water and Planning, Epsom, VIC, Australia
- 8Parks Victoria, Mildura, VIC, Australia
- 9School of Health and Life Sciences, Federation University, Ballarat, VIC, Australia
- 10Fire Management Branch, Department of Environment and Water, Adelaide, SA, Australia
Fire shapes ecosystems globally, including semi-arid ecosystems. In Australia, semi-arid ‘mallee’ ecosystems occur primarily across the southern part of the continent, forming an interface between the arid interior and temperate south. Mallee vegetation is characterized by short, multi-stemmed eucalypts that grow from a basal lignotuber. Fire shapes the structure and functioning of mallee ecosystems. Using the Murray Mallee region in south-eastern Australia as a case study, we examine the characteristics and role of fire, the consequences for biota, and the interaction of fire with other drivers. Wildfires in mallee ecosystems typically are large (1000s ha), burn with high severity, commonly cause top-kill of eucalypts, and create coarse-grained mosaics at a regional scale. Wildfires can occur in late spring and summer in both dry and wet years. Recovery of plant and animal communities is predictable and slow, with regeneration of eucalypts and many habitat components extending over decades. Time since the last fire strongly influences the distribution and abundance of many species and the structure of plant and animal communities. Animal species display a discrete set of generalized responses to time since fire. Systematic field studies and modeling are beginning to reveal how spatial variation in fire regimes (‘pyrodiversity’) at different scales shapes biodiversity. Pyrodiversity includes variation in the extent of post-fire habitats, the diversity of post-fire age-classes and their configuration. At regional scales, a desirable mix of fire histories for biodiversity conservation includes a combination of early, mid and late post-fire age-classes, weighted toward later seral stages that provide critical habitat for threatened species. Biodiversity is also influenced by interactions between fire and other drivers, including land clearing, rainfall, herbivory and predation. Extensive clearing for agriculture has altered the nature and impact of fire, and facilitated invasion by pest species that modify fuels, fire regimes and post-fire recovery. Given the natural and anthropogenic drivers of fire and the consequences of their interactions, we highlight opportunities for conserving mallee ecosystems. These include learning from and fostering Indigenous knowledge of fire, implementing actions that consider synergies between fire and other processes, and strategic monitoring of fire, biodiversity and other drivers to guide place-based, adaptive management under climate change.
Introduction
Fire shapes ecosystems worldwide (He et al., 2019; Kelly et al., 2020). Over half of Earth’s land surface is affected by fire, and some 30% experiences frequent fire (Chuvieco et al., 2008). There is increasing recognition of the role of fire as a major ecological and evolutionary force that has influenced global patterns of biodiversity, including the composition and structure of vegetation, species richness at local and landscape scales, levels of endemism and functional traits of plant and animal communities (He et al., 2019). Human activity and anthropogenic drivers (e.g., land use, biotic invasions, climate change) increasingly are transforming fire activity, with at least 4,400 species from a wide range of taxa and habitats facing threats associated with changing patterns of fire (Kelly et al., 2020). Importantly, fire does not occur in a uniform manner; rather, fire regimes – including the size, severity, frequency, season, extent and patchiness of fires – differ in distinctive ways between ecosystems (Chuvieco et al., 2008; Archibald et al., 2013). Consequently, for fire-prone ecosystems worldwide, understanding the role of fire in ecosystem function is critical for guiding future conservation and management.
Arid and semi-arid ecosystems make up a substantial component of the global area influenced by fire (Archibald et al., 2013). In Australia, arid ecosystems occupy ∼70% of the interior of the continent and encompass a range of vegetation types: Acacia woodlands, eucalypt woodlands, chenopod shrublands, hummock grasslands and tussock grasslands (Morton et al., 2011). To the north, driven by increasing and regular seasonal rainfall, arid ecosystems transition into tropical savannah woodlands and grasslands; to the south, they give way to semi-arid woodlands and shrublands. ‘Mallee’ vegetation - a major component of southern semi-arid woodlands - is a distinctive fire-prone ecosystem and the focus of this review. This ecosystem is dominated by evergreen sclerophyllous woodlands and shrublands that become highly flammable in dry summer months after the winter-spring growing season (Bradstock and Cohn, 2002), a pattern shared with other regions globally including the Mediterranean Basin, central and southern California, central Chile, and the Western Cape province, South Africa (Keeley et al., 2012).
‘Mallee’ is a colloquial term of Indigenous origin (Noble and Kimber, 1997; Yates et al., 2017), used to describe Eucalyptus species with a growth form of two or more stems of similar age arising from a subterranean lignotuber. Mallee eucalypts typically occur as a short (2–8 m) tree or tall shrub (Hill, 1989). The term also refers to a vegetation type, ‘mallee vegetation,’ dominated by an overstorey of mallee eucalypts. Mallee vegetation occurs at a continental scale across southern Australia, extending from south-western Western Australia to South Australia, Victoria and central New South Wales (Figure 1). At the time of European colonization, mallee vegetation probably covered around 302,000 km2. Since the mid-19th century, approximately one third of mallee vegetation has been cleared, principally for cereal cropping (Figure 1). Mallee ecosystems support a diverse flora and fauna, including numerous threatened species, such that effective conservation of this distinctive ecosystem has a key role in maintaining Australian biodiversity (Noble and Bradstock, 1989; Noble et al., 1990).
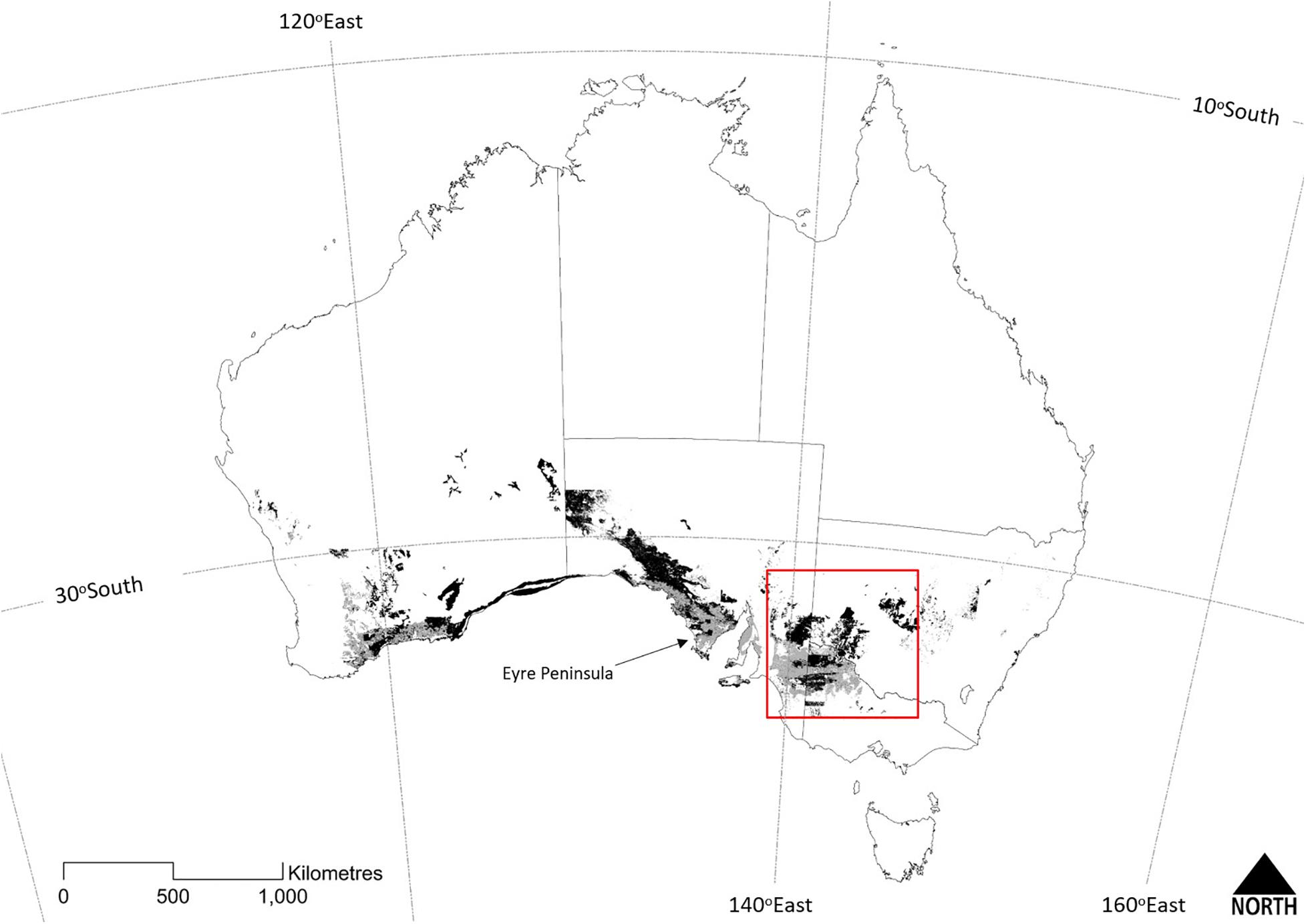
Figure 1. The temperate continental distribution of the Major Vegetation Group ‘mallee woodlands and shrublands’ across Australia (from Department of the Environment, 2018). Dark shading depicts extant vegetation and lighter shading depicts the likely former extent prior to clearing for agriculture. The red box frames the study area, the Murray-Darling Depression Bioregion (see Figure 2). Discontinuities in the extent of mallee vegetation across State borders reflects some inconsistencies between jurisdictional typologies.
Understanding the nature, characteristics and role of fire is crucial for conserving mallee biodiversity and responding to threats posed by anthropogenic change. Here, we review the context and role of fire in this semi-arid system, with particular reference to a case-study region in the Murray-Darling Depression Bioregion in south-eastern Australia (Figure 1), hereafter referred to as the ‘Murray Mallee region.’ We first set the context by outlining the landform and soils, climate, broad vegetation patterns, fauna, and human land use of this region. We then address four main questions. (1) What are the patterns and characteristic features of fire in semi-arid mallee ecosystems? (2) How do fire regimes shape biodiversity in this ecosystem? (3) How does fire interact with other drivers to influence biodiversity? (4) What are the potential consequences of a changing climate for fire regimes and their interactions with other drivers? We conclude with a summary of key issues for future conservation of mallee ecosystems and their biota and identify research questions to inform future management.
The Murray Mallee Region, South-Eastern Australia
Extensive tracts of mallee vegetation occur in several regions of southern Australia (Figure 1), notably: (a) the Murray Mallee region, encompassing adjoining areas in north-western Victoria, South Australia and western NSW; (b) the Eyre Peninsula and westward in southern South Australia; and (c) semi-arid parts of southern West Australia. While having a similar climate, mallee vegetation in western and south-eastern Australia have been geographically separated since the mid-Tertiary (Hill, 1989), and each has evolved a distinctive flora and fauna (Gosper et al., 2012a; Yates et al., 2017). Here, we focus on the Murray Mallee region (Figure 2), while also drawing on research from other mallee ecosystems in southern Australia.
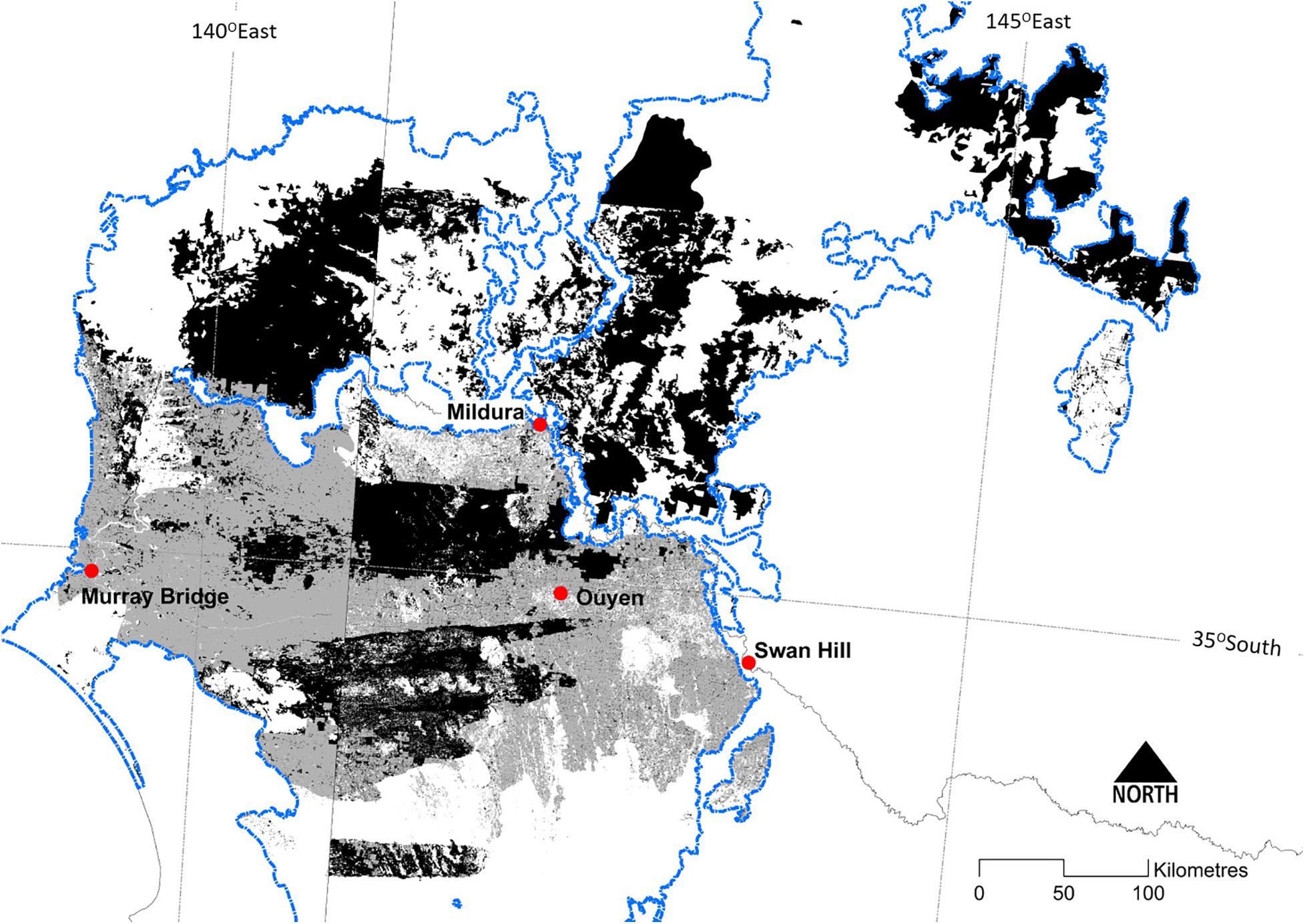
Figure 2. The distribution of the Major Vegetation Group ‘mallee woodlands and shrublands’ (Department of the Environment, 2018) within the Murray-Darling Depression Bioregion (Department of Sustainability Environment Water Population and Communities, 2013: the ‘Murray Mallee region’ in this review) (outlined in blue). Dark shading depicts extant vegetation and lighter shading depicts the likely former extent prior to clearing for agriculture. Discontinuities in the extent of mallee vegetation across State borders reflects some inconsistencies between jurisdictional typologies for vegetation.
Landform and Soils
The topography of the Murray Mallee region is predominantly flat, with modest local differences in elevation (∼20 m) primarily associated with dune systems and lunettes. At a regional scale, the landscape is broken up by saline flats and lakes (boinkas), which is more pronounced in mallee ecosystems in West Australia (O’Donnell et al., 2011a, b). Aside from the river and floodplain systems of the Murray and Darling Rivers, there are few large bodies of fresh surface water. Ephemeral water may pool in clay pans and depressions following heavy rains.
Mallee vegetation is largely restricted to aeolian landforms in the Murray Mallee region (typically dunes, interdunal swales and sandplains) within a broader mosaic interspersed with red earths, ephemeral lakes and lunettes that support very different vegetation communities, e.g., Allocasuarina woodlands and chenopod shrublands (Wasson, 1989). The region has two main aeolian formations, the Lowan Sands and the Woorinen Formation (Bowler and Magee, 1978; Pell et al., 2001). The Lowan Sands consists of west-south-west facing dunes; sediments are siliceous with very small quantities of clay and carbonate (Wasson, 1989). Dune systems of the Woorinen Formation comprise closely spaced east-west dunes, that are typically calcareous, and soils are heavier and finer textured than the Lowan Sands (Wasson, 1989).
Soil properties, especially texture and depth, affect the growth and distribution of overstorey Eucalyptus species (Parsons and Rowan, 1968; Sparrow, 1989; White, 2006; Pollock et al., 2018) and the composition of understorey species (Cohn et al., 2002; Haslem et al., 2010). This, in turn, influences the local rate of litter accumulation (Travers and Eldridge, 2013) and the flammability of vegetation (Bradstock and Cohn, 2002). Soil fertility is greater, and plant water availability is lower, where there is more clay in the soil (Parsons and Rowan, 1968). The combination of soil properties and flat topography in the Murray Mallee region create few moisture or edaphic barriers, allowing fire to spread rapidly whenever wind speed is sufficient to allow fire spread or ephemeral grasses comprise a well-connected fuel layer.
Climate
The region is semi-arid with cool winters and hot summers. The mean daily maximum temperature in summer months (January and February) exceeds 30°C and daily maxima >40°C are common (White, 2006). In winter, minimum temperatures can fall below 0°C overnight, with frequent frosts between May and September (White, 2006).
Mean annual rainfall ranges from ∼250 mm in the north to ∼500 mm in the south (White, 2006). In Australia, arid ecosystems experience rainfall that on a global scale is spatially and temporally unpredictable, such that large, infrequent rain events have a key role in shaping ecosystems (Morton et al., 2011). In contrast, semi-arid mallee ecosystems generally have more predictable and less-variable rainfall than the arid interior. Prolonged periods of below-average rainfall may still occur, as well as periods of high rainfall (White, 2006; Cullen and Grierson, 2009).
Vegetation
Mallee vegetation of the Murray Mallee region reflects variation in topography, soil texture, moisture availability and the long history of fire (Bradstock, 1989a; Cheal and Parkes, 1989; Hill, 1989; Parkes and Cheal, 1990; White, 2006; Cheal, 2010). Common plant families, apart from the Myrtaceae to which the eucalypts belong, include shrubs in the Fabaceae, Euphorbiaceae, Asteraceae, Proteaceae, Chenopodiaceae, Myoporaceae, and Cupressaceae (Bradstock and Cohn, 2002; Yates et al., 2017). While locally mediated by edaphic factors, the composition of the vegetation (e.g., life-form types) and fuel attributes (biomass, dryness and contiguity of fuels) correspond broadly with the north-south gradient in rainfall (Pausas and Bradstock, 2007; Gibson et al., 2014). There is a transition from greater cover of woody sclerophyllous shrubs in higher rainfall areas with sandy soils in the south, to a higher cover of the widespread and distinctive hummock grass Triodia scariosa in lower rainfall areas on fine textured clay-rich soils in the north (Gibson et al., 2014).
Here, we outline three broad vegetation types, Triodia Mallee, Chenopod Mallee and Heathy Mallee (Haslem et al., 2010; Yates et al., 2017) as a framework for describing mallee vegetation patterns and, subsequently, faunal patterns and fire responses.
Triodia Mallee represents plant communities on sandy flats and low dunes where the overstorey is dominated by eucalypts (including Eucalyptus dumosa and E. socialis) and the understorey typically is dominated by the perennial hummock grass Triodia scariosa (Figure 3A). Triodia Mallee is widespread across the region (Haslem et al., 2010) and fire-prone (Avitabile et al., 2013). Triodia hummocks are an important structural habitat component for a range of faunal species (Verdon et al., 2020), partly due to their amelioration of environmental temperature (Bell et al., 2021), but they are also a source of fuel for fire (Haslem et al., 2010).
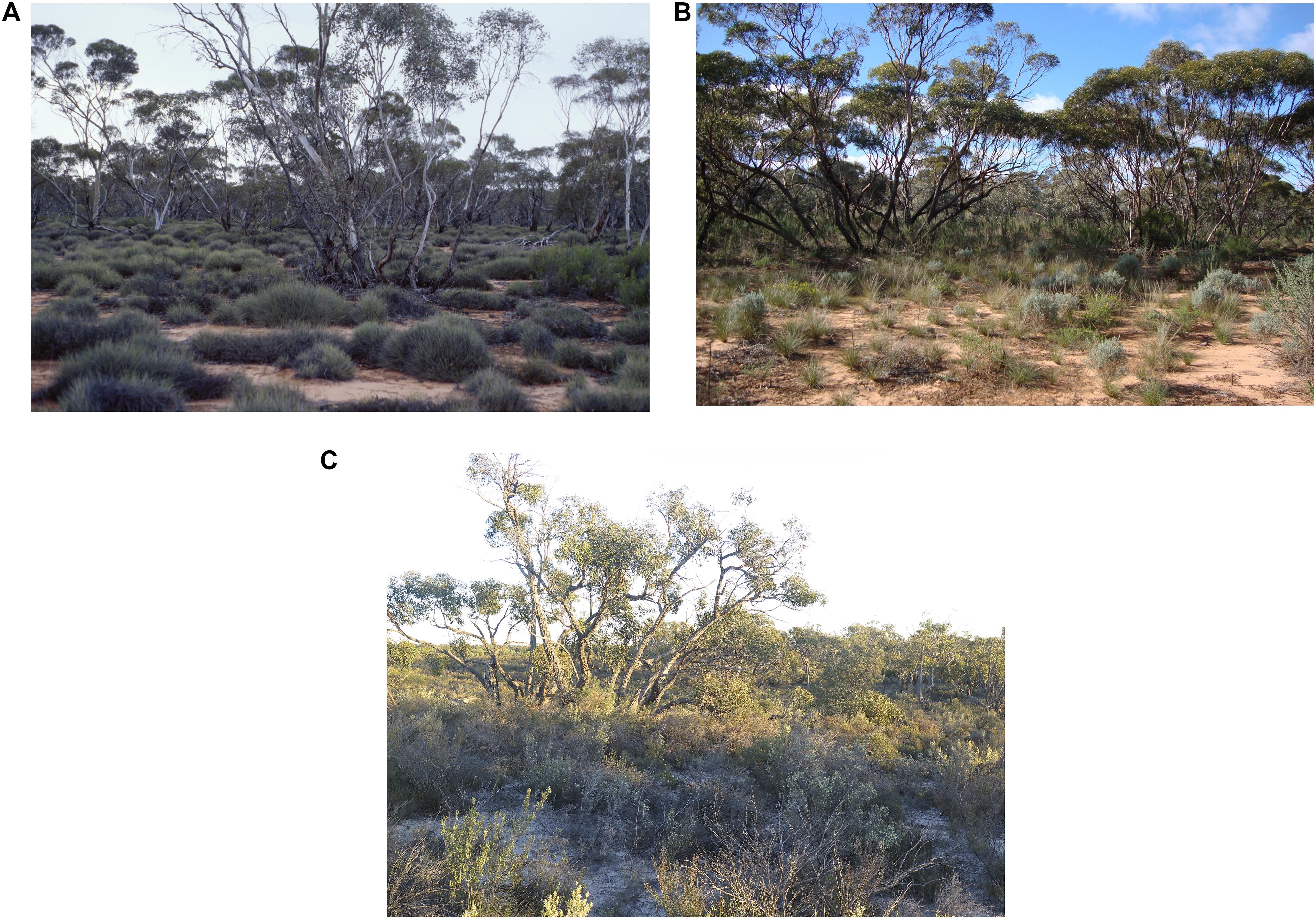
Figure 3. Examples of three broad types of mallee vegetation: (A) Triodia Mallee, (B) Chenopod Mallee, and (C) Heathy Mallee.
Chenopod Mallee vegetation commonly occurs on heavier-textured soils, notably the swales of the dune fields of the Woorinen Formation. Dominant eucalypt species include E. oleosa and E. gracilis, with an open understorey of low, perennial chenopod shrubs (e.g., Maireana, Sclerolaena, and Atriplex spp.) (Figure 3B). This vegetation type has a lower contiguity of ground-layer fuels and is less fire-prone; much of the long-unburnt mallee vegetation (e.g., >80 years since last fire) in the region is Chenopod Mallee.
Heathy Mallee vegetation occurs in the southern parts of the region where higher rainfall occurs, on deep aeolian sands (e.g., Big Desert, Little Desert, Ngarkat). It typically comprises sparse mallee eucalypts (e.g., E. costata, E. leptophylla, and E. arenacea) with a diverse understorey of sclerophyllous heathy shrubs (Figure 3C). On deep sands, it may give way to treeless heathlands lacking a mallee overstorey, or in places to a tree layer of desert stringybark (E. arenacea). The understorey is structurally and floristically diverse and includes numerous shrubs from the genera Banksia, Grevillea, Hakea, Leptospermum, and Melaleuca. The flowering patterns of these taxa provide a seasonal supply of nectar and seeds for fauna in many years.
Fauna
The fauna of mallee ecosystems reflects the biogeographic context, occupying a transition zone between arid and temperate biomes. The vertebrate fauna (particularly birds) includes representation of both Bassian (temperate) and Eyrean (arid) biogeographic elements, as well as species that occur throughout Australia (Menkhorst and Bennett, 1990).
Mallee ecosystems support distinct faunal communities (Schodde, 1981; Cogger, 1989; Menkhorst and Bennett, 1990), but few species are entirely dependent on mallee vegetation. Most species overlap in occurrence with an adjacent vegetation type (e.g., dryland and temperate woodlands, chenopod shrublands, heathlands). The only vertebrate taxa totally dependent on mallee vegetation are: four passerine birds, the white-bellied whipbird (Psophodes leucogaster leucogaster) (Burbidge et al., 2017), red-lored whistler (Pachycephala rufogularis), mallee emu-wren (Stipiturus mallee) and black-eared miner (Manorina melanotis); one reptile, the eared worm lizard (Aprasia aurita); and a small marsupial, the mallee ningaui (Ningaui yvonneae) (Menkhorst and Bennett, 1990).
The native mammal fauna of the Murray Mallee region at the time of European colonization (∼1840) was diverse; at least 43 species occurred in mallee vegetation (Bennett et al., 1989). Prominent components included members of the families Dasyuridae (marsupial carnivores), Peramelidae (bandicoots), Macropodidae (kangaroos and wallabies), Muridae (native rodents) and Vespertilionidae (insectivorous bats). Rapid and dramatic decline followed, with at least 13 species no longer present including five species now globally extinct (e.g., pig-footed bandicoot Chaeropus ecaudatus). Medium-sized species in the critical weight range 0.2–5 kg were most severely affected (Bennett et al., 1989), including ecosystem engineers (e.g., bandicoots, bettongs, and bilbies) that influence water infiltration and nutrient turnover through excavation of soils when foraging or burrowing (Eldridge and James, 2009). This regional loss of mammals was associated with massive changes to the vegetation (∼1860–1920) through grazing of domestic stock, introduction and over-abundance of the European rabbit (Oryctolagus cuniculus), and severe drought; accompanied by the introduction of exotic predators (red fox Vulpes vulpes, cat Felis catus) (Bennett et al., 1989, 2006).
Bird communities of mallee ecosystems in southern Australia comprise >150 species, dominated by insectivorous and nectarivorous passerines, with strong representation by parrots and raptors. They comprise taxa associated with arid Australia, melded with species from more-mesic zones (Schodde, 1990; Schodde and Mason, 1999). For example, in the widespread family Meliphagidae (honeyeaters), the white-eared honeyeater (Nesoptilotis leucotis), typical of mesic habitats, occurs alongside the yellow-plumed honeyeater (Ptilotula ornata) and grey-fronted honeyeater (Ptilotula plumula) associated with arid and semi-arid environments. The region also supports taxa that are widespread but exist as ‘isolated’ populations. For example, the striated grasswren (Amytornis striatus) occurs through much of arid Australia, but the isolated population in the region is a distinct subspecies (A. s. howei; Black et al., 2020). Similarly, the regent parrot (Polytelis anthopeplus monarchoides) is a locally isolated subspecies with a conspecific subspecies in south-western Western Australia.
Reptiles are a prominent component of the vertebrate fauna of mallee ecosystems (Cogger, 1989). A wide range of taxa occur including geckos (Families Gekkonidae, Carphodactylidae, and Diplodactylidae), legless lizards (Pygopodidae), skinks (Scincidae), dragons (Agamidae), goannas (Varanidae), blind snakes (Typhlopidae), python (Pythonidae) and venomous snakes (Elapidae). A relatively high richness of reptiles, both at individual sites and the regional scale, is characteristic of desert ecosystems in Australia (Pianka, 1969). Compositionally, the reptile fauna of mallee vegetation is more similar to that of the arid Eyrean biogeographic region than the mesic Bassian. Geckos, dragons and skinks dominate mallee assemblages, and genera such as Ctenotus, Lerista, and Diplodactylus that have speciated widely in arid environments are prominent in mallee vegetation (Menkhorst and Bennett, 1990).
While the number of frog species in mallee ecosystems is low, several species have adaptations that enable them to persist in this dry environment. In the Murray Mallee region, three species from the family Myobatrachidae occur in mallee vegetation (Menkhorst and Bennett, 1990). Of these, species from the genus Neobatrachus are most distinctively associated with mallee vegetation. They avoid desiccation by burrowing into the sandy soil, emerging to feed and breed in suitable conditions, typically following heavy rains.
The invertebrate fauna of mallee ecosystems is diverse, particularly ants (Andersen, 1982, 1983), beetles (Driscoll and Weir, 2005) and predatory arthropods – spiders, scorpions. Termites are widespread and ubiquitous and play a keystone role as herbivores and detritivores (Avitabile et al., 2015). The biogeography of invertebrates in mallee ecosystems is poorly documented, and the relatively few ecological studies of invertebrates in mallee communities relate mainly to responses to fire or land clearing (Andersen and Yen, 1985; Gullan et al., 1997; Schlesinger et al., 1997; Driscoll and Weir, 2005; Driscoll et al., 2020).
Human Land Use
Humans have lived in the Murray Mallee region for tens of thousands of years. The earliest archeological evidence comes from Lake Mungo and dates from 50 to 45,000 years before present (Ross, 1981; Bowler et al., 2003; Richards et al., 2007). The Murray Mallee region is home to Indigenous peoples who have maintained continuous connections to the land. The traditional homeland of some Indigenous peoples centered on river systems and lakes (e.g., Murray and Darling Rivers), with use of mallee vegetation along well-established paths (Clarke, 2009; Burch, 2020). The traditional homeland of other groups, such as the Ngarkat, included the extensive tracts of mallee vegetation (Clarke, 2009) where water was accessed from the roots of mallee trees and by building wells (Noble and Kimber, 1997; Barengi Gadjin Land Council, 2017). A wide range of plants and animals were used for food, medicine, ceremonies and trade. Kangaroos and smaller animals, such as stick-nest rats (Leporillus spp.), were hunted for food, and malleefowl (Leipoa ocellata) eggs were collected in some areas (Clarke, 2009).
Prior to European colonization, Indigenous peoples across Australia used cultural burning for a wide range of purposes including maintaining travel routes, promoting habitat for animals, harvesting of resources, ceremonies and supporting human health and well-being (e.g., Prober et al., 2016; Bliege Bird and Nimmo, 2018). Indigenous use of fire is a socially and ecologically complex practice, and knowledge is passed generationally within the context of a living culture (Victorian Traditional Owner Cultural Fire Knowledge and Group, 2019). Indigenous fire practices largely ceased in the Murray Mallee region following colonization and there is little written information about the extent and timing of Indigenous use of fire. However, there is emerging attention to traditional fire practices across south-eastern Australia (Smith et al., 2021).
A common theme of Indigenous use of fire is the importance of place-based knowledge; that is, including site-specific knowledge of a locality, its history, fire and fuel characteristics and likely impact on biota. For example, in mallee landscapes in Western Australia, the Ngadju people show how use of fire was tailored to particular locations and vegetation types: frequent fire was applied in some areas and others experienced far less fire (Prober et al., 2016). Intimate knowledge of plants and animals guided cultural burning: for example, prior to low severity burning, local areas were checked for important food plants (e.g., fruit trees), animals (e.g., malleefowl nests) and habitat (e.g., logs) (Prober et al., 2016). A link between anthropogenic fire and future fire risk was evident, with Ngadju emphasizing the importance of burning spinifex surrounding important assets (large, old mallee trees) to ensure they were not lost to wildfire (Prober et al., 2016).
Europeans commenced agriculture in the Murray Mallee region in the 1840s, initially by pastoral occupation (Broome et al., 2020). Traditional land-use practices of Indigenous peoples were rapidly curtailed as people were displaced from traditional lands through killing, disease and assimilation policies (Clarke, 2009). Widespread clearing of mallee vegetation commenced in the 1890s, and especially after World War I via soldier settlement schemes to cultivate land for cereal cropping, primarily south of the Murray River (Figure 2). Clearing targeted the most fertile soils; the large blocks of mallee vegetation remaining today (most in conservation reserves, Figure 4) are on less-fertile soils. Clearing of mallee vegetation and destruction of cryptogamic soil crusts through trampling by stock left soils vulnerable to wind erosion, and massive dust storms (e.g., in the 1930s) resulted in loss of valuable topsoil. Farm dams were excavated and created more reliable sources of water for stock and wildlife, but grazing pressure around such points exacerbated vegetation change and loss of topsoil (James et al., 1999). Clearing and fragmentation of vegetation, fire suppression, and introduction of planned burning have all contributed to changes in fire regimes.
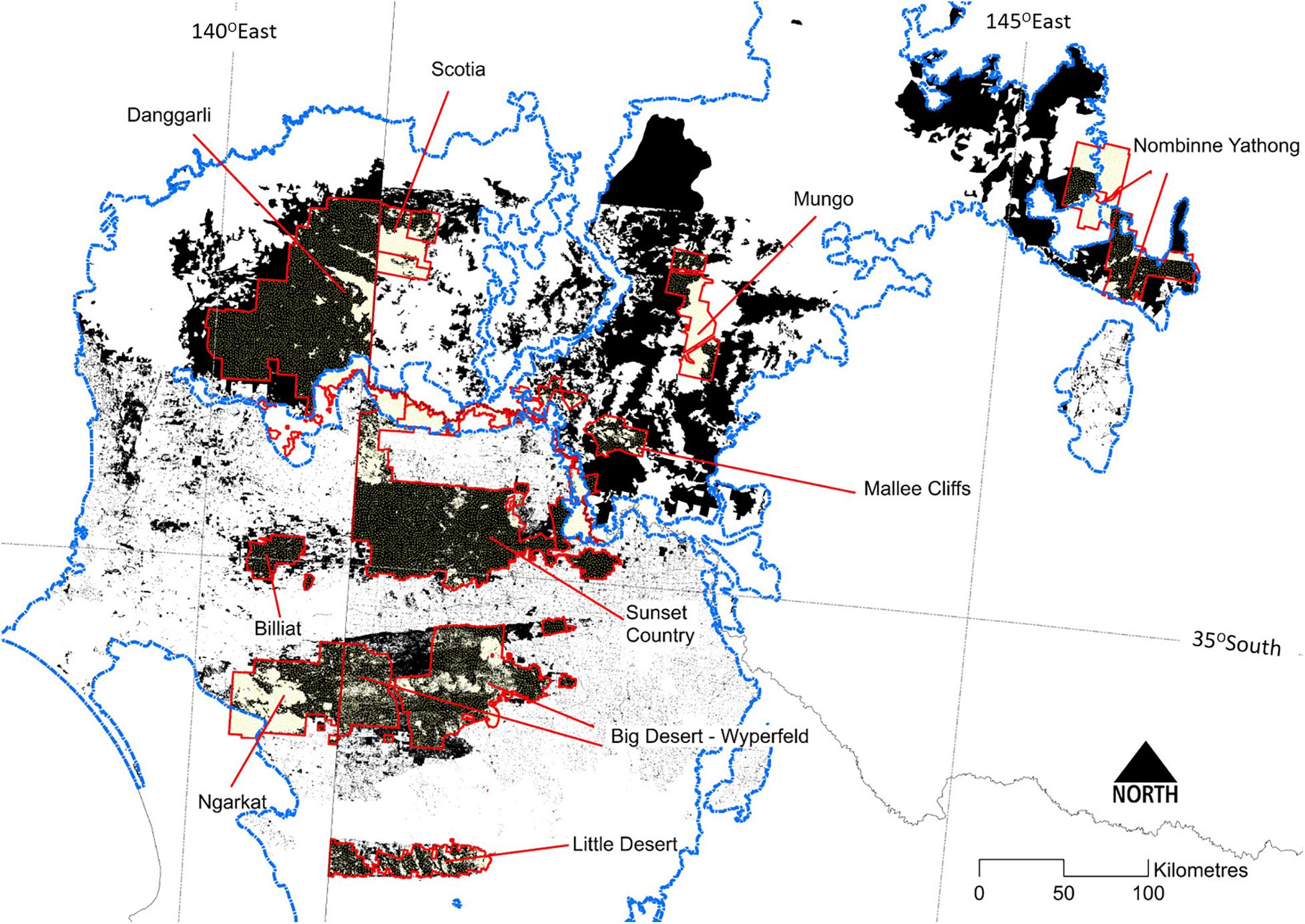
Figure 4. The distribution of extant (dark shading) mallee vegetation in the Murray-Darling Depression Bioregion (Murray Mallee region) (outlined in blue). Major protected areas (including conservation reserves) are bounded in red and shaded pale yellow.
Natural and Anthropogenic Drivers of Fire Regimes
Patterns and Characteristics of Fire in Mallee Ecosystems
The characteristic features of wildfire in mallee ecosystems (Table 1) differ from those in other ecosystems such as temperate forests, tropical savannah or arid hummock grasslands, resulting in more uniform burn severity. This leads to extensive portions of very large fire scars (>10,000 ha) sharing a synchronized stage of recovery post fire. Fires in other ecosystems (e.g., temperate forests) typically encounter more barriers to fire spread, both horizontally and vertically, resulting in greater heterogeneity across the post-fire landscape.
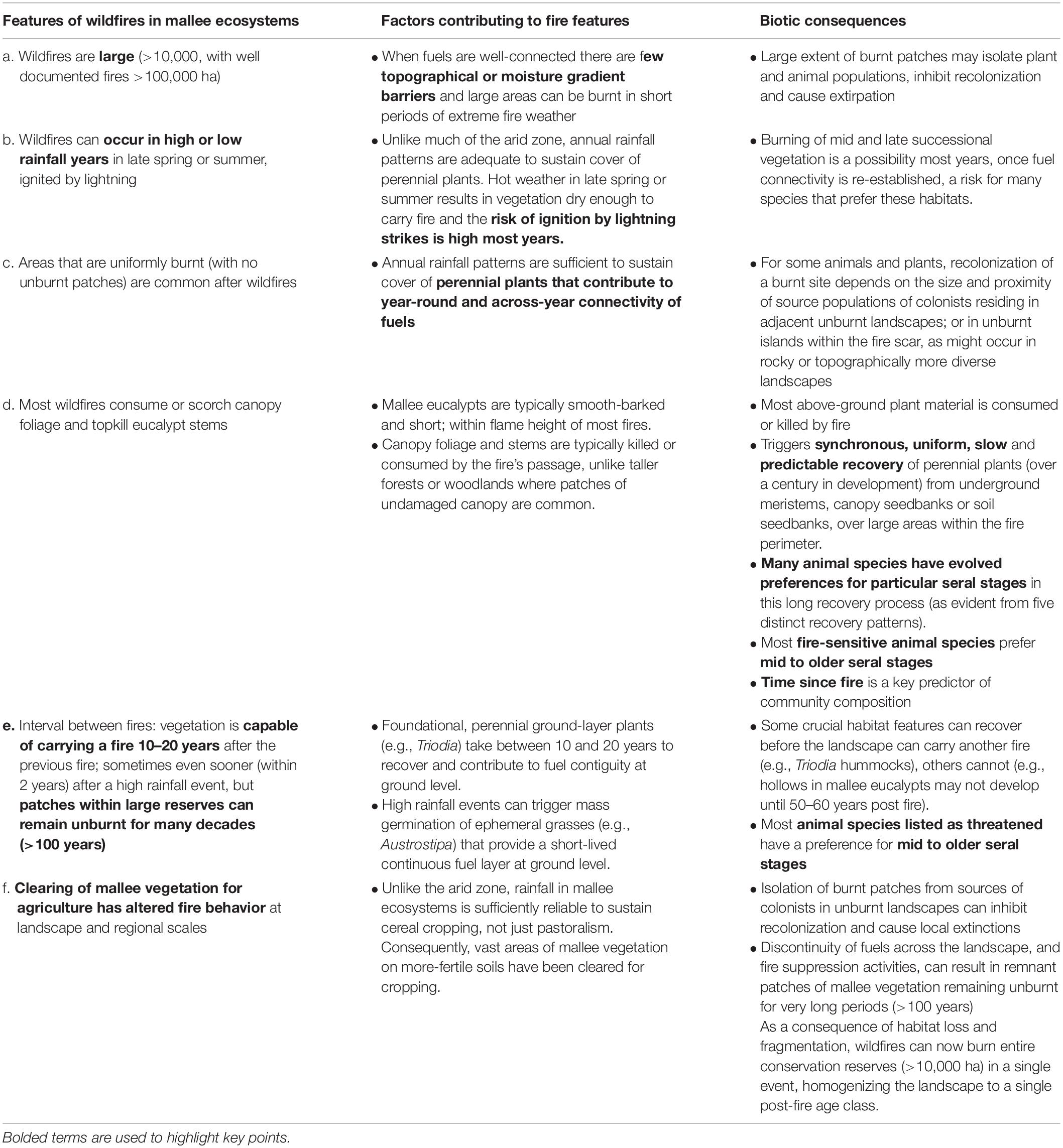
Table 1. Key features of wildfire in contemporary mallee ecosystems, the factors that contribute to those features and the biotic consequences.
Mallee vegetation is highly flammable, and in the Murray Mallee region wildfires can occur in any given year (Bradstock and Cohn, 2002). Rainfall is sufficiently reliable to sustain perennial plants as a fuel source, and dry, hot conditions in summer months increase the probability that vegetation will carry fire ignited by lightning strikes. Wildfires in southwestern Australian mallee tend to be linked to hot and dry conditions, with few or no fires in cool and wet summers (O’Donnell et al., 2011a, 2014).
Leaf and bark litter are key components of fuel in fires in mallee ecosystems (Bradstock and Gill, 1993). The distribution and abundance of litter contributes to the contiguity of fuels between trees and shrubs (McCaw, 1997). Surface fuels often consist of large ‘halos’ of shed bark and leaf litter around each mallee tree, with less fuel between halos. Wind is required for fire to spread between patches of fuel. Without strong wind, fire spread in mallee vegetation, even on hot and dry days, is limited (Cruz et al., 2013). This may partly explain the dominance of large fires in mallee ecosystems (Figure 5); once the fire-spread threshold is overcome, fires spread rapidly. The spatial patchiness of fuels in mallee vegetation increases from south to north with decreasing rainfall (Pausas and Bradstock, 2007; Gibson et al., 2014). Litter (e.g., depth, cover) does not accumulate in a continuous manner over time, but peaks at ∼20–30 years post-fire and then remains at a plateau or subsequently declines with time (Haslem et al., 2011). Ecosystem engineers, such as fossorial mammals (Hayward et al., 2016), termites and the malleefowl remove litter and consume or bury it, thereby reducing fuel contiguity in ways that can have a measurable impact on fire behavior (Smith et al., 2017).
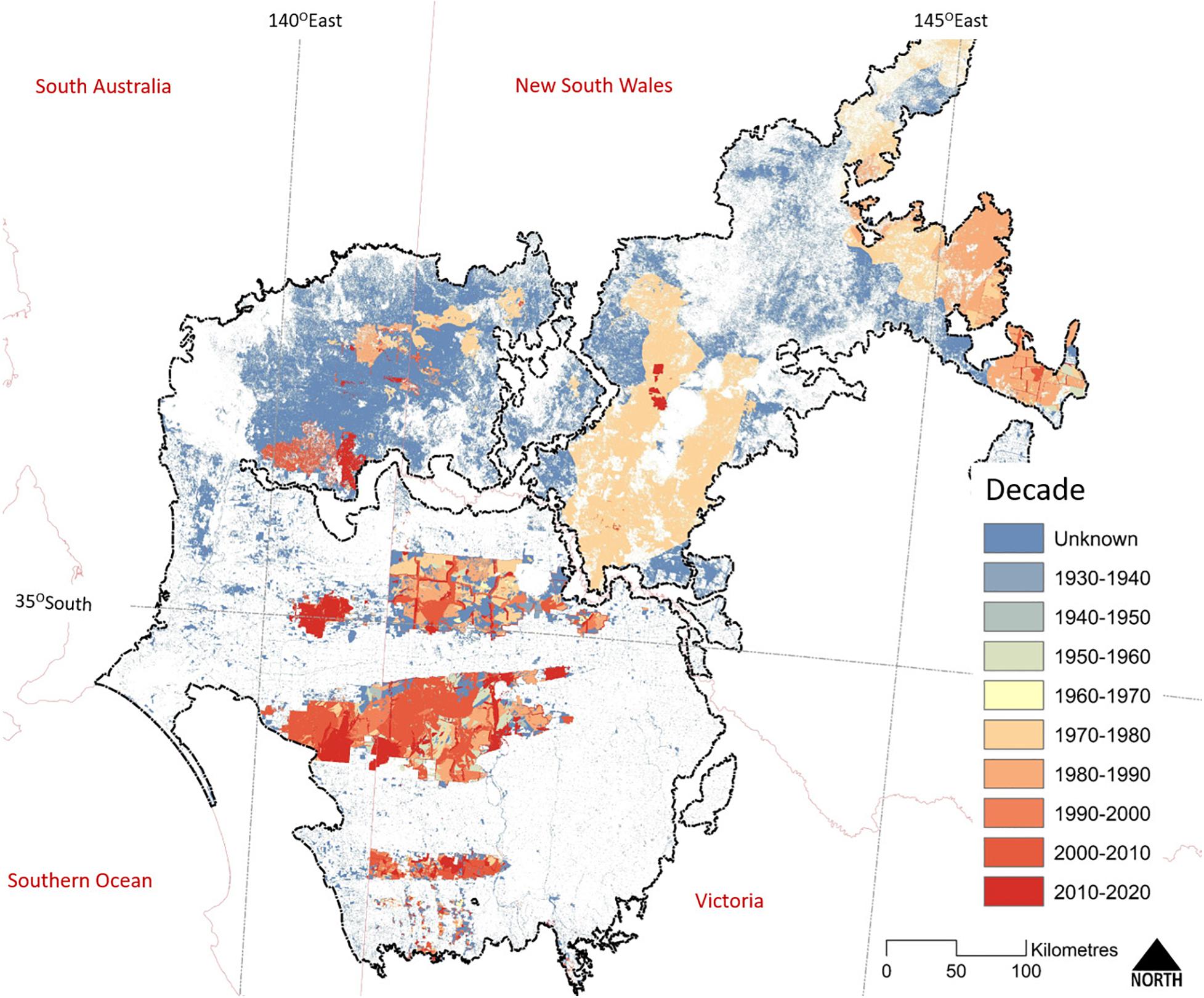
Figure 5. The extent of the last known fire within extant woody vegetation across the Murray-Darling Depression Bioregion (outlined in black). State boundaries are shown in pale red. The earliest recorded fires date from the 1930s; however, reliable and systematic recording of fire extent dates back to the mid-1970s only and correlates with the regular acquisition of cloud-free Landsat imagery. Map compiled from State fire-history datasets: Victoria (Department of Environment Land Water and Planning, 2020), New South Wales (National Parks and Wildlife Service, 2020), and South Australia (Department for Environment and Water, 2016).
Wildfires typically are large (Figure 5), often the result of multiple, lightning-triggered ignitions merging during a single storm event. Systematic mapping of fire using Landsat imagery for a 100,000 km2 study area in the northern Murray Mallee region provided a detailed understanding of contemporary fire history (1972–2007) (Avitabile et al., 2013). Large fires (>10,000 ha) occurred regularly (e.g., 16 between 1972 and 2007), with very large fires (>100,000 ha) occurring every 10–20 years; sometimes following drought and sometimes major rainfall events (as is also seen in West Australian mallee, O’Donnell et al., 2011b, 2014). Smaller fires (<10,000 ha, generally <100 ha) occur every year. Over this 35-year period, an area equivalent to 40% of mallee vegetation in the study area was burnt, of which large fires (>10,000 ha) were responsible for more than 89% of the area burnt.
The interval between wildfires can be long, due to the slow rate of fuel recovery (O’Donnell et al., 2014). Chenopod and Triodia Mallee vegetation are capable of carrying a fire 10–20 years after the previous fire; much sooner (within 2 years) if high rainfall has stimulated widespread growth of ephemeral grasses (Noble and Vines, 1993; O’Donnell et al., 2011a, 2014). Heathy Mallee vegetation can burn in less than 10 years after a previous wildfire, as is evident in the higher fire frequency in Ngarkat Reserve (Figure 6). Nevertheless, patches within large reserves can remain unburnt for many decades (>100 years, Clarke et al., 2010). Fire mapping for 1972–2007 (Avitabile et al., 2013) showed that despite the regular occurrence of large fires, less than 3% of mallee vegetation experienced more than one fire during this 35-year period. Pausas and Bradstock (2007) estimated the fire cycle for conservation reserves in the region (number of years to burn an area equal to the reserve) to be between 125 and 206 years.
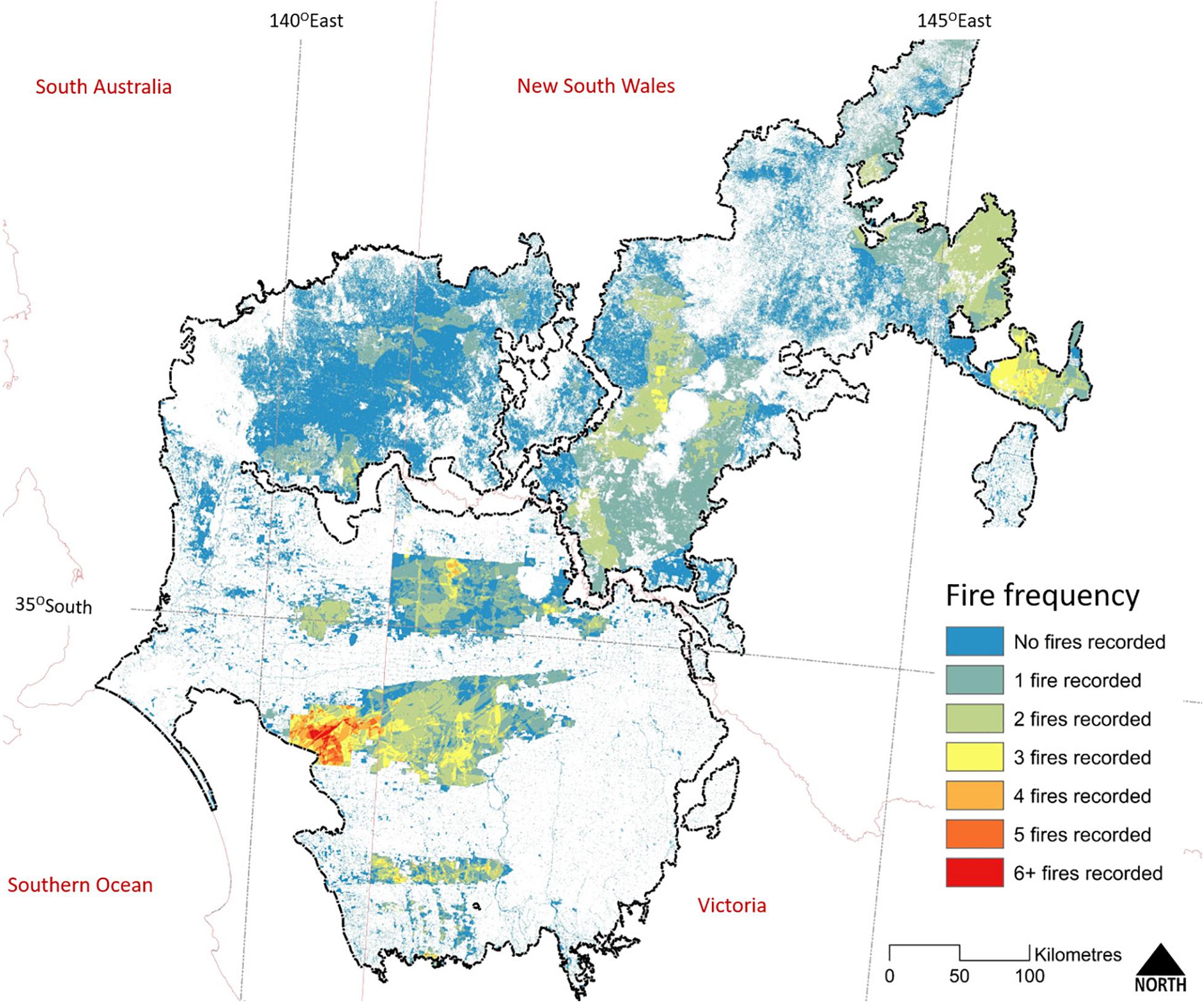
Figure 6. The frequency of known fires within extant woody vegetation across the Murray-Darling Depression Bioregion (outlined in black). State boundaries are shown in pale red. The earliest recorded fires date from the 1930s; however, reliable and systematic recording of fire extent only date back to the mid-1970s and correlates with the regular acquisition of cloud-free Landsat imagery. Map compiled from State fire history datasets as for Figure 5.
Most wildfires burn with high severity (Figures 7A,B) (Clarke et al., 2010). Mallee eucalypts are short, thin-barked and many have strips of decorticating bark that bridge the vertical gap between ground fuels and canopy. Flame heights of ground-level fires readily scorch or consume the eucalypt canopy (Bradstock and Gill, 1993) and ‘topkill’ stems (Figure 7B). Thus, wildfire usually triggers a replacement of standing eucalypt stems, unlike many taller, mesic eucalypt forests and woodlands where patches of undamaged canopy within fire scars are common (Leonard et al., 2014). The homogeneous severity of wildfire is highest in areas with few topographic or moisture gradients to inhibit fire spread (such as sandplains of Triodia Mallee), although some unburnt patches do occur (Berry et al., 2015). Wildfire in contemporary mallee ecosystems typically produces a coarse-grained mosaic of large blocks of vegetation of a common seral stage (10,000s of ha in area), adjacent to other large blocks of vegetation of different seral stages (Figures 5, 7A).
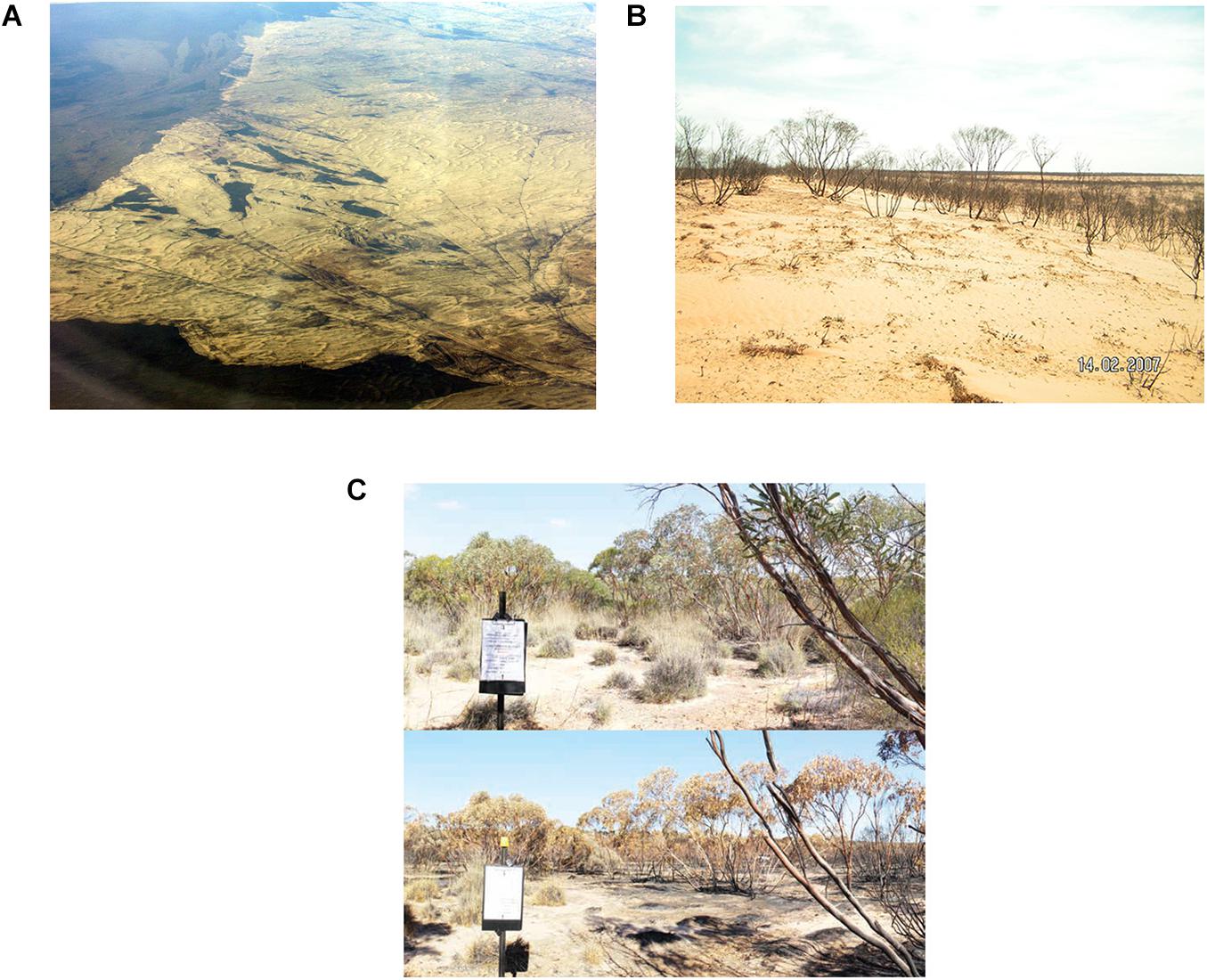
Figure 7. (A) Aerial view of a large wildfire scar in the Big Desert, Victoria. (B) Ground-level view of Triodia Mallee recently burnt by wildfire. (C) Photopoint images of a site in mallee vegetation before and after a planned fire, conducted for experimental purposes.
In addition to wildfires, land managers conduct planned burns during late autumn and early spring when climatic conditions enhance the controlled use of fire (e.g., Sandell et al., 2006; Figure 7C). These burns aim to impede the spread of future wildfires under more-severe conditions. They may include: (a) small scale ignition of individual Triodia hummocks, applied on foot, in a 50–100 m strip beside a track to remove ground-level fuels without consuming the canopy of mallee eucalypts; (b) air- or ground-ignited strategic strips several kilometers wide and tens of kilometers long that are lit from existing fire scars to reduce both ground layer and canopy fuels; and (c) patchy burning of blocks of mallee vegetation (100s ha in area) with the aim of removing ground and canopy fuels in parts of the landscape. In some cases (e.g., in Heathy Mallee), planned burns may also have an additional ecological goal, such as to trigger regeneration of serotinous plants.
Drivers of Fire Regimes
The extent and configuration of wildfires in mallee vegetation depend on multiple factors: fire-weather conditions, fuel load, and the spatial arrangement and contiguity of fuels (Bradstock and Cohn, 2002). While interactions between these variables create some heterogeneity in fire severity, extensive burnt areas are common (Avitabile et al., 2013).
‘Fire weather’ conditions – the combination of climatic trends and daily weather that increases the likelihood of large fires – include lightning-inducing weather changes, high temperature, low humidity, and strong gusty wind changes (Luke and McArthur, 1978; Long, 2006). Days of extreme fire weather result in vegetation dry enough to act as a continuous combustible fuel layer, prone to ignition by lightning strikes (Cheal et al., 1979; Bradstock and Cohn, 2002). In the Murray Mallee region, days of extreme fire weather occur in late spring through summer (November to March), and most fire events coincide with dry winds from the north to northwest, followed by south-westerly changes (Long, 2006).
Irregular pulses of above-average rainfall (e.g., once or twice a decade) are a driver of short-term availability of fuels due to widespread, increased cover of ephemeral grasses (Figure 8B) (Ludwig et al., 1990; Gibson et al., 2014, 2015). When dry, such grasses increase both the quantity and, particularly, the contiguity of fuels. In the absence of an ignition source these fine fuels are consumed by herbivores or detritivores. However, some of the largest fires in the region (e.g., 658,000 ha across New South Wales and South Australia in 1976) have occurred in summer months following a period of high rainfall (Noble et al., 1980; Avitabile et al., 2013). Similar patterns of large fires following wetter than average conditions in the preceding year have also been reported in southwestern Australia (O’Donnell et al., 2011a).
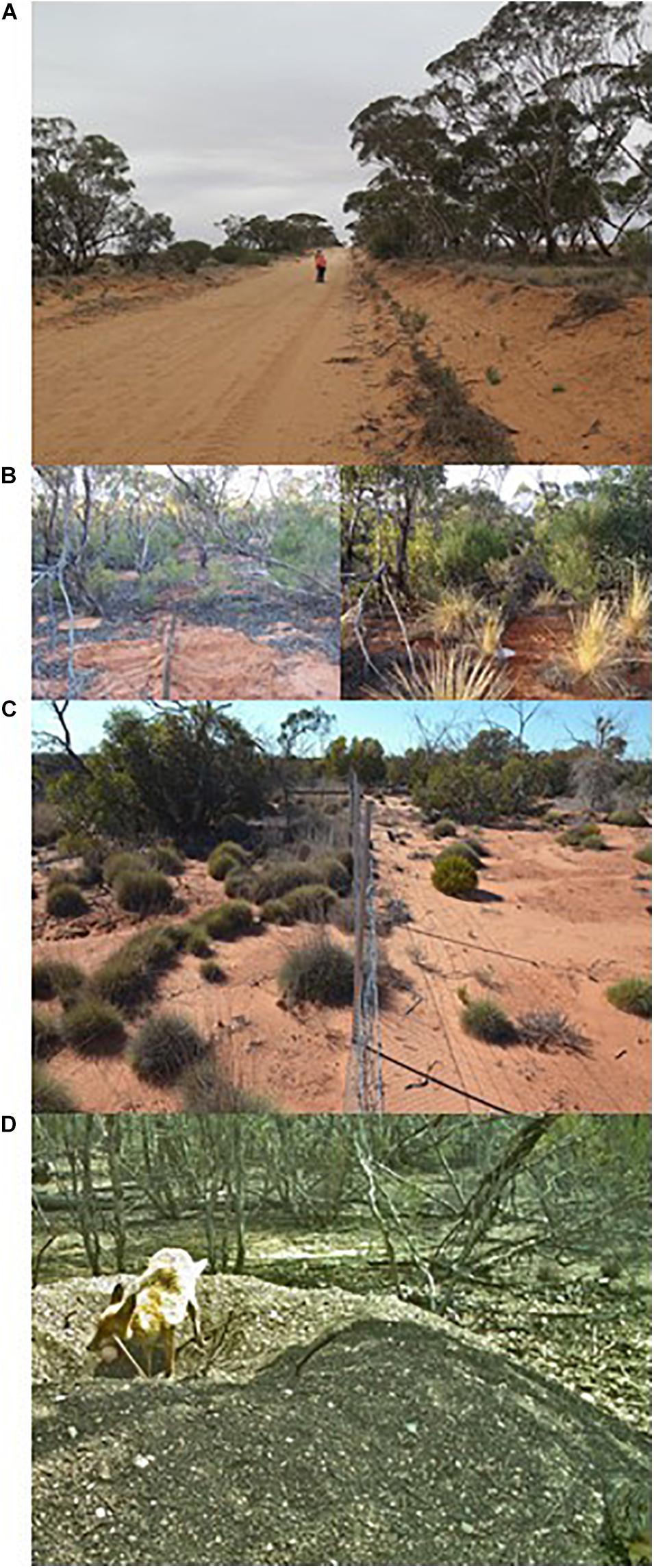
Figure 8. Illustrations of drivers that interact with fire in mallee ecosystems. (A) Fragmentation of mallee vegetation for cereal cropping, resulting in long unburnt linear corridors. (B) Contrasting levels of Austrostipa and shrub cover in drought (2007, left) and after high rainfall (2010–2011, right) in Tarawi Nature Reserve. (C) Fence line comparison in Tarawi Nature Reserve showing vegetation differences in 2013 due to greater herbivory by mammals on right hand side of fence, 7 years after both sides of the fence had been burnt (photo courtesy David Keith). (D) Exotic predator (red fox) preying upon the egg of an ecosystem engineer (malleefowl – partially visible in lower left of frame). Photo courtesy of Riverina Local Land Services.
Stands of some plant species (e.g., Callitris, Allocasuarina, Triodia) can influence the passage of fire and hence the fine-scale patchiness and occurrence of unburnt patches. For example, dense stands of Callitris verrucosa that establish in Heathy Mallee vegetation after decades without fire (White, 2006) appear to impede the spread of fire due to transformation of the litter layer (Bradstock, 1989b) and possibly by reducing wind strength (Cruz et al., 2013). Such stands have the potential to create unburnt fire shadows in their lee (Benshemesh, 1990). In contrast, Triodia hummocks enhance the passage of fire by connecting fuels across the landscape (Dodson, 1982).
How Do Fire Regimes Shape Biodiversity?
Fire regimes shape biodiversity in mallee ecosystems at multiple scales: at the local scale (e.g., 10s of ha) by triggering post-fire secondary succession, and at landscape (1000s–10,000s ha) and regional scales (100,000s ha) through the effects of spatiotemporal variation on the distribution of species. We first summarize knowledge of the response of plant and animal species at the local scale to two key aspects of the fire regime, time since last fire and fire interval, and then consider how spatial patterns of fire at the landscape and regional scale can influence the biota.
Responses to Time Since Fire and Inter-fire Interval
Vegetation
Plant species of mallee vegetation possess life-history traits that enable persistence through recurrent disturbances. Among woody species, obligate seeders predominate (Clarke et al., 2015) and the majority have soil-stored seed banks (e.g., Acacia, Senna, Beyeria). Serotiny (canopy seed storage) is relatively uncommon in the Murray Mallee region (e.g., Callitris and Hakea spp., Bradstock and Cohn, 2002). Traits vary along the rainfall gradient: with decreasing rainfall the proportion of obligate seeders increases while resprouting species decrease (Pausas and Bradstock, 2007).
Fire drives the structure of mallee vegetation (e.g., Gosper et al., 2012b). Wildfires topkill or consume above-ground vegetation, thus vegetation structure changes in a synchronous and relatively predictable way with time since fire as recovery occurs. Such a synchronized structural response to time since fire is more prevalent in mallee vegetation than in temperate forests where, for many tree species, epicormic re-sprouting along trunks and limbs ensures rapid recovery of the canopy after fire (Rainsford et al., 2020). Key structural components strongly influenced by time since fire include mallee eucalypts, understorey vegetation (e.g., chenopods and perennial grasses like Triodia scariosa), extent and cover of litter, and structural features such as tree hollows (Haslem et al., 2012; Kenny et al., 2018).
Recruitment of mallee eucalypt seedlings following fire is rare, unlike many other Eucalyptus species, despite the seeds being protected from fire in woody capsules: harvesting of seeds by ants may be a factor (Wellington and Noble, 1985). It is not clear what combination of triggers is needed for successful establishment of seedlings, but fire alone is insufficient. Competition with resprouting adults may also inhibit establishment of seedlings (White et al., 2003). Post-fire recruitment of eucalypt seedlings may be greater when above-average rainfall follows a fire (Wellington and Noble, 1985) and grazing pressure is low (Westbrooke and Florentine, 2005). Established mallee eucalypts typically recover from fire by sprouting dozens of stems from below-ground lignotubers (Noble, 2001). The number of stems per lignotuber declines with time since fire, accompanied by a steady increase in the height of canopy foliage (Haslem et al., 2011; Kenny et al., 2018). A decline in the density of trees with time since fire (e.g., Chenopod Mallee), and associated increases in spacing, result in reduced contiguity of fuels. In the absence of fire, or where isolated individual trees escape damage from fire, stems reach much greater sizes.
Time to senescence of mallee eucalypts is not known. Above-ground stems over 200 years in age can appear healthy and underground lignotubers may be many centuries old (Tyson et al., 1998). A difficulty in determining longevity is that there are few stands of known post-fire age greater than 100 years. Fire mapping based on satellite imagery is available only from 1972 (Avitabile et al., 2013), and the precision of other methods (e.g., modeled relationships between stem diameter and age) diminishes greatly beyond 90 years post-fire (Clarke et al., 2010; Callister et al., 2016). The nature of successional change in mallee vegetation after prolonged absence of fire (e.g., hundreds of years) is not clear. Heathy Mallee can become dominated by Callitris verrucosa as other shrubs (e.g., Banksia spp.) senesce (Cheal, 2010).
Recruitment of seedlings of grass, herb and shrub species occurs within the first 2 years after fire (Noble et al., 1980; Wilson et al., 1988). In Triodia Mallee, this response is generally transitory and Triodia becomes the dominant species after ∼5 years (Noble et al., 1980; Pickett et al., 1987; Letnic et al., 2004). This pattern differs in central NSW and the Eyre Peninsula, where Triodia increases after fire but shrubs remain dominant throughout (Gibson et al., 2014, 2015). The cover of Triodia hummocks increases steeply from ∼ 5 to 20 years post-fire (Haslem et al., 2011; Kenny et al., 2018), with maximum cover at ∼30 years since fire. With increasing age, cover may decline or reach a plateau, depending on soil type and topographic position (Verdon et al., 2020). The growth form of this dominant species also changes through time: with increasing post-fire age (∼30 years), hummocks may die in the center but continue peripheral growth, thus forming a ‘ring’ of live plant material with dead material in the center. The proportion of plants occurring in ‘ring form’ reaches a peak at ∼50 years, though hummock-form plants occur throughout the chronosequence (Kenny et al., 2018).
Stems of mallee eucalypts typically are killed by wildfire. Stem mortality is also common after high-intensity planned burns, but not all are fully consumed; some dead stems remain standing. Over time, dead stems fall and become logs, peaking in density 10–20 years after fire (Haslem et al., 2011). If the interval between the two most recent fires is long (e.g., >80 years), the diameter of these stems will be large when killed by fire. Such large dead stems can remain standing for decades and provide valuable nesting and roosting hollows for fauna (Figure 9). The availability of hollows provided by dead stems increases with the interval between fires (Haslem et al., 2012). Living stems typically do not develop hollows suitable for fauna until >60 years post-fire (Haslem et al., 2012). Similarly, slow development of key structural attributes has been reported in southwest Australian mallee (Gosper et al., 2012b). Although small hollows become larger over time, many are lost as stem densities decline with time since fire. Consequently, for species using medium (4.0–9.9 cm entrance diameter) and large hollows (≥10 cm), such as parrots, owlet nightjar (Aegotheles cristatus), larger reptiles and bats, there is a very long period before this resource becomes available, coupled with extensive areas of younger fire age-classes where hollows are effectively absent (particularly in Triodia Mallee).
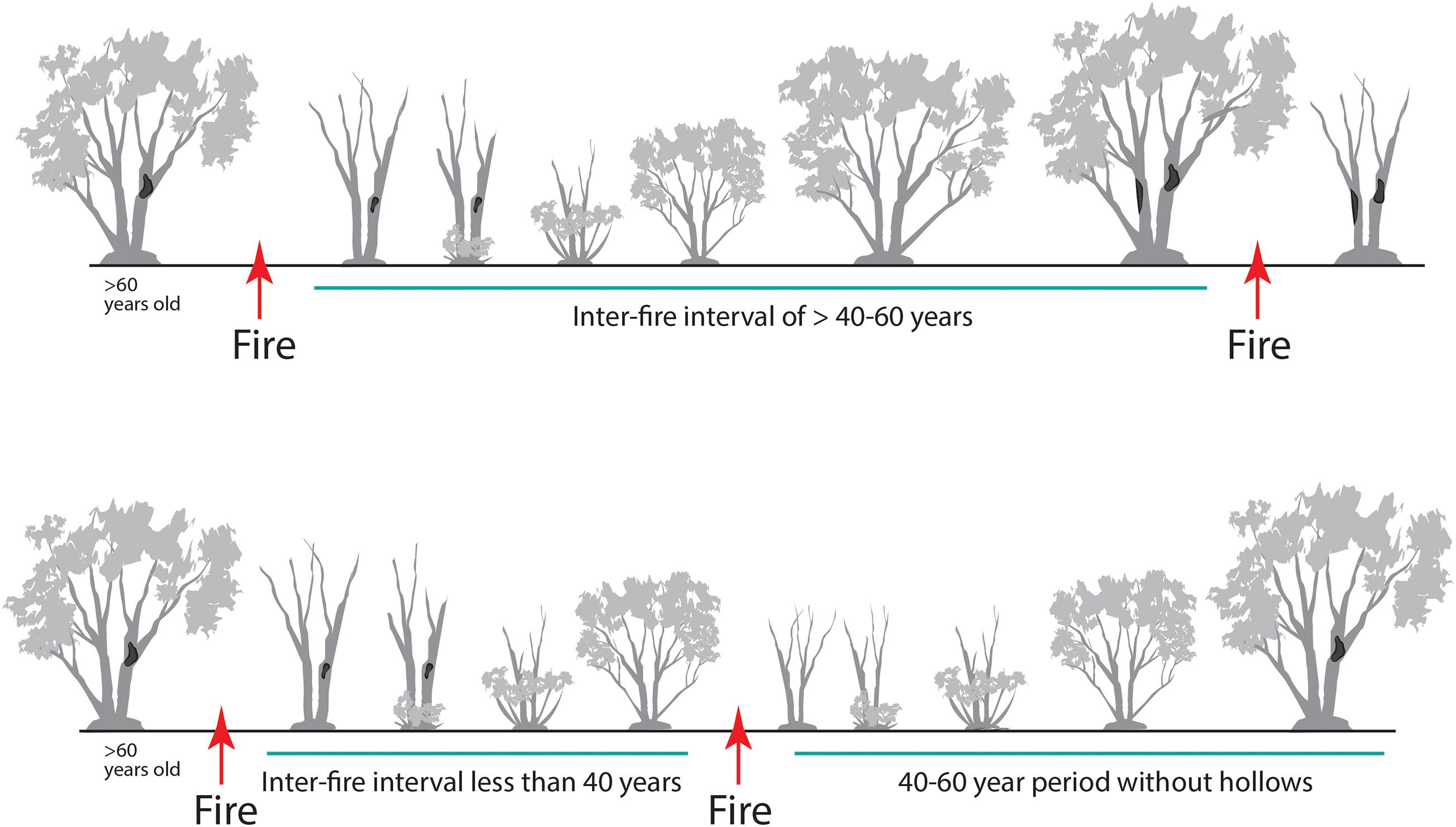
Figure 9. A graphical model of changes in availability of large dead trees and tree hollow occurrence (dark patches on stems) with variation in inter-fire interval. Intervals that are too brief (<40 years) result in fewer hollows developing in live stems prior to the next fire, and fewer (dead) stems containing hollows persisting after the next fire.
The interval between fires may also affect the persistence of plant species. For example, simulated fire intervals that were too short (<20 years) or long (>50 years), both resulted in reduced population size of the obligate seeder Callitris verrucosa due to the elimination of juveniles and senescence of adults, respectively (Bradstock et al., 2006). In southwest Australia, mallee communities dominated by obligate seeders are less resilient to variation between inter-fire intervals than communities dominated by resprouters (Keeley, 1986; Gosper et al., 2012a, b). Considerable variability in the rate at which seed banks accumulate (both above and below ground) has been reported among geographically dispersed populations of obligate seeders, making determination of minimum and maximum tolerable fire intervals challenging (Gosper et al., 2013). Inter-fire interval can also influence the above-ground occurrence of plant species (Avitabile, 2014). The probability of occurrence for 14 of 43 modeled plant species was significantly affected by inter-fire interval. Nine species showed increased probability of occurrence when time between successive fires was long, with some species (e.g., Maireana pentatropis, Roepera apiculata, and R. aurantiaca) becoming more prevalent when the interval between fires is greater than 60–80 years (and still increasing after 90 years). Conversely, the probability of occurrence declined for three species (Acacia sclerophylla, Daviesia benthamii, and Sclerolaena diacantha) when the inter-fire interval was greater than 40–60 years.
Animals
With changes in vegetation composition and structure over time (Figure 10), the suitability of mallee vegetation as habitat for many animal species also changes (Friend, 1993; Kelly et al., 2010, 2011; Nimmo et al., 2012; Watson et al., 2012b; Smith et al., 2013, 2016). Time since fire is an important predictor of the occurrence of numerous species. Here, we identify six generalized responses to time since fire (Figure 11), based on occurrence of vertebrate species across a chronosequence of 100 years.
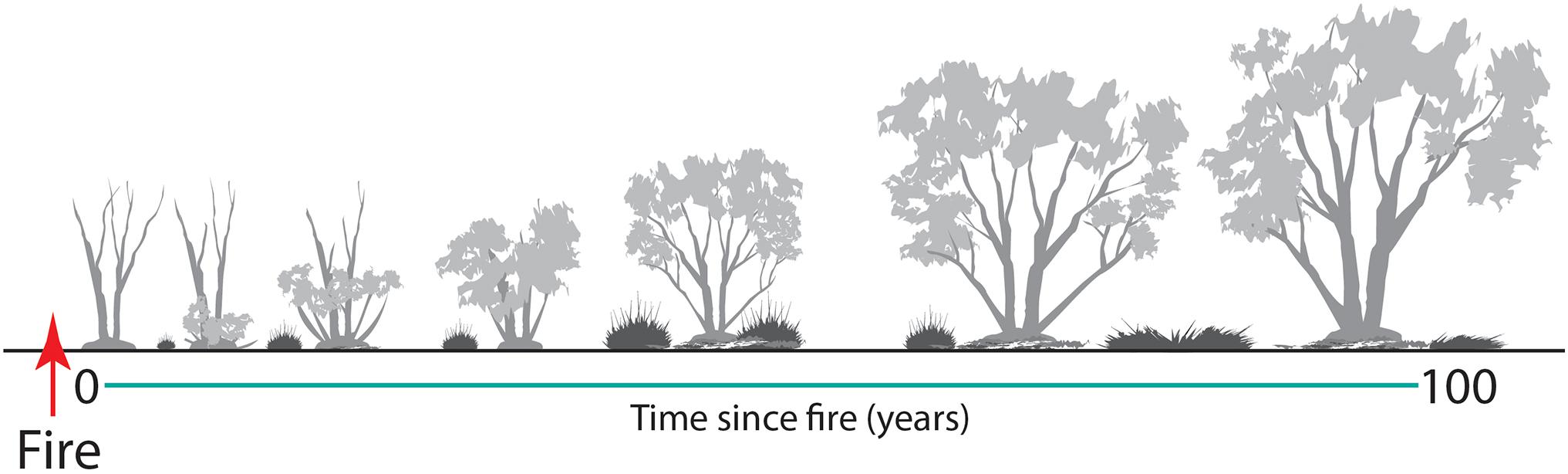
Figure 10. A graphical model of structural changes in Triodia Mallee over time since fire. Highlighted is the post-fire development of mallee stems from an underground lignotuber, and changes in Triodia cover, litter cover and canopy height.
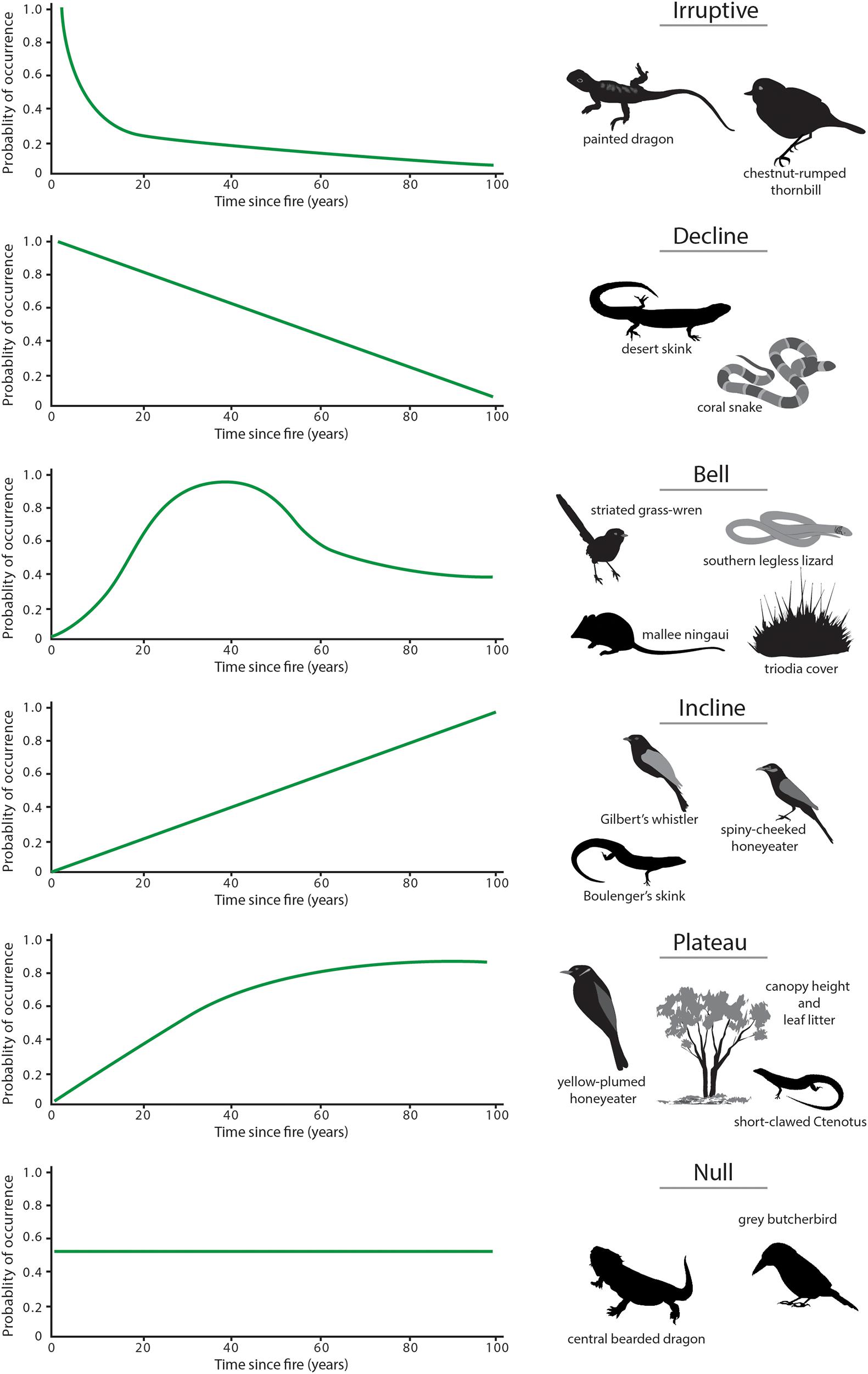
Figure 11. Idealized responses of mallee fauna to time since the last fire. ‘Response curves’ represent six discrete patterns of the change in the probability of occurrence of a species with time since the last fire over a 100-year post-fire chronosequence: ‘irruptive,’ ‘decline,’ ‘bell-shaped,’ ‘plateau,’ ‘incline,’ and ‘null’ (or no clear response). Images are of exemplar species that showed these responses.
(a) Irruptive: Species that occur with greatest frequency of occurrence in early post-fire vegetation (<10 years since fire) tend to be those that burrow, or favor open spaces or bare ground for foraging, as the extent of such areas is high immediately after fire. Examples include the painted dragon (Ctenophorus pictus) and the insectivorous bird, chestnut-rumped thornbill (Acanthiza uropygialis). These species decline rapidly in occurrence in mid and older seral stages.
(b) Decline: These species, such as the desert skink (Liopholis inornata) and coral snake (Brachyurophis australis), also decline in occurrence with increasing time since fire, but in a more gradual manner (Figure 11). This response reflects the habitat becoming increasingly less suitable as post-fire succession proceeds.
(c) Bell: Species with this response type typically are associated with habitat attributes that reach their peak in mid-successional stages post-fire. For example, the southern legless lizard (Delma australis), the mallee ningaui and the striated grasswren are each closely associated with Triodia hummocks as habitat for shelter, refuge, and foraging. Their probability of occurrence peaks in mid-aged vegetation (∼20–40 years since fire) and is lower in younger and older vegetation, coinciding with reduced cover and complexity of Triodia.
(d) Incline: Species that display an incline response, such as the spiny-cheeked honeyeater (Acanthagenys rufogularis), striped honeyeater (Plectorhyncha lanceolata), Gilbert’s whistler (Pachycephala inornata) and southern scrub-robin (Drymodes brunneopygia), respond to post-fire habitats that continue to increase in complexity with time. The occurrence of such species showed no evidence of reaching a plateau – at least over the century time-scale studied.
(e) Plateau: Species that show a plateau response to fire (e.g., Murray striped skink Ctenotus brachyonyx, yellow-plumed honeyeater, Gould’s wattled bat Chalinolobus gouldii) have a low probability of occurrence in early post-fire succession, reach an asymptote in mid-age vegetation and then remain at similar abundance in older vegetation. For the yellow-plumed honeyeater, for example, this pattern corresponds with the growth form of mallee eucalypts and abundance of attributes such as canopy cover, stem size and bark cover (Haslem et al., 2011), which provide foraging substrates.
(f) Null: In all groups studied, a substantial number of species show no significant change in probability of occurrence with time since fire; for example, 14 of 30 species of birds (Watson et al., 2012b) and 6 of 17 species of reptiles (Nimmo et al., 2012) modeled for the Murray Mallee region.
The response of species to time since fire may also vary between vegetation types and spatially between geographic areas (Nimmo et al., 2014). For example, of 17 species of reptile in the Murray Mallee region with sufficient data to model, 11 species displayed significant relationships with time since fire: nine species in Triodia Mallee, four species in Chenopod Mallee and only two in both (Nimmo et al., 2012). Similar observations were evident for bird species (Watson et al., 2012b). These results can be attributed to differences in vegetation composition and habitat attributes between vegetation types, and to differences in flammability.
There is a risk that the capacity to detect some species varies with time since fire and may influence apparent abundance and therefore fire response patterns (Driscoll et al., 2012, 2020). For example, some cryptic reptiles become more active after fire, increasing trap rates within a time frame too short to represent change in actual density. Such species can exhibit an apparent irruptive response, but this may be due to greater detectability, presumably related to reduced shelter opportunities and changes in prey availability (Driscoll et al., 2012). In contrast, some species may have behavioral and physiological adaptations, such as torpor, that allow them to avoid starvation or predation following fire by sheltering in place (Geiser et al., 2017; Nimmo et al., 2019). This could lead to lower detectability immediately following fire and being recorded as absent at sites where they remain but are inactive. Consequently, some uncertainty around the shape of species’ responses to time since fire is difficult to resolve.
Less is known on how invertebrates respond to time since fire. In the Murray Mallee, an examination of 21 species of invertebrates from key groups (psyllids, termites, scorpions, centipedes) in Triodia and Chenopod Mallee did not detect any species exhibiting a significant response across a 100-year chronosequence (Avitabile, 2010). Many termite species nest in subterranean burrows and appear resilient to fire (Avitabile et al., 2015; Davis et al., 2018). In mallee vegetation of the Eyre Peninsula, South Australia, Teasedale et al. (2013) found no difference in abundance between post-fire age classes for 15 orders of invertebrates; but, at a finer resolution, 17 morphospecies did show such variation. Two closely related Lycosid spiders showed contrasting responses: one taxon was most abundant in early succession (4–5 years) and the other in later succession (>40 years), indicating the importance of a (morpho)species-level approach rather than broad categories (Teasedale et al., 2013). Similarly, in mallee-heath shrublands of southwestern Australia, Friend and Williams (1996) found that variation in the abundance of most Orders of invertebrates was not strongly related to fire. However, Coleoptera and Diptera were most abundant >40 years post-fire and Hemiptera and Orthoptera peaked in earlier seral stages.
While recent studies have advanced knowledge of how animal species respond to time since fire, little is known of the effects of repeated fires on their distribution and abundance in mallee ecosystems. It is likely that interactions between fire interval (or fire frequency) and vegetation structure influence habitat suitability, with mammals being the most well-studied group to date (Senior et al., 2021). For example, fires at shorter intervals (<40 years) that reduce the abundance of dead stems with hollows, reduce the suitability of habitat for species that depend on tree cavities, such as the south-eastern long-eared bat (Nyctophilus corbeni) (Lumsden et al., 2008; Senior et al., 2021).
Key challenges for future studies are to better understand: (a) how extreme intervals (too long or too short) influence the persistence of plants (see Gosper et al., 2013); (b) how repeated fires (and fire interval) influence animal populations; (c) how the seasonal timing of fires and (d) post-fire seasonal and annual rainfall patterns affect plants and animals.
Responses to Spatial Patterns of Fire
Fire creates a spatial pattern of burned areas and unburnt patches of different sizes and shapes (Figure 7A). The characteristics of fire (Table 1), the vertical connectivity of fuels and the limited topographic relief in mallee ecosystems mean that vegetation typically burns completely or else remains unburnt (O’Donnell et al., 2011a), rather than generating a fine mosaic of differing levels of fire severity at a local scale as commonly occurs in forest ecosystems with greater rugosity (e.g., moisture and flammability differences between ridges, slopes and gullies). Over time, additional fires occur across the landscape, thus creating a coarse-grained ‘visible mosaic’ comprising large (100s and 1000s ha) stands or patches of different age since the last fire (e.g., Figure 7A). Additional spatial complexity is created by the interval between fires: for example, two stands of mallee vegetation, both 5 years since fire, may differ in subtle ways if the interval since the previous fire was 10 years for one and 80 years for the other. The term ‘invisible mosaic’ refers to these spatial differences that reflect the temporal history in the landscape, but which are not necessarily visually apparent (Bradstock et al., 2005).
Spatial patterns of fire affect the biota of mallee ecosystems in several ways. The size, shape and patchiness of a fire affect the context of sites, both those burnt and those that remain unburnt. While individuals of some species (e.g., fossorial reptiles) can persist on a burnt site, a key issue for many plants and animals is the capacity to recolonise post-fire, either from internal sources within the fire boundary or external sources (Bradstock et al., 2005; Nimmo et al., 2019; Senior et al., 2021). For example, Watson et al. (2012a) found sites distant from unburnt vegetation supported fewer bird species, but those that contained small unburnt patches supported more species than uniformly burnt sites. Unburnt patches within the fire boundary (Figure 7A) may act as refuges where organisms can escape the fire or may assist animals to recolonise post-fire. Berry et al. (2015) found the number of bird species increased with the size of unburnt patches (1–12 ha in size) remaining in a burnt mallee landscape, but these patches had fewer bird species than large unburnt tracts outside the fire boundary. Together, these results suggest that large fires of uniform severity that homogenize landscapes to a single fire age-class may reduce faunal diversity, even of mobile taxa like birds.
Based on the observation that different species favor different stages in post-fire succession, it has been hypothesized that landscapes that have a greater diversity of post-fire age classes (‘pyrodiversity’) should also have a greater biodiversity (Bradstock et al., 2005; Parr and Andersen, 2006). In one of the first empirical examinations of spatial and temporal variation in pyrodiversity, faunal communities in the Murray Mallee were surveyed in a series of 28 ‘landscapes’ (each 12.5 km2 in area) that differed in the diversity of post-fire age-classes and extent of long-unburnt vegetation. Contrary to expectations, a positive association between species richness and the diversity of post-fire age classes was not detected at this scale, for either birds, mammals or reptiles (Kelly et al., 2012; Taylor et al., 2012; Farnsworth et al., 2014). Key influences on biodiversity included the proportion of older vegetation (>35 years-since-fire) in the landscape (birds, and the mallee ningaui) (Kelly et al., 2012; Taylor et al., 2012), environmental gradients and biogeographic barriers (reptiles) (Nimmo et al., 2013; Farnsworth et al., 2014) and recent rainfall history (rodents and pygmy-possums) (Kelly et al., 2012). Lack of a strong relationship with age-class diversity may be attributed to several factors, including that few of these faunal species occur exclusively in a particular age-class and that there are few species entirely restricted to early successional vegetation.
These findings highlight the complexity of fire management in semi-arid mallee and other ecosystems. Clearly, species show different responses to time-since-fire. Some require a minimum area of a particular post-fire age class(es) (unknown for most species) to be present to persist in the landscape. For such species, there needs to be other suitable patches developing within dispersing distance for the time in the future when the patch(es) they currently occupy are no longer suitable. Therefore, a spatial diversity of post-fire age-classes is required at larger scales (e.g., a large conservation reserve or regional network of reserves comprised of many landscape elements) to ensure a progression of seral stages will be available within dispersal distance for all species, in the long term. Modeling to identify an ‘optimum’ mix of fire age-classes in the Murray Mallee region indicated that a desirable mix of fire histories for biodiversity conservation includes a combination of early, mid and late post-fire age-classes, weighted toward late seral stages (Kelly et al., 2015). Detailed modeling of a wider range of seral stages clarified a preference for vegetation 11–35 and >80 years post-fire that provides critical habitat for many threatened species (Giljohann et al., 2017a). However, a key challenge for future studies is to understand how the spatial configuration of fire age-classes (patch ages, sizes, and proximity) influences species responses because, generally, little is known about dispersal. A second challenge is how such knowledge can inform management actions.
How Do Interactions Between Fire and Other Drivers Shape Biodiversity?
Habitat Loss and Fragmentation
Fire regimes and the loss and fragmentation of habitat interact in three main ways (Driscoll et al., 2021): (a) fire can be an agent of habitat loss and fragmentation, whereby fire removes or fragments habitat, or creates and connects habitat; (b) habitat loss and fragmentation can influence fire, with a consequence being that fire regimes are altered (e.g., changes to fire ignition and spread; Parsons and Gosper, 2011); and (c) the two do not influence each other, but fire and fragmentation interact to affect the responses of the biota.
In mallee ecosystems, extensive wildfire can remove and fragment habitat for species that depend on mid to late successional vegetation or attributes associated with these stages. For example, for species strongly associated with habitat components such as Triodia hummocks, canopy foliage or deep litter layers, fire results in loss of habitat until it is replaced by successional regrowth (e.g., Kelly et al., 2010). Extensive fire can result in fragmentation of a species’ distribution into localized populations, separated by unsuitable, early successional vegetation or farmland. This occurs, for example, for the mallee emu-wren, a small passerine dependent on mid-successional Triodia Mallee vegetation. Brown et al. (2013) found limited genetic structure within this species when it occupied continuous mallee landscapes; attributed to populations persisting via dispersal of individuals and colonization of temporally dynamic patches of suitable habitat. However, this species now has a patchy regional distribution across major conservation reserves (e.g., Murray-Sunset National Park) and has become locally extinct in some smaller reserves due in part to a large fire incinerating most of a reserve (i.e., Billiat Reserve) (Figure 4) (Brown et al., 2009; Verdon et al., 2020).
Conversely, for an early successional species, lack of fire and consequent successional change may make habitats less suitable and limit movements and population connectivity. The knob-tailed gecko (Nephrurus stellatus), an early successional species in mallee vegetation on the Eyre Peninsula, increases in population density for 10–15 years after fire, then declines dramatically by >30 years post-fire (Driscoll et al., 2012). Change in habitat suitability with post-fire succession results in decreased dispersal of individuals and a decrease in genetic diversity of local populations (Smith et al., 2016).
Habitat loss and fragmentation in the Murray Mallee region has also affected fire regimes. In cleared farmland landscapes, mallee vegetation typically persists as small disturbed fragments and linear networks along road reserves (Figure 8A). As is also the case in fragmented mallee systems in western Australia (Parsons and Gosper, 2011), small fragments now rarely experience fire due to reduced ignition, greater suppression efforts and reduced connectivity of fuels. One consequence is that with increasing post-fire age of mallee eucalypts (e.g., >80 years), stem sizes are large and tree-hollow formation has progressed to provide an important resource for hollow-dependent species (e.g., parrots, bats). Should fire occur, these isolated patches (and even larger ones like Billiat Reserve, Figure 4) are now unlikely to follow the same patterns of plant and animal succession as observed in larger tracts of vegetation due to isolation limiting colonization (Nimmo et al., 2019). Their post-fire faunal communities are likely to reflect the species present in surrounding modified environments (Simms et al., 2019), and result in more generalist faunal communities.
Rainfall and Drought
Rainfall interacts with fire to shape ecosystem structure and ecological processes in several ways. First, increased rainfall stimulates germination and growth of plants, whereas drought suppresses such activity. Thus, for a particular post-fire successional stage, above-average rains may result in a greater diversity of plants or disproportionate cover of species relative to a drier situation. Second, increased rainfall stimulates primary productivity, expressed in greater plant biomass and structural complexity, and increased flowering, fruiting and seeding, thus altering food resources and structural habitats for fauna. Third, changes in primary productivity and plant growth influence the likelihood of future fire, as discussed previously in Patterns and Characteristics of Fire in Mallee Ecosystems.
Repeated sampling of fauna and vegetation structure at a set of sites in the Murray Mallee region before and after a period of above-average rainfall (the Mallee Hawkeye Project; Avitabile, 2014) provided insight into the interacting effects of fire and rainfall on biota (Connell et al., 2021). Repeat surveys were conducted: (a) in 2006–2008, during the Millennium Drought; (b) in 2011–2012 during a subsequent major La Niña rainfall event; and (c) in 2014–2015, in dry conditions following the La Niña event. The Millennium Drought was one of the most severe, extended droughts on record for the Murray Darling Basin, with below-average annual rainfall from 2001 to 2009 (Leblanc et al., 2012). The following La Niña event had exceptional, drought-breaking rains which comprised the highest mean annual rainfall recorded for the Basin (Leblanc et al., 2012). The post-La Niña rainfall period had below-average rainfall. The Mallee Hawkeye Project shed new light on the impacts of drought and rainfall upon key components of the ecosystem: vegetation, birds, mammals and reptiles, outlined below.
Following above-average rainfall, there was no overall, region-wide increase in the cover of Triodia, unlike the post-rainfall irruption of annual grasses such as Austrostipa spp. (Noble and Vines, 1993). However, there was a decrease in the strength of the relationship of Triodia cover with time since fire. After years of average/below-average rainfall, time-since-fire had a strong effect on T. scariosa (Haslem et al., 2011). After 2 years of above-average rainfall, this strong effect was no longer observed (Avitabile, 2014). Further, there was biogeographic variation in the response of Triodia to time since fire: more arid sites showed a stronger response than sites with higher annual rainfall (Avitabile, 2014). This suggests that above-average rainfall can release time since fire related constraints on Triodia cover in the wetter parts of its range, but not in the more arid parts.
High rainfall had a massive effect on bird communities, more than doubling the incidence of many species and species richness at sites post-rainfall. For several years following high rainfall, there were changes to post-fire responses of some, but not all, species compared with drought conditions (Connell et al., 2021). Some, like the yellow-plumed honeyeater in Triodia Mallee, exhibited peaks of occurrence across a greater range of post-fire ages. Many other species exhibited a heightened preference for mid to older post-fire vegetation over younger age-classes following wetter conditions. Connell et al. (2021) suggested the longevity of rainfall effects on ecosystems might itself have a successional basis, whereby resources boosted by rainfall persist for longer periods in older fire-age classes than in recently burned vegetation.
Capture rates of rodents (house mouse Mus musculus, Bolam’s mouse Pseudomys bolami) and the western pygmy possum (Cercartetus concinnus) are associated with higher antecedent rainfall (Kelly et al., 2013). Seed set by grasses following pulses of heavy rainfall provide short-term food resources that may result in population increases of granivorous small mammals and birds (Kelly et al., 2013; Connell et al., 2021).
Overall, reptile responses to time since fire did not change appreciably between low and high rainfall periods. For example, capture rates of the southern legless lizard were highest in mid-successional vegetation (bell-shaped response) during both survey periods; and for the mallee dragon (Ctenophorus fordi) in early successional vegetation, irrespective of high or low rainfall period (Avitabile, 2014).
The multi-taxa, long-term data set amassed during the Mallee Hawkeye Project highlights how important the climatic context is on the effects of fire on biota. It suggests species may be more vulnerable to wildfire or planned fire under certain climatic conditions than others—for example, planned fires conducted in preferred post-fire age classes (e.g., older vegetation) during periods when a species’ population is low (e.g., following prolonged drought) may pose high risk (Clarke, 2008). Further monitoring is needed to understand the longevity of the beneficial effects of high rainfall events to biota.
Herbivory
Herbivory interacts with fire to influence the distribution and abundance of biota, primarily through differential levels of herbivory in post-fire successional stages. Here, we focus on vertebrate mammalian herbivores, but note that the role of herbivory by invertebrates (e.g., termites, psyllids, ants, locusts and grasshoppers) is likely to be profound – though poorly understood and documented. Mammalian herbivores include native species (e.g., western grey kangaroo Macropus fuliginosus, red kangaroo M. rufus) and introduced exotic species (e.g., goat Capra hircus, European rabbit, brown hare Lepus capensis). Some native herbivores are now regionally extinct (e.g., stick-nest rat Leporillus conditor) whereas others (e.g., western grey kangaroo) are probably more abundant than historically, due to loss of top-order predators and provision of permanent water for stock.
Herbivory can threaten a range of rare plant species (Sandell, 2006; Cheal, 2009), especially palatable species, as it affects their rates of survival, growth, reproduction and recruitment. It can shift community composition toward dominance by unpalatable species (Keith and Tozer, 2012). The threat is greatest in early post-fire succession when ephemeral species establish and otherwise-unpalatable plants are at their most vulnerable (Cohn and Bradstock, 2000).
The interaction of frequent fire and herbivory results in an even greater impact than just fire alone (Driscoll et al., 2012; Keith, 2012). The effect of herbivory on vegetation recovery post-fire has broader ecological implications when it affects the structural habitat components or food sources of other animals (Figure 8C). For example, grazing pressure on the regeneration of a foundation species such as Triodia (Cohn and Bradstock, 2000) could have detrimental effects on Triodia-specialist vertebrates if regeneration is inhibited.
The interaction between herbivory and fire can also vary as a function of abiotic conditions (particularly rainfall). After monitoring herbivore exclusion and control plots for more than a decade following fire and a flood at Nagaella Station in NSW, Florentine et al. (2015) concluded that flooding and herbivory combined led to greater long-term modification of vegetation composition and structure than did fire alone. In an experimental study (Giljohann et al., 2017b) that spanned the ‘Millennium Drought’ and subsequent record-breaking rainfall, Triodia recruitment and post-fire regeneration were markedly reduced by mammalian herbivory when below-average rainfall followed prescribed fires. In contrast, mammalian herbivory following wildfire had a minor effect on recruitment and post-fire regeneration during the same period (Giljohann et al., 2017b). Herbivory may be more intense in smaller burnt areas where herbivores are attracted to congregate at higher densities and benefit from the juxtaposition of shelter and foraging areas in unburnt and recently burnt areas, respectively.
There is potential for the interaction between herbivory and fire to have major, long-term impacts on the structure, composition and distribution of mallee communities. However, the unpredictable and idiosyncratic nature of major rainfall events and fires, coupled with the slow recovery rates of vegetation highlight the value of the opportunistic establishment of herbivore exclusion and control plots that can be monitored over decades following such events. Only by committing to long-term monitoring will a deeper understanding of these crucial, but dynamic, interactions be gained.
Predation
The role of top-order predators, notably the dingo (Canis dingo) as a keystone species that influences ecosystem structure and function in arid Australia, is increasingly recognized (Letnic et al., 2009, 2012). Two main mechanisms are proposed. First, by reducing the activity and abundance of feral and native herbivores (e.g., feral goats, large macropod species), dingoes contribute to a cascade of positive effects for plant and animal species at lower trophic levels. Second, by regulating meso-predator abundance and behavior there can be benefits for populations of prey species, including threatened small mammals (Ritchie et al., 2012; Hunter et al., 2018).
In semi-arid mallee ecosystems, the mammalian predator community has changed markedly since European colonization (Bennett et al., 1989). The dingo, formerly widespread and common, is persecuted by livestock graziers via trapping and poisoning, and no longer occurs in many areas in the Murray Mallee. Dingoes persist in the Big Desert-Wyperfeld system, are occasionally seen in the Little Desert, but are rare elsewhere. Native quolls (Dasyurus spp.) have been eliminated. Two exotic and invasive ‘meso-predators,’ the red fox (Figure 8D) and cat, are widespread. There is some evidence that foxes avoid, or are suppressed by, dingoes in the Big Desert (Geary et al., 2018): fewer foxes were recorded in areas with more signs of dingoes. Such an outcome may benefit native prey of foxes, including threatened or near-threatened species (e.g., Mitchell’s hopping mouse, malleefowl), but this requires further examination.
Fire can potentially interact with predation (Geary et al., 2020). Fire changes the structure of habitats by removing vegetative cover and refuges, making prey species more exposed to predation. A more-open structure in recently burned areas may result in both a localized increase in predator activity and greater hunting success, both of which can result in increased mortality of prey species and limit post-fire population recovery (Doherty et al., 2015; Hradsky, 2020). This is likely to occur in mallee ecosystems, but further work is required to quantify variation in predation in relation to post-fire succession.
Fire and Interacting Drivers in a Changing Climate
In an era of a changing global climate, it is essential to consider how semi-arid mallee ecosystems will respond to future perturbations. Given predicted climate changes for the region (Table 2), we consider two pressing questions: (a) how might fire regimes and other interacting drivers be affected by climate change? and (b) which elements of the biotic environment have the capacity to respond to changes in these drivers and which do not?
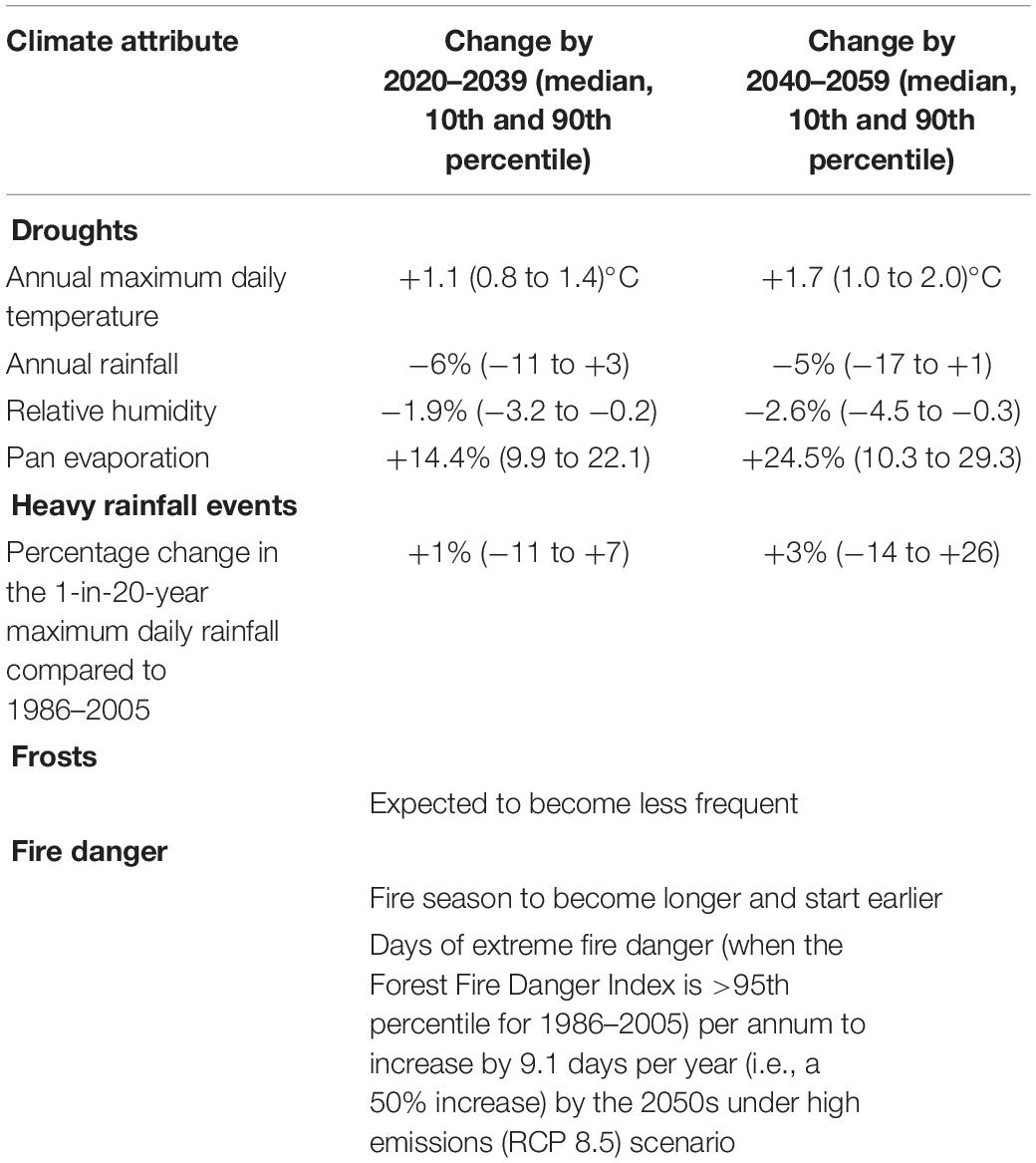
Table 2. Climate projections for Mildura under a medium (RCP4.5) emissions reduction scenario (Clarke et al., 2019).
The most recent climate forecasts for the Murray Mallee region suggest the next 10 to 30 years will be characterized by higher temperatures, reduced humidity, higher evaporation rates, longer and more severe droughts, greater variability in the quantity and timing of major rainfall events, and fewer frosts (Table 2). The northern parts of the region are forecast to experience a climate with more similarities to the arid zone than the semi-arid (Clarke et al., 2019).
At a regional scale, these climatic changes are forecast to result in a 50% increase in the number of days of extreme fire danger, and a fire season that starts earlier and is longer in duration. Thus, potential changes to fire regimes, along with climatic changes in water availability, are likely to have extensive ecological ramifications. Other drivers (e.g., topography, soils, biogeographic history) will constrain the ability of the biota to cope with these changes.
Whether climatic changes in mallee ecosystems cause fire activity to increase or decrease will depend on the interplay between four ‘switches’ (sensu Bradstock, 2010), including biomass growth, availability of fuel, fire weather, and ignition sources. On the one hand, vegetation growth and fuel accumulation may decline under reduced annual rainfall – and considered in isolation might be expected to reduce fire activity. On the other hand, higher temperatures may promote greater availability of dry fuel and increased fire activity (O’Donnell et al., 2011a). The occurrence of dry lightning is suggested to increase in Mediterranean climates, like mallee ecosystems, under climate change (Mariani et al., 2018; Dowdy, 2020). The relationships between these switches and their outcomes require testing but we can use ecological knowledge to explore alternative scenarios of potential fire regime change.
First, an increase in fire frequency in the Murray Mallee region could have a negative impact on many species, given that resources required by some are still developing more than a century after a fire (Haslem et al., 2011) and that a significant proportion of faunal species do not reach their peak abundance until 30 or more years post-fire. Second, if fires become more extensive, severe and even less patchy, it is likely to affect less-mobile species with limited capacity to relocate to unburnt patches, or recolonize from outside the fire perimeter, especially where mallee vegetation has been fragmented by agriculture. Third, under a drier climate scenario, the rate at which vegetation recovers after fire (e.g., years to first flowering or seeding) will likely be slowed, contributing to what has been termed an ‘interval squeeze’ (Keeley et al., 2005; Enright et al., 2015). Thus, insufficient seeds are produced between fires to replace individuals killed by the previous fire, leading to local extinction. Alternatively, if climate change results in a scenario of reduced fire activity, this may be easier to manage; strategic use of planned fire could be used to trigger germination of plants that require more fire, or to create habitat for animals that prefer early or mid-successional habitats.
A changing climate will also affect diverse processes that interact with fire in shaping mallee ecosystems. Decreased moisture availability could profoundly affect processes by which carbohydrates become available to heterotrophs. For example, if eucalypt flowering becomes less frequent and less seasonal under a drying scenario, this could affect the survivorship and reproductive success of both resident and nomadic nectarivores (Keast, 1968). Similarly, climate-driven declines of psyllids, which produce carbohydrate-rich lerps eaten by many invertebrates and vertebrates (Paton, 1980), would likely diminish the capacity of these heterotrophs to recover after fire.
Desiccation and degradation of cryptogamic soil crusts under a drying climate will likely result in increased wind and water erosion and accompanying loss or redistribution of soil nutrients. Dust storms, previously triggered by overgrazing and clearing of native vegetation (Broome et al., 2020) may become more common with longer dry periods under climate change (Speer, 2013). The regional loss of nutrients is likely to take centuries to recover, but the potential effects on post-fire recovery of the biota are unknown.
A further issue concerns the capacity of biota to respond to extreme events, both droughts and wet years, which have long-lasting impacts. While the abundance of some bird species more than doubled after wet years, populations of some endangered birds did not recover from drought even after high rainfall (Connell et al., 2021). Species able to rapidly capitalize on unpredictable flushes of resources may benefit from an increased likelihood of major rainfall events. Whether such events will be sufficiently frequent for species to recover and avoid local extinction during longer and more severe droughts, is unclear. The subdued topography of mallee ecosystems and their nutrient-poor soils appear to offer little in the form of permanent drought refuges. Nevertheless, there remains much to learn about how variation in habitat suitability is associated with topography (Selwood et al., 2019; Verdon et al., 2019). Much will depend on a species’ ability to move between resources (Nimmo et al., 2019), and whether habitat connectivity enables such movement.
Where to From Here?
Mallee ecosystems have extraordinary value for the conservation of biodiversity in Australia: they support a diverse and distinctive flora and fauna, including many species of threatened conservation status, some critically endangered. Although extensively cleared for agriculture, large areas remain in conservation reserves allowing opportunities for pro-active conservation and management.
Conservation Priorities in a Flammable Ecosystem
Primary challenges for conservation in mallee ecosystems in relation to fire are: (a) to determine what ‘desirable’ fire mosaics, with sufficient older seral stages to sustain the biota in the long-term, look like at local and regional scales and (b) how best to achieve these through a combination of planned fire, wildfire prevention and suppression, and other active management.
A key goal in fire management is to protect and foster the development of older post-fire age classes in all regional blocks of mallee vegetation, while avoiding a single wildfire burning an entire reserve. The predicted worsening of fire weather and climatic conditions (Table 2) suggest that natural ignitions and uncontrolled wildfire will deliver sufficient younger post-fire age classes to accommodate species with a preference for early successional stages. Protecting key habitats will be enhanced by a capacity to link knowledge of species distributions in relation to post-fire succession and environmental variation with ‘real world,’ spatially explicit simulations of alternative fire management strategies. This will assist fire managers to evaluate the risks and benefits of different combinations of fire management actions. Monitoring of fuels and vegetation recovery, and a willingness to adjust burning prescriptions in an adaptive manner, will be crucial.
Management interventions that reduce the detrimental impacts of drivers that interact with fire will assist plant and animal populations to thrive post-fire and recover in good climatic seasons after disturbance. These include sustained efforts to reduce or eliminate feral herbivores and predators, and the resources on which they rely (e.g., artificial watering points; James et al., 1999). We must also anticipate climatically induced shifts in the distribution of foundation species (e.g., Triodia), and potential invasive species (e.g., buffel grass Cenchrus ciliaris) (Read et al., 2020) that would cause major changes to fire regimes. Both affect the connectivity of ground fuels (Gibson et al., 2014). Such changes could result in irreversible losses of biodiversity on a regional scale.
Protecting and maintaining existing native vegetation is a high priority, particularly large contiguous blocks (on both public and private lands) of sufficient scale to enable internal recolonization following disturbance events, such as wildfire. Enhancing the effectiveness of crucial corridors for dispersal, including mesic woodlands along major river systems and north-south connections along climatic gradients, will support resilience to a changing climate. Likewise, increasing the contiguity between remaining areas of native vegetation, particularly conservation reserves separated by croplands, is an ambitious goal, but such broad-scale revegetation is being undertaken successfully (Koch, 2015; Berkinshaw et al., 2016) and must be commensurate to the magnitude of the challenge facing the extraordinary biota of this region.
Reintroduction of species historically present offers exciting opportunities for the conservation of threatened species and the restoration of ecosystem function (Hayward et al., 2016). Several such mammal species (e.g., brush-tailed bettong Bettongia penicillata, burrowing bettong B. lesueur, greater bilby Macrotis lagotis) function as ecosystem engineers and as such may play a role in moderating fire regimes. Current trials of reintroductions of these and other species in several locations (e.g., Scotia Sanctuary, Mallee Cliffs NP) could pave the way for wider efforts, although control of introduced predators is a necessary and formidable challenge.
Conservation of mallee ecosystems will also require ongoing vigilance and response to changes in human land-use. Such changes in coming decades may include changes in agricultural practices and products in response to climate change; increased human access, roading and recreational use within mallee ecosystems; the introduction and spread of new invasive weeds; increased isolation and reduced capacity for dispersal by species; and the potential for new exploitative uses of mallee vegetation to provide products for human society.
Research Priorities
Despite the extensive body of research summarized in this review, two crucial questions still need place-based solutions if the insights gained are to be translated into on-ground actions that will enhance the conservation of mallee biodiversity.
1. What fire regimes should be fostered, and at what scale, to sustain biodiversity in mallee ecosystems?
While scientists are applauded for discovering principles or insights with global applicability, land managers are judged by whether their interventions in particular locations and times lead to desired outcomes. The latter’s place-based focus has the potential to align with the deep appreciation of ‘place’ inherent in Indigenous peoples’ understanding and application of fire. For example, insights into the use of fire in semi-arid environments (including mallee vegetation) by Indigenous Ngadju people in southern Western Australia (Prober et al., 2016) highlight a place-based approach to where, when and how fire is applied (including some locations where fire is excluded). Use of fire is tailored to actual places, takes into account the idiosyncratic effects of drought/wet cycles due to unique topography, soils, waterbodies, vegetation and rock formations; and recognizes the need to protect important resources. Effective partnerships and collaboration with Indigenous people in the management of mallee ecosystems offer new opportunities, including fostering Indigenous knowledge of fire and its cultural benefits. The challenge is how to translate general principles about the fire ecology of a region into place-based solutions that effectively conserve ecological assets and cultural values.
Addressing this over-arching question requires still further attention to outstanding ecological questions relating to how mallee ecosystems function (Table 3, questions 1–5). The better key ecological drivers and how they interact (Table 3, questions 6–15) are understood, the better equipped multiple stakeholders will be to explore likely future scenarios and the potential impacts of interventions (Table 4), and so make informed choices between specific place-based management actions.
2. When and where should conservation actions be undertaken to achieve desirable fire regimes and avoid undesirable fire regimes?
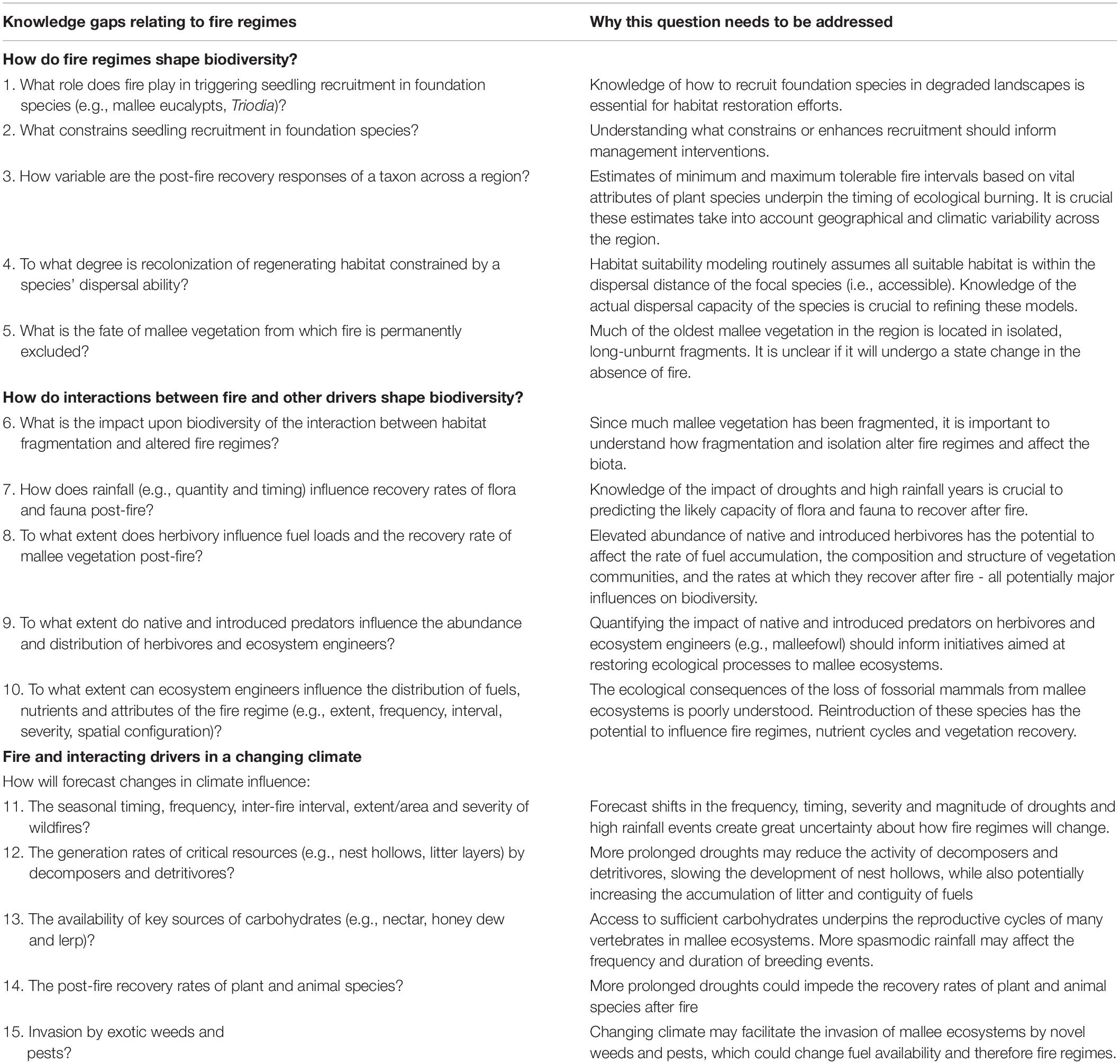
Table 3. Knowledge gaps and research priorities relating to how fundamental components of mallee ecosystems operate.
Most insights into the role of fire in mallee ecosystems have been gained from correlational studies (e.g., sampling designs based on space-for-time substitution) or long-term monitoring of ‘treatment’ sites without matching controls, rather than through controlled experimental manipulations. Nevertheless, management interventions – such as wildfire suppression, planned burning, weed and pest control – are regularly applied, often in the absence of rigorous monitoring of outcomes. We propose that this second over-arching question can be addressed by spatially explicit scenario modeling, combined with experimental and opportunistic application of management interventions (Table 4) that are routinely monitored to determine their ecological outcomes. Adopting a genuinely experimental and adaptive approach to management interventions could produce deeper ecological insights into how mallee ecosystems function, and also more relevant, spatially explicit solutions to the challenges these systems face.
Author Contributions
MC and AB led the Mallee Hawkeye Project, they assembled and edited the manuscript with significant assistance from LK, KG, and AH. All authors contributed to the conceptual development of the manuscript and provided review and edits across the manuscript.
Conflict of Interest
The authors declare that the research was conducted in the absence of any commercial or financial relationships that could be construed as a potential conflict of interest.
The reviewer, CG, declared a past co-authorship with two of the authors, SW and MWh, to the handling editor.
Acknowledgments
We acknowledge the traditional owners of the lands where this research was conducted. We pay our respects to their elders, past, present and emerging. This synthesis arose from a workshop at La Trobe University held as part of the Mallee-Hawkeye Project, funded by the then Department of Environment and Primary Industries, Victoria, Australia. We extend our gratitude to the staff from agencies, non-government organizations and institutions who have been so generous in sharing their time, knowledge, expertise and support for the many research and management projects that underpin this synthesis; in particular Ross Bradstock, Jemima Connell, Ray Dayman, Malcom Gill, Victor Hurley, Jose Lahoz-Monfort, Peter Sandell, and Simon Verdon. Many thanks to Clare Kelly for designing Figures 9–11.
References
Andersen, A. N. (1982). “Seed removal by ants in the mallee of northwestern Victoria,” in Ant-Plant Interactions in Australia, ed. R. Buckley (Netherlands: Springer), 31–43. doi: 10.1007/978-94-009-7994-9_5
Andersen, A. N. (1983). Species diversity and temporal distribution of ants in the semi-arid mallee region of north-western Victoria. Aust. J. Ecol. 8, 127–137. doi: 10.1111/j.1442-9993.1983.tb01600.x
Andersen, A. N., and Yen, A. L. (1985). Immediate effects of fire on ants in the semi-arid mallee region of north-western Victoria. Aust. J. Ecol. 10, 25–30. doi: 10.1111/j.1442-9993.1985.tb00860.x
Archibald, S., Lehmann, C. E. R., Gómez-Dans, J. L., and Bradstock, R. A. (2013). Defining pyromes and global syndromes of fire regimes. Proc. Natl. Acad. Sci. U.S.A. 110, 6442–6447. doi: 10.1073/pnas.1211466110
Avitabile, S. (2010). The Effect of Fire Regimes on the Biodiversity of Selected Invertebrates in the Murray Mallee Habitats of South-Eastern Australia. Ph.D. thesis. Melbourne, VIC: La Trobe University.
Avitabile, S. (2014). Mallee Hawkeye Project: Final Report 2011-2014. Melbourne, VIC: Department of Environment and Primary Industries.
Avitabile, S. C., Callister, K. E., Kelly, L. T., Haslem, A., Fraser, L., Nimmo, D. G., et al. (2013). Systematic fire mapping is critical for fire ecology, planning and management: a case study in the semi-arid Murray Mallee, south-eastern Australia. Landsc. Urban Plan. 117, 81–91. doi: 10.1016/j.landurbplan.2013.04.017
Avitabile, S. C., Nimmo, D. G., Bennett, A. F., and Clarke, M. F. (2015). Termites are resistant to the effects of fire at multiple spatial scales. PLoS One 10:e0140114. doi: 10.1371/journal.pone.0140114
Barengi Gadjin Land Council (2017). Country Plan: Growing What is Good. Voices of the Wotjabaluk Nations. Horsham VIC: Barengi Gadjin Land Council.
Bell, K. J., Doherty, T. S., and Driscoll, D. A. (2021). Predators, prey or temperature? Mechanisms driving niche use of a foundation plant species by specialist lizards. Proc. R. Soc. B Biol. Sci. 288:20202633. doi: 10.1098/rspb.2020.2633
Bennett, A. F., Lumsden, L. F., and Menkhorst, P. W. (1989). “Mammals of the mallee region of southeastern Australia,” in Mediterranean Landscapes in Australia: Mallee Ecosystems and their Management, eds J. C. Noble and R. A. Bradstock (Melbourne, VIC: CSIRO Publishing), 191–220.
Bennett, A. F., Lumsden, L. F., and Menkhorst, P. W. (2006). Mammals of the Mallee region, Victoria: past, present and future. Proc. R. Soc. Vic. 118, 259–280.
Benshemesh, J. (1990). “Management of Malleefowl with regard to fire,” in The Mallee Lands: A Conservation Perspective, eds J. C. Noble, P. J. Joss, and G. K. Jones (Melbourne, VIC: CSIRO Publishing), 206–211.
Berkinshaw, T. D., Durant, M., and Koch, P. J. (2016). WildEyre Conservation Action Planning Report June 2015. Report to the WildEyre Working Group. Brisbane: Greening Australia.
Berry, L. E., Lindenmayer, D. B., and Driscoll, D. A. (2015). Large unburnt areas, not small unburnt patches, are needed to conserve avian diversity in fire-prone landscapes. J. Appl. Ecol. 52, 486–495. doi: 10.1111/1365-2664.12387
Black, A., Dolman, G., Wilson, C. A., Campbell, C. D., Pedler, L., and Joseph, L. (2020). A taxonomic revision of the Striated Grasswren Amytornis striatus complex (Aves: Maluridae) after analysis of phylogenetic and phenotypic data. Emu 120, 191–200. doi: 10.1080/01584197.2020.1776622
Bliege Bird, R., and Nimmo, D. (2018). Restore the lost ecological functions of people. Nature Ecol. Evol. 2, 1050–1052. doi: 10.1038/s41559-018-0576-5
Bowler, J. M., Johnston, H., Olley, J. M., Prescott, J. R., Roberts, R. G., Shawcross, W., et al. (2003). New ages for human occupation and climate change at Lake Mungo. Australia. Nature 421, 837–840. doi: 10.1038/nature01383
Bowler, J. M., and Magee, J. W. (1978). Geomorphology of the Mallee Region in semi arid northern Victoria and western New South Wales. Proc. R. Soc. Vic. 90, 5–25.
Bradstock, R. A. (1989a). “Dynamics of a perennial understorey,” in Mediterranean Landscapes in Australia: Mallee Ecosystems and their Management, eds J. C. Noble and R. A. Bradstock (Victoria, VIC: CSIRO Publishing), 141–154.
Bradstock, R. A. (1989b). “Relationship between fire regimes, plant species and fuels,” in The Mallee Lands – a Conservation Perspective, eds J. C. Noble, P. J. Joss, and G. K. Jones (East Melbourne, VIC: CSIRO), 218–223.
Bradstock, R. A. (2010). A biogeographic model of fire regimes in Australia: current and future implications. Glob. Ecol. Biogeog. 19, 145–158. doi: 10.1111/j.1466-8238.2009.00512.x
Bradstock, R. A., Bedward, M., and Cohn, J. S. (2006). The modelled effects of differing fire management strategies on the conifer Callitris verrucosa within semi-arid mallee vegetation in Australia. J. Appl. Ecol. 43, 281–292. doi: 10.1111/j.1365-2664.2006.01142.x
Bradstock, R. A., Bedward, M., Gill, A. M., and Cohn, J. S. (2005). Which mosaic? A landscape ecological approach for evaluating interactions between fire regimes, habitat and animals. Wildl. Res. 32, 409–423. doi: 10.1071/wr02114
Bradstock, R. A., and Cohn, J. S. (2002). “Fire regimes and biodiversity in semi-arid mallee ecosystems,” in Flammable Australia: the Fire Regimes and Biodiversity of a Continent, eds R. A. Bradstock, J. E. Williams, and A. M. Gill (Cambridge: Cambridge University Press), 239–258.
Bradstock, R. A., and Gill, A. M. (1993). Fire in semi-arid, mallee shrublands: size of flames from discrete fuel arrays and their role in the spread of fire. Int. J. Wildl. Fire 3, 3–12. doi: 10.1071/wf9930003
Broome, R., Fahey, C., Gaynor, A., and Holmes, K. (2020). Mallee Country: Land, People, History. Clayton: Monash University Publishing.
Brown, S., Clarke, M., and Clarke, R. (2009). Fire is a key element in the landscape-scale habitat requirements and global population status of a threatened bird: the mallee emu-wren (Stipiturus mallee). Biol. Conserv. 142, 432–445. doi: 10.1016/j.biocon.2008.11.005
Brown, S. M., Harrisson, K. A., Clarke, R. H., Bennett, A. F., and Sunnucks, P. (2013). Limited population structure, genetic drift and bottlenecks characterise an endangered bird species in a dynamic, fire-prone ecosystem. PLoS One 8:e59732. doi: 10.1371/journal.pone.0059732
Burbidge, A. H., Joseph, L., Toon, A., White, L. C., McGuire, A., and Austin, J. J. (2017). A case for realigning species limits in the southern Australian whipbirds long recognised as the Western Whipbird (Psophodes nigrogularis). Emu 117, 254–263. doi: 10.1080/01584197.2017.1313685
Burch, J. (2020). Parish plans as a source of evidence of Aboriginal land use in the Mallee back country. Provenance J. Public Rec. Office Vic. 18, 9–21.
Callister, K. E., Griffioen, P. A., Avitabile, S. C., Haslem, A., Kelly, L. T., Nimmo, D. G., et al. (2016). Historical maps from modern images: using remote sensing to model and map century-long vegetation change in a fire-prone region. PLoS One 11:e0150808. doi: 10.1371/journal.pone.0150808
Cheal, D. (2009). Twenty years of grazing reduction in semi-arid woodlands. Pacific Cons. Biol. 15, 268–277. doi: 10.1071/pc090268
Cheal, D. (2010). Growth Stages and Tolerable Fire Intervals for Victoria’s Native Vegetation Data Sets. Fire and Adaptive Management Report No. 84. East Melbourne, VIC: Department of Sustainability and Environment.
Cheal, D. C., and Parkes, D. M. (1989). “Mallee vegetation in Victoria,” in Mediterranean Landscapes in Australia: Mallee Ecosystems and their Management, eds J. C. Noble and R. A. Bradstock (Victoria, VIC: CSIRO Publishing), 125–140.
Cheal, P., Day, J. C., and Meredith, C. (1979). Fire in the National Parks of North-West Victoria. Melbourne, VIC: National Parks Service.
Chuvieco, E., Giglio, L., and Justice, C. (2008). Global characterization of fire activity: towards defining fire regimes from Earth observation data. Glob. Change Biol. 14, 1488–1502. doi: 10.1111/j.1365-2486.2008.01585.x
Clarke, J. M., Grose, M., Thatcher, M., Round, V., and Heady, C. (2019). Mallee Climate Projections 2019. Melbourne, VIC: CSIRO.
Clarke, M. F. (2008). Catering for the needs of fauna in fire management: science or just wishful thinking? Wildl. Res. 35, 385–394. doi: 10.1071/wr07137
Clarke, M. F., Avitabile, S. C., Brown, L., Callister, K. E., Haslem, A., Holland, G. J., et al. (2010). Ageing mallee eucalypt vegetation after fire: insights for successional trajectories in semi-arid mallee ecosystems. Aust. J. Bot. 58, 363–372. doi: 10.1071/bt10051
Clarke, P. A. (2009). “Aboriginal culture and the Riverine environment,” in The Natural History of the Riverland and Murraylands, ed. J. T. Jennings (Adelaide: Royal Society of South Australia), 142–161.
Clarke, P. J., Lawes, M. J., Murphy, B. P., Russell-Smith, J., Nano, C. E. M., Bradstock, R., et al. (2015). A synthesis of postfire recovery traits of woody plants in Australian ecosystems. Sci. Total Environ. 534, 31–42. doi: 10.1016/j.scitotenv.2015.04.002
Cogger, H. G. (1989). “Herpetofauna,” in Mediterranean Landscapes in Australia. Mallee Ecosystems and their Management, eds J. C. Noble and R. A. Bradstock (Melbourne, VIC: CSIRO Publishing), 250–265.
Cohn, J. S., and Bradstock, R. A. (2000). Factors affecting post-fire seedling establishment of selected mallee understorey species. Aust. J. Bot. 48, 59–70. doi: 10.1071/bt98031
Cohn, J. S., Bradstock, R. A., and Burke, S. (2002). Effects of time-since-fire, topography and resprouting eucalypts on ephemeral understorey species composition, in semi-arid Mallee communities in western NSW. Cunninghamia 7, 579–600.
Connell, J., Hall, M., Nimmo, D., Watson, S. J., and Clarke, M. F. (2021). Fire, drought and flooding rains: the effect of climatic extremes on bird species’ responses to time since fire. Divers. Distrib. (in press).
Cruz, M. G., McCaw, W. L., Anderson, W. R., and Gould, J. S. (2013). Fire behaviour modelling in semi-arid mallee-heath shrublands of southern Australia. Environ. Modell. Softw. 40, 21–34. doi: 10.1016/j.envsoft.2012.07.003
Cullen, L. E., and Grierson, P. F. (2009). Multi-decadal scale variability in autumn-winter rainfall in south-western Australia since 1655 AD as reconstructed from tree rings of Callitris columellaris. Clim. Dyn. 33, 433–444. doi: 10.1007/s00382-008-0457-8
Davis, H., Richie, E. G., Avitabile, S., Doherty, T., and Nimmo, D. G. (2018). Testing the assumptions of the pyrodiversity begets biodiversity hypothesis for termites in semi-arid Australia. R. Soc. Open Sci. 5:1725055. doi: 10.1098/rsos.172055
Department for Environment and Water (2016). Last Bushfire and Prescribed Burn Boundaries. Available online at: https://data.sa.gov.au/data/dataset/last-fire (accessed on December 2020).
Department of Environment Land Water and Planning (2020). Fire History Last Burnt. Available online at: http://services.land.vic.gov.au/catalogue/metadata?anzlicId=ANZVI0803004774&publicId=guest&extractionProviderId=1#tab1 (accessed on December 2020)
Department of Sustainability Environment Water Population and Communities (2013). Interim Biogeographic Regionalisation for Australia (IBRA), Version 7.
Department of the Environment (2018). Major Vegetation Groups. National Vegetation Information System, Version 6.
Dodson, J. (1982). “Current status of mallee ecosystems,” in Threats to Mallee in New South Wales, ed. J. A. Mabutt (Sydney, NSW: Department of Environment and Planning), 17–33.
Doherty, T. S., Dickman, C. R., Nimmo, D. G., and Ritchie, E. G. (2015). Multiple threats, or multiplying the threats? Interactions between invasive predators and other ecological disturbances. Biol. Cons. 190, 60–68. doi: 10.1016/j.biocon.2015.05.013
Dowdy, A. J. (2020). Climatology of thunderstorms, convective rainfall and dry lightning environments in Australia. Clim. Dyn. 54, 3041–3052. doi: 10.1007/s00382-020-05167-9
Driscoll, D. A., Armenteras, D., Bennett, A. F., Brotons, L., Clarke, M. F., Doherty, T. S., et al. (2021). How fire interacts with habitat loss and fragmentation. Biol. Rev. 96, 976–998.
Driscoll, D. A., Smith, A. L., Blight, S., and Maindonald, J. (2012). Reptile responses to fire and the risk of post-disturbance sampling bias. Biodiv. Cons. 21, 1607–1625. doi: 10.1007/s10531-012-0267-5
Driscoll, D. A., Smith, A. L., Blight, S., and Sellar, I. (2020). Interactions among body size, trophic level and dispersal traits predict beetle detectability and occurrence responses to fire. Ecol. Entom. 45, 300–310. doi: 10.1111/een.12798
Driscoll, D. A., and Weir, T. (2005). Beetle responses to habitat fragmentation depend on ecological traits, remnant condition and shape. Conserv. Biol. 19, 182–194. doi: 10.1111/j.1523-1739.2005.00586.x
Eldridge, D. J., and James, A. I. (2009). Soil-disturbance by native animals plays a critical role in maintaining healthy Australian landscapes. Ecol. Manage. Rest. 4, 114–119.
Enright, N. J., Fontaine, J. B., Bowman, D. M. J. S., Bradstock, R. A., and Williams, R. J. (2015). Interval squeeze: altered fire regimes and demographic responses interact to threaten woody species persistence as climate changes. Front. Ecol. Environ. 13:265–272. doi: 10.1890/140231
Farnsworth, L. M., Nimmo, D. G., Kelly, L. T., Bennett, A. F., and Clarke, M. F. (2014). Does pyrodiversity beget alpha, beta or gamma diversity? A case study using reptiles from semi-arid Australia. Divers. Distrib. 20, 663–673. doi: 10.1111/ddi.12181
Florentine, S., Milberg, P., Di Stefano, J., Westbrooke, M., and Graz, P. (2015). Decade-long response of arid-land mallee vegetation to fire, flooding and grazing in south-eastern Australia. J. Arid Environ. 121, 7–14. doi: 10.1016/j.jaridenv.2015.05.006
Friend, G. R. (1993). Impact of fire on small vertebrates in mallee woodlands and heathlands of temperate Australia: a review. Biol. Cons. 65, 99–114. doi: 10.1016/0006-3207(93)90439-8
Friend, G. R., and Williams, M. R. (1996). Impact of fire on invertebrate communities in mallee-heath shrublands of southwestern Australia. Pac. Cons. Biol. 2, 244–267. doi: 10.1071/pc960244
Geary, W., Ritchie, E. G., Lawton, J. A., Healey, T. R., and Nimmo, D. G. (2018). Incorporating disturbance into trophic ecology: fire history shapes mesopredator suppression by an apex predator. J. Appl. Ecol. 55, 1594–1603. doi: 10.1111/1365-2664.13125
Geary, W. L., Doherty, T. S., Nimmo, D. G., Tulloch, A. I. T., and Ritchie, E. G. (2020). Predator responses to fire: a global systematic review and meta-analysis. J. Anim. Ecol. 89, 955–971. doi: 10.1111/1365-2656.13153
Geiser, F., Stawski, C., Wacker, C. B., and Nowack, J. (2017). Phoenix from the ashes: fire, torpor, and the evolution of mammalian endothermy. Front. Physiol. 8:842. doi: 10.3389/fphys.2017.00842
Gibson, R., Bradstock, R., Penman, T., and Driscoll, D. A. (2014). Changing dominance of key plant species across a Mediterranean climate region; implications for fuel types and future fire regimes. Plant Ecol. 215, 83–95. doi: 10.1007/s11258-013-0280-0
Gibson, R., Bradstock, R., Penman, T., Keith, D. A., and Driscoll, D. A. (2015). Climatic, vegetation and edaphic influences on the probability of fire across Mediterranean woodlands of south eastern Australia. J. Biogeog. 42, 1750–1760. doi: 10.1111/jbi.12547
Giljohann, K. M., Kelly, L. T., Connell, J., Clarke, M. F., Clarke, R. H., Regan, T. R., et al. (2017a). Assessing the sensitivity of biodiversity indices used to inform fire management. J. Appl. Ecol. 55, 461–471. doi: 10.1111/1365-2664.13006
Giljohann, K. M., McCarthy, M. A., Keith, D. A., Kelly, L. T., Tozer, M. G., and Regan, T. J. (2017b). Interactions between rainfall, fire and herbivory drive resprouter vital rates in a semi-arid ecosystem. J. Ecol. 105, 1562–1570. doi: 10.1111/1365-2745.12768
Gosper, C. R., Prober, S. M., and Yates, C. J. (2013). Estimating fire interval bounds using vital attributes: Implications of uncertainty and among-population variability. Ecol. Appl. 23, 924–935. doi: 10.1890/12-0621.1
Gosper, C. R., Yates, C. J., and Prober, S. M. (2012a). Changes in plant species and functional composition with time since fire in two mediterranean climate plant communities. J. Veg. Sci. 23, 1071–1081. doi: 10.1111/j.1654-1103.2012.01434.x
Gosper, C. R., Yates, C. J., Prober, S. M., and Parsons, B. C. (2012b). Contrasting changes in vegetation structure and diversity with time since fire in two Australian Mediterranean-climate plant communities. Aust. Ecol. 37, 164–174. doi: 10.1111/j.1442-9993.2011.02259.x
Gullan, P. J., Cranston, P. S., and Cook, L. G. (1997). The response of gall-inducing scale insects (Hemiptera: Eriococcidae: Apiomorpha Rubsaamen) to the fire history of mallee eucalypts in Danggali Conservation Park, South Australia. Trans. R. Soc. South Aust. 121, 137–146.
Haslem, A., Avitabile, S. C., Taylor, R. S., Kelly, L. T., Watson, S. J., Nimmo, D. G., et al. (2012). Time-since-fire and inter-fire interval influence hollow availability for fauna in a fire-prone system. Biol. Conserv. 152, 212–221. doi: 10.1016/j.biocon.2012.04.007
Haslem, A., Callister, K. E., Avitabile, S. C., Griffioen, P. A., Kelly, L. T., Nimmo, D. G., et al. (2010). A framework for mapping vegetation over broad spatial extents: a technique to aid land management across jurisdictional boundaries. Landscape Urban Plan. 97, 296–305. doi: 10.1016/j.landurbplan.2010.07.002
Haslem, A., Kelly, L. T., Nimmo, D. G., Watson, S. J., Kenny, S. A., Taylor, R. S., et al. (2011). Habitat or fuel? Implications of long-term, post-fire dynamics for the development of key resources for fauna and fire. J. Appl. Ecol. 48, 247–256. doi: 10.1111/j.1365-2664.2010.01906.x
Hayward, M. W., Ward-Fear, G., L’Hotellier, F., Herman, K., Kabat, A. P., and Gibbons, J. P. (2016). Could biodiversity loss have increased Australia’s bushfire threat? Anim. Cons. 19, 490–497. doi: 10.1111/acv.12269
He, T., Lamont, B. B., and Pausas, J. G. (2019). Fire as a key driver of Earth’s biodiversity. Biol. Rev. 94, 1983–2010. doi: 10.1111/brv.12544
Hill, K. D. (1989). “Mallee eucalypt communities: their classification and biogeography,” in Mediterranean Landscapes in Australia: Mallee Ecosystems and Their Management, eds J. C. Noble and R. A. Bradstock (Melbourne, VIC: CSIRO Publishing), 93–108.
Hradsky, B. A. (2020). Conserving Australia’s threatened native mammals in predator-invaded, fire-prone landscapes. Wildl. Res. 47, 1–15. doi: 10.1071/wr19027
Hunter, D. O., Lagisz, M., Leo, V., Nakagawa, S., and Letnic, M. (2018). Not all predators are equal: a continent-scale analysis of the effects of predator control on Australian mammals. Mamm. Rev. 48, 108–122. doi: 10.1111/mam.12115
James, C. D., Landsberg, J., and Morton, S. R. (1999). Provision of watering points in the Australian arid zone: a review of effects on biota. J. Arid Env. 41, 87–121. doi: 10.1006/jare.1998.0467
Keast, A. (1968). Seasonal movements in the Australian honeyeaters (Meliphagidae) and their ecological significance. Emu 67, 159–209. doi: 10.1071/mu967159
Keeley, J. E. (1986). “Resilience of Mediterranean shrub communities to fires,” in Resilience in Mediterranean-Type Ecosystems, eds D. Bell, A. J. M. Hopkins, and B. B. Lamont (Dordrecht: DrW Junk), 95–112. doi: 10.1007/978-94-009-4822-8_7
Keeley, J. E., Bond, W. J., Bradstock, R. A., Pausas, J. G., and Rundel, P. W. (2012). Fire in Mediterranean Ecosystems: Ecology, Evolution and Management. Cambridge: Cambridge University Press.
Keeley, J. E., Fotheringham, C. J., and Baer-Keeley, M. (2005). Determinants of postfire recovery and succession in Mediterranean-climate shrublands of California. Ecol. Appl. 15, 1515–1534. doi: 10.1890/04-1005
Keith, D. A. (2012). “Functional traits: their roles in understanding and predicting biotic responses to fire regimes from individuals to landscapes,” in Flammable Australia: Fire Regimes, Biodiversity and Ecosystems in a Changing World, eds R. A. Bradstock, A. M. Gill, and R. J. Williams (Collingwood: CSIRO Publishing), 97–125.
Keith, D. A., and Tozer, M. G. (2012). The influence of fire, herbivores and rainfall on vegetation dynamics in the mallee: a long-term experiment. Proc. Linn. Soc. NSW 134, A39–A54.
Kelly, L. T., Bennett, A. F., Clarke, M. F., and McCarthy, M. A. (2015). Optimal fire histories for biodiversity conservation. Conserv. Biol. 29, 473–481. doi: 10.1111/cobi.12384
Kelly, L. T., Dayman, R., Nimmo, D. G., Clarke, M. F., and Bennett, A. F. (2013). Spatial and temporal drivers of small mammal distributions in a semi-arid environment: the role of rainfall, vegetation and life-history. Aust. Ecol. 38, 786–797. doi: 10.1111/aec.12018
Kelly, L. T., Giljohann, K. M., Duane, A., Aquilué, N., Archibald, S., Batllori, E., et al. (2020). Fire and biodiversity in the Anthropocene. Science 370:eabb0355. doi: 10.1126/science.abb0355
Kelly, L. T., Nimmo, D. G., Spence-Bailey, L. M., Clarke, M. F., and Bennett, A. F. (2010). The short-term responses of small mammals to wildfire in semiarid mallee shrubland, Australia. Wildl. Res. 37, 293–300. doi: 10.1071/wr10016
Kelly, L. T., Nimmo, D. G., Spence-Bailey, L. M., Haslem, A., Watson, S. J., Clarke, M. F., et al. (2011). Influence of fire history on small mammal distributions: insights from a 100-year post-fire chronosequence. Divers. Distrib. 17, 462–473. doi: 10.1111/j.1472-4642.2011.00754.x
Kelly, L. T., Nimmo, D. G., Spence-Bailey, L. M., Taylor, R. S., Watson, S. J., Clarke, M. F., et al. (2012). Managing fire mosaics for small mammal conservation: a landscape perspective. J. Appl. Ecol. 49, 412–421. doi: 10.1111/j.1365-2664.2012.02124.x
Kenny, S. A., Bennett, A. F., Clarke, M. F., and Morgan, J. W. (2018). Time-since-fire and climate interact to affect the structural recovery of an Australian semi-arid plant community. Austral. Ecol. 43, 456–469. doi: 10.1111/aec.12582
Koch, P. J. (2015). Habitat 141 Landscape Conservation Action Plan. Summary Report. Unpublished Report for the Habitat 141 Alliance. Brisbane: Greening Australia.
Leblanc, M., Tweed, S., Van Dijk, A., and Timbal, B. (2012). A review of historic and future hydrological changes in the Murray-Darling Basin. Glob. Planet Change 80-81, 226–246. doi: 10.1016/j.gloplacha.2011.10.012
Leonard, S., Bennett, A. F., and Clarke, M. F. (2014). Determinants of the occurrence of unburnt patches: potential biotic refuges in a large intense wildfire in south-eastern Australia. For. Ecol. Manag. 314, 85–93. doi: 10.1016/j.foreco.2013.11.036
Letnic, M., Dickman, C. R., Tischler, M. K., Tamayo, B., and Beh, C.-L. (2004). The responses of small mammals and lizards to post-fire succession and rainfall in arid Australia. J. Arid Environ. 59, 85–114. doi: 10.1016/j.jaridenv.2004.01.014
Letnic, M., Koch, F., Gordon, C., Crowther, M. S., and Dickman, C. R. (2009). Keystone effects of an alien top predator stem extinctions of native mammals. Proc. R. Soc. B 276, 3249–3256. doi: 10.1098/rspb.2009.0574
Letnic, M., Ritchie, E. G., and Dickman, C. R. (2012). Top predators as biodiversity regulators: the dingo Canis lupus dingo as a case study. Biol. Rev. 87, 390–413. doi: 10.1111/j.1469-185x.2011.00203.x
Long, M. (2006). A climatology of extreme fire weather days in Victoria. Aust. Meteor. Mag. 55, 3–18.
Ludwig, J. A., Macleod, N. D., and Noble, J. C. (1990). “An expert system for fire management in mallee reserves,” in The Mallee Lands – a Conservation Perspective, eds J. C. Noble, P. J. Joss, and G. K. Jones (East Melbourne, VIC: CSIRO Publishing), 214–217.
Luke, R. H., and McArthur, A. G. (1978). Bushfires in Australia. Canberra, VIC: Australian Government Publishing Service.
Lumsden, L., Nelson, J., and Lindeman, M. (2008). Ecological Research on the Eastern Long-eared Bat Nyctophilus timoriensis (South-Eastern Form). A Report to the Mallee Catchment Management Authority. Heidelberg, VIC: Arthur Rylah Institute for Environmental Research, Department of Sustainability and Environment.
Mariani, M., Holz, A., Veblen, T. T., Williamson, G., Fletcher, M.-S., and Bowman, D. M. J. S. (2018). Climate change amplifications of climate-fire teleconnections in the Southern Hemisphere. Geophys. Res. Lett. 45, 5071–5081. doi: 10.1029/2018gl078294
McCaw, W. L. (1997). Predicting Fire Spread in Western Australian Mallee-Heath Shrubland. PhD thesis. Sydney, NSW: University of New South Wales.
Menkhorst, P. W., and Bennett, A. F. (1990). “Vertebrate fauna of mallee vegetation in southern Australia,” in The Mallee Lands: A Conservation Perspective, eds J. C. Noble, P. J. Joss, and G. K. Jones (East Melbourne, VIC: CSIRO Publishing), 39–53.
Morton, S. R., Stafford Smith, D. M., Dickman, C. R., Dunkerley, D. L., Friedel, M. H., McAllister, R. R., et al. (2011). A fresh framework for the ecology of arid Australia. J. Arid Environ. 75, 313–329. doi: 10.1016/j.jaridenv.2010.11.001
National Parks and Wildlife Service (2020). NPWS Fire History - Wildfires and Prescribed Burns. Available online at: https://data.gov.au/dataset/ds-nsw-1f694774-49d5-47b8-8dd0-77ca8376eb04/details?q= (accessed on December 2020).
Nimmo, D. G., Avitabile, S., Banks, S. C., Bliege Bird, R., Callister, K., Clarke, M. F., et al. (2019). Animal movements in fire-prone landscapes. Biol. Rev. 94, 981–998.
Nimmo, D. G., Kelly, L. T., Farnsworth, L. M., Watson, S. J., and Bennett, A. F. (2014). Why do some species have geographically varying responses to fire history? Ecography 37, 805–813. doi: 10.1111/ecog.00684
Nimmo, D. G., Kelly, L. T., Spence-Bailey, L. M., Watson, S. J., Haslem, A., White, J. G., et al. (2012). Predicting the century-long post-fire responses of reptiles. Glob. Ecol. Biogeog. 21, 1062–1073. doi: 10.1111/j.1466-8238.2011.00747.x
Nimmo, D. G., Kelly, L. T., Spence-Bailey, L. M., Watson, S. J., Taylor, R. S., Clarke, M. F., et al. (2013). Fire mosaics and reptile conservation in a fire-prone region. Cons. Biol. 159, 248–256.
Noble, J., and Vines, R. (1993). Fire studies in mallee (Eucalyptus spp.) communities of western New South Wales: grass fuel dynamics and associated weather patterns. Rangeland J. 15, 270–297. doi: 10.1071/rj9930270
Noble, J. C. (2001). Lignotubers and meristem dependence in mallee (Eucalyptus spp.) coppicing after fire. Aust. J. Bot. 49, 31–41.
Noble, J. C., and Bradstock, R. A. (eds) (1989). Mediterranean Landscapes in Australia: Mallee Ecosystems and Their Management. Melbourne, VIC: CSIRO Publishing.
Noble, J. C., Joss, P. J., and Jones, G. K. (eds) (1990). The Mallee Lands: A Conservation Perspective. East Melbourne, VIC: CSIRO Publications.
Noble, J. C., and Kimber, R. G. (1997). On the ethnoecology of mallee root-water. Aborig. Hist. 21, 170–202.
Noble, J. C., Smith, A. W., and Leslie, H. W. (1980). Fire in the mallee shrublands of western New South Wales. Aust. Rangel. J. 2, 104–114. doi: 10.1071/rj9800104
O’Donnell, A. J., Boer, M. M., McCaw, W. L., and Grierson, P. F. (2011a). Climatic anomalies drive wildfire occurrence and extent in semi-arid shrublands and woodlands of southwest Australia. Ecosphere 2, 1–15.
O’Donnell, A. J., Boer, M. M., McCaw, W. L., and Grierson, P. F. (2011b). Vegetation and landscape connectivity control wildfire intervals in unmanaged semi-arid shrublands and woodlands in Australia. J. Biogeogr. 38, 112–124. doi: 10.1111/j.1365-2699.2010.02381.x
O’Donnell, A. J., Boer, M. M., McCaw, W. L., and Grierson, P. F. (2014). Scale-dependent thresholds in the dominant controls of wildfire size in semi-arid southwest Australia. Ecosphere 5:93.
Parkes, D. M., and Cheal, D. C. (1990). “Perceptions of mallee vegetation,” in The Mallee Lands: A Conservation Perspective, eds J. C. Noble, P. J. Joss, and G. K. Jones (East Melbourne, VIC: CSIRO Publications), 3–7.
Parr, C. L., and Andersen, A. N. (2006). Patch mosaic burning for biodiversity conservation: a critique of the pyrodiversity paradigm. Cons. Biol. 20, 1610–1619. doi: 10.1111/j.1523-1739.2006.00492.x
Parsons, B. C., and Gosper, C. R. (2011). Contemporary fire regimes in a fragmented and an unfragmented landscape: implications for vegetation structure and persistence of the fire-sensitive malleefowl. Int. J. Wild. Fire 20, 184–194. doi: 10.1071/wf09099
Parsons, R. F., and Rowan, J. N. (1968). Edaphic range and cohabitation of some mallee eucalypts in south-eastern Australia. Aust. J. Bot. 16, 109–116. doi: 10.1071/bt9680109
Paton, D. C. (1980). The importance of manna, honeydew and lerp in the diet of honeyeaters. Emu 80, 213–226. doi: 10.1080/01584197.1980.11799277
Pausas, J. G., and Bradstock, R. A. (2007). Fire persistence traits of plants along a productivity and disturbance gradient in Mediterranean shrublands of south-east Australia. Glob. Ecol. Biogeogr. 16, 330–340. doi: 10.1111/j.1466-8238.2006.00283.x
Pell, S. D., Chivas, A. R., and Williams, I. S. (2001). The Mallee Dunefield: development and sand provenance. J. Arid Environ. 48, 149–170. doi: 10.1006/jare.2000.0751
Pianka, E. R. (1969). Habitat specificity, speciation, and species density in Australian desert lizards. Ecology 50, 498–502. doi: 10.2307/1933908
Pickett, S. T. A., Collins, S. L., and Armesto, J. J. (1987). Models, mechanisms and pathways of succession. Bot. Rev. 53, 335–371. doi: 10.1007/bf02858321
Pollock, L. J., Kelly, L. T., Thomas, F. M., Soe, P., Morris, W. K., White, M., et al. (2018). Combining functional traits, the environment and multiple surveys to understand semi-arid tree distributions. J. Veg. Sci. 29, 967–977. doi: 10.1111/jvs.12686
Prober, S. M., Yuen, E., O’Connor, M. H., and Schultz, L. (2016). Ngadju kala: Australian Aboriginal fire knowledge in the Great Western Woodlands. Austral. Ecol. 41, 716–732. doi: 10.1111/aec.12377
Rainsford, F. W., Kelly, L. T., Leonard, S. W. J., and Bennett, A. F. (2020). Post-fire development of faunal habitat depends on plant regeneration traits. Austral. Ecol. 45, 800–812.
Read, J. L., Firn, J., Grice, A. C., Murphy, R., Ryan-Colton, E., and Schlesinger, C. A. (2020). Ranking buffel: comparative risk and mitigation costs of key environmental and socio-cultural threats in central Australia. Ecol. Evol. 10, 12745–12763. doi: 10.1002/ece3.6724
Richards, T., Pavlides, C., Walshe, K., Webber, H., and Johnston, R. (2007). Box Gully: new evidence for Aboriginal occupation of Australia south of the Murray River prior to the last glacial maximum. Arch. Ocean. 42, 1–11. doi: 10.1002/j.1834-4453.2007.tb00001.x
Ritchie, E. G., Elmhagen, B., Glen, A. S., Letnic, M., Ludwig, G., and McDonald, R. A. (2012). Ecosystem restoration with teeth: what role for predators? Trends Ecol. Evol. 27, 265–271. doi: 10.1016/j.tree.2012.01.001
Ross, A. (1981). Holocene environments and prehistoric site patterning in the Victorian mallee. Arch. Ocean. 16, 145–155. doi: 10.1002/j.1834-4453.1981.tb00025.x
Sandell, P. (2006). Promoting woodland recovery in the Victorian mallee parks. Proc. R. Soc. Vict. 118, 313–321.
Sandell, P., Tolhurst, K. G., Dalton, J., Scott, B., and Smith, M. (2006). Fire management prescriptions for the Victorian Mallee Parks. Proc. R. Soc. Vic. 118, 395–412.
Schlesinger, C. A., Noble, I. R., and Weir, T. (1997). Fire studies in mallee (Eucalyptus spp.) communities of western New South Wales: reptile and beetle populations in sites of differing fire history. Rangelands J. 19, 190–205. doi: 10.1071/rj9970190
Schodde, R. (1981). “Bird communities of the Australian mallee: composition, derivation, distribution, structure and seasonal cycles,” in Ecosystems of the World. II Mediterranean-Type Shrublands, eds F. di Castri, D. W. Goodall, and R. L. Specht (Amsterdam: Elsevier), 387–415.
Schodde, R. (1990). “The bird fauna of the mallee - its biogeography and future,” in The Mallee Lands: a Conservation Perspective, eds J. C. Noble, P. J. Joss, and G. K. Jones (Melbourne, VIC: CSIRO), 61–70.
Schodde, R., and Mason, I. J. (1999). The Directory of Australian Birds - Passerines: A Taxonomic and Zoogeographic Atlas of the Biodiversity of Birds in Australia and its Territories. Collingwood: CSIRO Publishing.
Selwood, K. E., Cunningham, S. C., and Mac Nally, R. (2019). Beyond refuges: identifying temporally dynamic havens to support ecological resistance and resilience to climatic disturbances. Biol. Cons. 233, 131–138. doi: 10.1016/j.biocon.2019.02.034
Senior, K. L., Giljohann, K. M., McCarthy, M. A., Rainsford, F. W., and Kelly, L. T. (2021). Predicting mammal responses to pyrodiversity: from microbats to macropods. Biol. Conserv. 256:109031. doi: 10.1016/j.biocon.2021.109031
Simms, A., Scott, M., Watson, S., and Leonard, S. (2019). Attenuated post-fire fauna succession: the effects of surrounding landscape context on post-fire colonisation of fauna. Wildl. Res. 46, 247–255. doi: 10.1071/wr18131
Smith, A., Avitabile, S. C., and Leonard, S. W. J. (2017). Less fuel for the fire: malleefowl (Leipoa ocellata) nesting activity affects fuel loads and fire behaviour. Wildl. Res. 43, 640–648. doi: 10.1071/wr16127
Smith, A. L., Bull, C. M., and Driscoll, D. A. (2013). Successional specialization in a reptile community cautions against widespread planned burning and complete fire suppression. J. Appl. Ecol. 50, 1178–1186. doi: 10.1111/1365-2664.12119
Smith, A. L., Landguth, E. L., Bull, C. M., Banks, S. C., Gardner, M. G., and Driscoll, D. A. (2016). Dispersal responses override density effects on genetic diversity during post-disturbance succession. Proc. R. Soc. B: Biol. Sci. 283, 20152934. doi: 10.1098/rspb.2015.2934
Smith, W., Neale, T., and Weir, J. K. (2021). Persuasion without policies: the work of reviving Indigenous peoples’ fire management in southern Australia. Geoforum 120, 82–92. doi: 10.1016/j.geoforum.2021.01.015
Sparrow, A. (1989). “Mallee vegetation in South Australia,” in Mediterranean Landscapes in Australia: Mallee Ecosystems and their Management, eds J. C. Noble and R. A. Bradstock (Victoria, VIC: CSIRO Publishing), 109–124.
Speer, M. S. (2013). Dust storm frequency and impact over eastern Australia determined by state of Pacific climate system. Weather Clim. Extremes 2, 16–21. doi: 10.1016/j.wace.2013.10.004
Taylor, R. S., Watson, S. J., Nimmo, D. G., Kelly, L. T., Bennett, A. F., and Clarke, M. F. (2012). Landscape-scale effects of fire on bird assemblages: does pyrodiversity beget biodiversity? Divers. Distrib. 18, 519–529. doi: 10.1111/j.1472-4642.2011.00842.x
Teasedale, L. C., Smith, A. L., Thomas, M., Whitehead, C. A., and Driscoll, D. A. (2013). Detecting invertebrate responses to fire depends on sampling method and taxonomic resolution. Aust. Ecol. 38, 874–883. doi: 10.1111/aec.12024
Travers, S. K., and Eldridge, D. J. (2013). Increased rainfall frequency triggers an increase in litter fall rates of reproductive structures in an arid eucalypt woodland. Aust. Ecol. 38, 820–830. doi: 10.1111/aec.12055
Tyson, M., Vaillancourt, R. E., and Reid, J. B. (1998). Determination of clone size and age in a mallee eucalypt using RAPDs. Aust. J. Bot. 46, 161–172. doi: 10.1071/bt97003
Verdon, S. J., Watson, S. J., and Clarke, M. F. (2019). Modelling variability in the fire response of an endangered bird to improve fire-management. Ecol. Appl. 29, e01980. doi: 10.1002/eap.1980
Verdon, S. J., Watson, S. J., Nimmo, D. G., and Clarke, M. F. (2020). Are all fauna associated with the same structural features of the foundation species Triodia scariosa? Austral Ecol. 45, 773–787.
Victorian Traditional Owner Cultural Fire Knowledge and Group (2019). The Victorian Traditional Owner Cultural Fire Strategy. Melbourne, VIC: Federation of Victorian Traditional Owner Corporations, Department of Environment, Water and Planning, Parks Victoria, Country Fire Authority.
Wasson, R. J. (1989). “Landforms,” in Mediterranean Landscapes in Australia: Mallee Ecosystems and their Management, eds J. C. Noble and R. A. Bradstock (Victoria, VIC: CSIRO Publishing), 13–34.
Watson, S. J., Taylor, R. S., Nimmo, D. G., Kelly, L. T., Clarke, M. F., and Bennett, A. F. (2012a). The influence of unburnt patches and distance from refuges on post-fire bird communities. Anim. Conserv. 15, 499–507.
Watson, S. J., Taylor, R. S., Nimmo, D. G., Kelly, L. T., Haslem, A., Clarke, M. F., et al. (2012b). Effects of time since fire on birds: how informative are generalized fire response curves for conservation management? Ecol. Appl. 22, 685–696.
Wellington, A. B., and Noble, I. R. (1985). Post-fire recruitment and mortality in a population of the mallee Eucalyptus incrassata in semi-arid, south-eastern Australia. J. Ecol. 73, 645–656.
Westbrooke, M. E., and Florentine, S. K. (2005). Rainfall-driven episodic flood events: are they a major factor in moulding New South Wales arid land vegetation patterns? Aust. Geog. 36, 171–181.
White, M., Oates, A., Barlow, T., Pelikan, M., Brown, J., Rosengren, N., et al. (2003). The Vegetation of North-West Victoria: a Report to the Wimmera, North Central and Mallee Catchment Management Authorities. Melbourne, VIC: Arthur Rylah Institute for Environmental Research.
White, M. D. (2006). The mallee vegetation of north western Victoria. Proc. R. Soc. Vic. 118, 229–243.
Wilson, A. D., Hodgkinson, K. C., and Noble, J. C. (1988). “Vegetation attributes and their application to the management of Australian rangelands,” in Vegetation Science Applications for Rangeland Analysis and Management, ed. P. T. Tueller (Netherlands: Springer), 253–294.
Keywords: fire regimes, mallee, biodiversity, species responses to fire, pyrodiversity
Citation: Clarke MF, Kelly LT, Avitabile SC, Benshemesh J, Callister KE, Driscoll DA, Ewin P, Giljohann K, Haslem A, Kenny SA, Leonard S, Ritchie EG, Nimmo DG, Schedvin N, Schneider K, Watson SJ, Westbrooke M, White M, Wouters MA and Bennett AF (2021) Fire and Its Interactions With Other Drivers Shape a Distinctive, Semi-Arid ‘Mallee’ Ecosystem. Front. Ecol. Evol. 9:647557. doi: 10.3389/fevo.2021.647557
Received: 30 December 2020; Accepted: 28 April 2021;
Published: 25 May 2021.
Edited by:
Eddie John Van Etten, Edith Cowan University, AustraliaReviewed by:
Alison O’Donnell, University of Western Australia, AustraliaCarl R. Gosper, Conservation and Attractions (DBCA), Australia
Copyright © 2021 Clarke, Kelly, Avitabile, Benshemesh, Callister, Driscoll, Ewin, Giljohann, Haslem, Kenny, Leonard, Ritchie, Nimmo, Schedvin, Schneider, Watson, Westbrooke, White, Wouters and Bennett. This is an open-access article distributed under the terms of the Creative Commons Attribution License (CC BY). The use, distribution or reproduction in other forums is permitted, provided the original author(s) and the copyright owner(s) are credited and that the original publication in this journal is cited, in accordance with accepted academic practice. No use, distribution or reproduction is permitted which does not comply with these terms.
*Correspondence: Michael F. Clarke, m.clarke@latrobe.edu.au