Anthropogenically-induced range expansion as an invasion front in native species: An example in North American flying squirrels
- Department of Fish and Wildlife Conservation, Virginia Tech, Blacksburg, VA, United States
Biological invasions are typically framed as non-native species impacting the populations of native species or ecosystems. However, in a changing world, taxonomically similar native species that were previously parapatric or allopatric may become increasingly sympatric over short time periods (<100 years). In the context of climate change in the Northern Hemisphere, this may have a negative impact on northern species whose ranges are being invaded by southern species. To highlight factors that may influence invasion fronts in native species, I use two species of North American flying squirrels, small-bodied nocturnal arboreal Sciurids, as an example. I discuss what factors may enable or limit the expansion of southern flying squirrels (SFS; Glaucomys fuscus) into northern flying squirrel (NFS; Glaucomys sabrinus) habitat and potential impacts that anthropogenically-induced factors have on range shift dynamics. The range expansion of SFS may impact NFS via resource competition, hybridization, and parasite-mediation. Factors potentially enabling the expansion of SFS into NFS habitat include anthropogenic habitat disturbance and climate change, wherein historical land-use (i.e., logging) alters forest composition increasing habitat suitability for SFS and a warming climate allows SFS to expanded their ranges northward into colder regions. Shifts in forest species composition from historical logging may interact with a warming climate to enable SFS to quickly expand their range. Factors limiting SFS expansion include thermoregulation limitations and absence of potential food and denning resources. The factors influencing the dynamics between these two species may be applicable to the shifting ranges of other taxonomically and functionally similar native species in the context of a rapidly changing world in the Anthropocene.
1. Introduction
Biological invasions are considered one of the greatest threats impacting biodiversity worldwide, wherein anthropogenically introduced invasive species are causing native species declines (Bellard et al., 2016) and negatively impacting ecosystem functions (Fleming et al., 2013; Zipkin et al., 2020). Typically, the focus of biological invasions is on species introduced between continents, such as eastern grey squirrels (Sciurus carolinensis) from North America to Europe (Gurnell et al., 2004; Bertolino et al., 2014) or European rabbits (Oryctolagus cuniculus) to Australia (Mutze et al., 2016; Roy-Dufresne et al., 2019). However, shifting ranges of native species due to anthropogenic activities and climate change over short time periods (e.g., <100 years) may have negative effects on resident native species whose ranges are being invaded by creating non-analogue conditions (e.g., novel conditions that different from the baseline conditions; Peel et al., 2017; Wallingford et al., 2020).
Since biological invasions are characterized by their celerity compared to shifts in species’ ranges over longer biological timescales (Valéry et al., 2008), there is increasing support to view some species with anthropogenically-induced range expansion as invasive and to understand the mechanisms that allow them to displace other native species (Valéry et al., 2009; Wallingford et al., 2020). Although classifying a native species as invasive is a debated topic in the field of invasion biology (Essl et al., 2019; Urban, 1988), native species can negatively impact other native species or ecosystems via anthropogenically-induced range expansions into new systems, causing resident species declines or trophic cascades (Newsome and Ripple, 2014; Holm et al., 2016). Native species taking advantage of human-modified environments to expand their ranges and establish in novel areas without direct human interference (e.g., intentional introductions) are called “neonatives” (Essl et al., 2019).
Neonatives can negatively impact the distribution and populations of native resident species, especially where species were previously parapatric (Kelly et al., 2003; Elmhagen et al., 2017). For taxonomically similar species, hybridization within a newly established sympatric zone may have conservation implications for resident species (Mank et al., 2004; Gómez et al., 2015), particularly for endangered subpopulations or if resident species have highly fragmented distributions. Anthropogenically-induced range shifts that lead to novel sympatry may also increase rates of hybridization (Stronen et al., 2012).
Recent climate-related range expansions and extirpations are already occurring in mammal species (Chin et al., 2011; Wiens, 2016). Elevational and latitudinal shifts in small mammal ranges have occurred over the last century in North America (Mortiz et al., 2008; Myers et al., 2009; Rowe et al., 2009). Factors influencing potential range shifts tend to consist of abiotic and biotic factors which interact to impede or facilitate changes in a species’ distribution (Sexton et al., 2009; HillRisLambers et al., 2013; Miller et al., 2020).
Although climate change is linked to range shifts, other factors such as anthropogenic land use (e.g., habitat conversion to agriculture, fire regime shifts, logging) have also influenced shifts in species distributions (Rowe et al., 2009; Rowe and Terry, 2014; Kelt et al., 2017). Little attention has been given to how anthropogenic habitat alteration may allow native species to naturally disperse and colonize areas they were unable to colonize previously (Essl et al., 2019). Anthropogenic alterations to habitat may also interact with climate change to influence species range shifts (Rowe, 2007; Wan et al., 2022), particularly at the most southern or northern distributions of their ranges (McCain and King, 2014; Essl et al., 2019). Some species with range contractions may be tracking climate change, but range contractions could also be due to competition with a novel native competitor with a recently expanded range.
In North America, range shifts and hybridization of two species of flying squirrel (Glaucomys spp.) has occurred. In recent decades, there has been rapid range expansions of southern flying squirrel (SFS; G. volans) into northern flying squirrel (NFS; G. sabrinus) habitat (Bowman et al., 2005; Myers et al., 2009; Garroway et al., 2011; Lazure et al., 2016). Other observations show the recent sympatry or replacement of NFS by SFS at several sites (Myers et al., 2009; Wood et al., 2016; Diggins et al., 2020b; O’Brien et al., 2022). Hybridization between SFS and NFS has been observed in contact zones (Garroway et al., 2010). Since the rapid range expansion of SFS may have negative conservation implications for NFS (Weigl, 2007), my objective is to discuss SFS as a potential neonative. I discuss the ecology of flying squirrels, distribution of both NFS and SFS, and recently observed range shifts of SFS to provide context on these species. I then examine how interspecific factors that may influence species turnover, as well as factors that aid or limit SFS range expansion.
2. Ecology of flying squirrels
North American flying squirrels are nocturnal, arboreal Sciurids occurring in forested habitats (Figure 1; Hough and Dieter, 2009; Diggins et al., 2017; Dolan and Carter, 1977; Wells-Gosling and Heaney, 1984). Associated with boreal and montane conifer forests (e.g., Abies spp., Picea spp., Tsuga spp.), mixed forests, and occasionally deciduous forests, NFS occur in Canada and the northern United States with disjunct populations in the Rocky Mountains, Black Hills, and Appalachian Mountains (Hough and Dieter, 2009; Diggins et al., 2017). Smaller-bodied than NFS, SFS is associated with temperate to sub-tropical deciduous, coniferous (Pinus spp.), and mixedwood forests in the eastern half of United States and southeastern Canada (Figure 1; Gilmore and Gates, 1985; Taulman and Smith, 2004; Jacques et al., 2017), with disjunct populations in montane forests in Mexico and Central America (Campuano-Chávez-Peón et al., 2014). Both species have varied diets consisting of plant materials (e.g., nuts, seeds, berries), fungi, lichen, and carrion (Dolan and Carter, 1977; Wells-Gosling and Heaney, 1984; Mitchell, 2001), although hard mast (i.e., Carya spp. nuts, Quercus spp. acorns, Fagus grandifolia beechnuts) is important to SFS during winter and is typically stored in caches (Muul, 1968; Thomas and Weigl, 1998; Helmick et al., 2014).
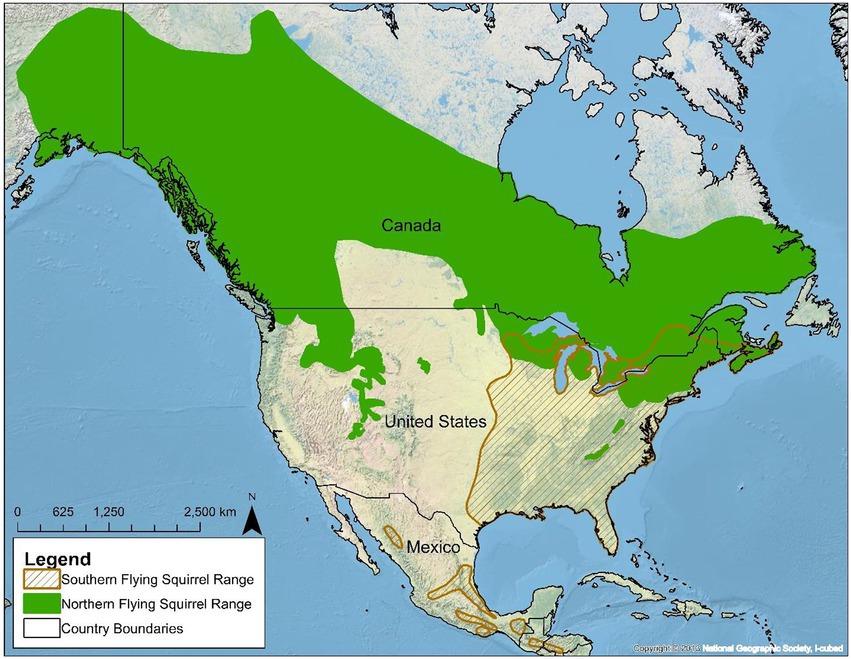
Figure 1. The geographic range of northern flying squirrels (Glaucomys sabrinus) and southern flying squirrels (Glaucomys volans) in North America.
Both species are highly social, exhibiting overlapping home ranges with conspecifics (Bendel and Gates, 1987; Holloway and Malcolm, 2007; Jacques et al., 2017; Diggins and Ford, 2021), communal denning (Stihler et al., 1987; Layne and Raymond, 1994; Reynolds et al., 2009), and large vocal repertoires for intraspecific communication, including alarm and mating calls (Gilley et al., 2019; Diggins, 2021). Denning aggregations can occur year-round (Reynolds et al., 2009; Diggins and Ford, 2021; Doty et al., 2022), but aggregation size typically increases in winter to reduce thermoregulatory costs associated with colder months of the year (Muul, 1968; Stapp et al., 1991; Thorington et al., 2010). Denning aggregations can contain related and unrelated individuals (Thorington et al., 2010; Garroway et al., 2013), wherein related aggregations in spring and summer are typically natal aggregations (i.e., nursing mother and offspring; Doty et al., 2022). Average litter size is similar between both species, although SFS litters are slightly larger on average (Dolan and Carter, 1977; Wells-Gosling and Heaney, 1984). Females typically produce one litter a year, although individuals of both species have been observed producing two litters a year (Reynolds et al., 2009; Patterson and Patterson, 2010; Smith et al., 2011).
3. Geographic range and sympatry
Many small mammal species are habitat specialists and do not occur in all habitats within their geographic range. Overlapping ranges between taxonomically similar small mammal species are typically segregated by habitat type or utilization of different resources allowing coexistence within an area (M’Closkey, 1978; Murúa and González, 1982; McCay et al., 2004). Additionally, behavioral factors, such as aggressive interspecific interactions, may partition sympatric sister species into certain habitats (Chappell, 1978; Bleich and Price, 1995).
Both NFS and SFS squirrels typically occur in distinct habitats across their large geographic ranges. In the eastern part of their range, NFS is associated with cooler montane and boreal conifer and mixedwood forests, which provide important food sources such as hypogeal fungi. Throughout their range, SFS is associated with more austral forests, especially woodlands with hard mast trees. Although NFS and SFS are mostly allopatric throughout the majority of their geographic ranges, these species are parapatric to sympatric along the southern peripheries of NFS geographic distribution and the northern parts of SFS geographic distribution (Figure 1). Where sympatry does occur, it typically is found in mixed hardwood-conifer ecotones and forests in the Appalachian Mountains, New England, Great Lakes, and the southern portion of the Canadian Shield.
Sympatry between NFS and SFS where their ranges meet or overlap is not universal. Some long-term studies (~20 years) around the Great Lakes Region show stable zones of local sympatry. But in the central and southern Appalachian Mountains, 30+ years of long-term nest box monitoring show strong habitat segregation between the species and a lack of local sympatry. In some areas sympatry is recent (<30 years) and resulted in species turnover (Wood et al., 2016; Diggins et al., 2020b; O’Brien et al., 2022). Although certain sites exhibit stable NFS-SFS sympatry in the Great Lakes Region, it is unknown if NFS-SFS sympatry occurred historically or if ~20 year of monitoring can accurately assess stable sympatry given that species turnover may take decades to observe. Unstable sympatry (i.e., where sympatry dynamically occurs during certain times of year or during good mast years between parapatric populations of NFS and SFS, but is not constant) has been observed in the southern Appalachians (Weigl et al., 1992; Weigl, 2007). In interspecific interactions, SFS is considered more aggressive than NFS and may exclude NFS from nest sites (Weigl, 1978). Despite some cooccurrence resulting in unstable sympatry within tension zones or localized sympatry within certain parts of their range, resource specialization and interspecific aggression are considered the two main factors reducing probability of sympatry for both species (Muul, 1968; Weigl, 1978).
4. Observed range shifts
Recent range shifts have occurred in both SFS and NFS. SFS expanded their known range by approximately 200 km in Ontario, Canada (Bowman et al., 2005; Garroway et al., 2011), 225 km in Michigan, USA (Myers et al., 2009), and 60–150 km in Quebec, Canada (Lazure et al., 2016). The establishment of SFS where they were once absent or rare has led to the decline or extirpation of NFS from some of those areas (Myers et al., 2009; Wood et al., 2016). In Pennsylvania, where NFS is a state endangered species, some sites only hosted NFS historically, but now NFS are sympatric or have been replaced by SFS (Mahan et al., 1999; Diggins et al., 2020b). Shifts in ranges may occur rapidly: repeated surveys in Michigan across a 30-year period showed the northward range expansion of SFS and northward range contraction of NFS from the southern to the northern part of the state (Myers et al., 2009). Range shifts can also be dynamic: SFS expanded their range northward during a 40-year period in Ontario, but also showed a partial range collapse in the newly expanded area over a short time period (Bowman et al., 2005). Species replacement rates have also occurred in less than 20 years (Wood et al., 2016; O’Brien et al., 2022), possibly leading to the extirpation or decline of local populations of NFS over relatively short time periods. However, range shifts and species turnover rates may be impacted by multiple interacting factors that are not uniform across the contact zone for these species.
5. Interspecific factors influencing species turnover
When two taxonomically similar species co-occur sympatrically in an area, various factors may influence the coexistence of both species or the replace of one species over the other. In the case of NFS and SFS, these species tend to be parapatric or sympatric in areas of overlap. In parts of their range where NFS and SFS tend to be parapatric (e.g., the southern Appalachians), narrow zones of unstable sympatry along conifer-hardwood ecotones are typical (Weigl, 2007). I will discuss three factors driven by species interactions that may influence NFS replacement by SFS on a local scale: parasite-mediation, hybridization, and resource competition (Figure 2).
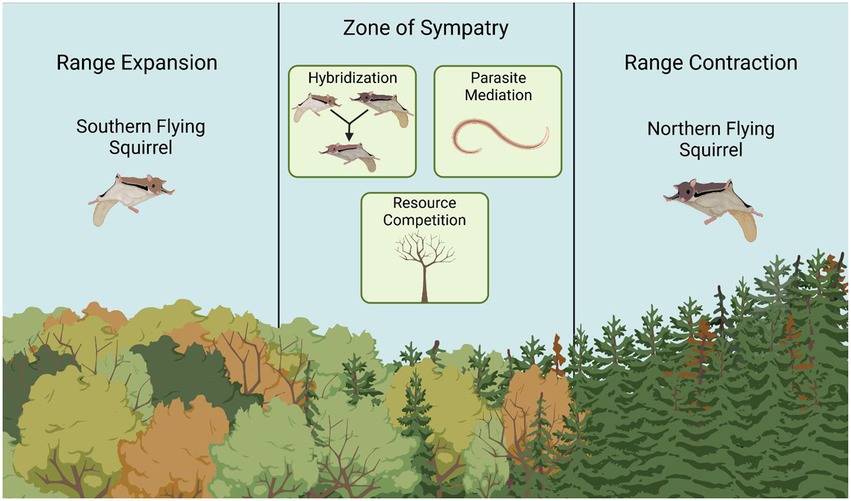
Figure 2. Southern flying squirrels (SFS; Glaucomys volans) and northern flying squirrels (NFS; Glaucomys sabrinus) exhibit geographic range overlap in the Appalachians, New England, and the Great Lakes Region in North America. Anthropogenically-induced range shifts due to historical land use and climate change have caused the range expansion of SFS and range contraction of NFS to higher latitudes and elevations. In zones of sympatry, hybridization, parasite mediation via the nematode Strongyloides robustus, and competition for resources such as nest trees, may be factors contributing the displacement of NFS by SFS. This figure was made with BioRender (www.biorender.com) and edited in Microsoft Paint.
5.1. Parasite-mediated competition
Climate change can exacerbate parasite-mediated competition between species by altering the survival, development, and transmission of parasites, causing unpredictable ecological impacts (Polley et al., 2010; Carlson et al., 2017). Novel interspecific parasite-mediated competition may help facilitate an invading species’ replacement of a resident species by reducing survival of the resident species (Romeo et al., 2021), especially where invading species are now sympatric with endangered resident populations (Mahan and Steele, 2022).
Strongyloides robustus is a nematode parasite that occurs in both NFS and SFS, as well as other sympatric squirrel species such as eastern grey squirrels (Sciurus carolinensis) and American red squirrels (Tamiasciurus hudsonicus; Weigl, 1978; Espenshade and Stewart, 2013; O’Brien et al., 2022). S. robustus infections have no discernable impacts on SFS, but can be deleterious to NFS leading to decreases in body condition and even mortality (Weigl, 1978; O’Brien et al., 2022). The nematode causes damage to the lungs and small intestines, impacting the nutritional state and health of NFS, causing emaciation and increasing the squirrel’s susceptibility to pneumonia (Weigl et al., 1992). The range of S. robustus is thought to be limited by cold temperatures, which reduce the hatching success of eggs and extend the time for larval development compared to warmer temperatures (Wetzel and Weigl, 1994). However, S. robustus occurrence in Nova Scotia, Canada (Bartlett, 1995) may indicate geographic variation in cold tolerance in this species. Climate change is predicted to allow the expansion of S. robustus to higher latitudes and elevations (Weigl, 2007), potentially leading to persistent populations of S. robustus in areas that may have previously been unfavorable for establishment due to colder temperatures. Recent range expansion of S. robustus into Ontario was associated with northward SFS range expansion (Coombs, 2010), indicating climate-induced range shifts in SFS may facilitate the spread of S. robustus to higher latitudes and elevations.
Transmission of nematodes between SFS and NFS may occur at shared denning sites or feeding areas (Weigl, 2007), with transmission most likely occurring in nests previously occupied by SFS or during interspecific nesting aggregations. Nesting material may provide suitable microclimates for S. robustus eggs or larvae to survive during colder winter months long enough to infect their hosts (Pauli et al., 2004), especially in nests that contain larger aggregations of flying squirrels, such as natal denning aggregations during early spring or large winter aggregations (Wetzel and Weigl, 1994). Soil substrates can also host S. robustus and allow parasite transmission from SFS to NFS in feeding areas shared by both species (Weigl, 2007).
Potential species turnover rates mediated by S. robustus infections may be associated with milder winters and population declines could occur over short time periods. In North Carolina, USA, NFS and SFS had high population numbers within a sympatric zone along the northern hardwood-spruce ecotone in the 1980s. S. robustus prevalence in NFS increased after two mild winters and was subsequently followed by a decline in the NFS population (Weigl et al., 1992), indicating a potential parasite-induced population reduction within a short timeframe. Anthropogenic modifications to NFS habitat may have enabled SFS invasion of higher elevation sites in North Carolina during the summer months, facilitating the spread of S. robustus (Weigl, 2007). Capture success of NFS was substantially lower in trapping efforts at the same sites in the 2010s (Diggins et al., 2017) compared to the 1980s (Weigl et al., 1992) despite similar methodology. Shifts in capture success potentially indicate a lack of long-term recovery in NFS populations after population declines coincided with the first detections of S. robustus in those North Carolina populations. Although a recent study in a SFS-NFS hybrid zone across 30 sites in Canada found no support for parasite-mediated competition between the two species (O’Brien et al., 2022), this study only occurred for one season. Another study in a recent hybrid zone in Ontario found S. robustus in NFS was most likely caused by interspecies transmission, but prevalence was too low to facilitate parasite-mediated competition (Coombs, 2010), although this study was also limited to one field season. Additionally, O’Brien et al. (2022) found a weak relationship between S. robustus prevalence and NFS body condition, but how infection influences body condition over time was not explored. Krichbaum et al. (2010) found no evidence of negative impacts of S. robustus infection on the body condition of wild NFS specimens, but only 4 specimens were examined providing limited inference.
The impact of S. robustus infection may be difficult to detect in the field, especially if individual recapture rates are low, reducing the ability to track infection rates and body condition over time. Infection rates may vary seasonally, where the lowest prevalence of S. robustus occurs in winter and highest in summer and early fall (Weigl et al., 1992). Moreover, a study on the impacts of S. robustus on European red squirrel (S. vulgaris) survival in Italy required 9 continuous years of monitoring data to be able to detect the parasite’s impact on squirrel populations (Romeo et al., 2021). Therefore, long-term monitoring may be required to determine which factors aid in the spread of S. robustus and how this parasite may help facilitate species turnover at a site across time.
High prevalence of S. robustus in sympatric squirrels indicates other potential reservoirs besides SFS (Espenshade and Stewart, 2013; O’Brien et al., 2022). Interactions between diurnal eastern grey squirrels and American red squirrels with nocturnal flying squirrels may be limited due to differences in their activity patterns. For example, NFS only exhibited S. robustus infections when sympatric with SFS, but not in parts of its range where it was sympatric with red squirrels and SFS did not occur (Krichbaum et al., 2010). However, the transmission of S. robustus could still occur due to heterospecific space use (e.g., use of the same nest sites).
The prevalence of S. robustus varies geographically between species, wherein prevalence within new zones of sympatry at northern latitudes may be lower than areas where sympatry has occurred for longer periods of time (Wetzel and Weigl, 1994; Pauli et al., 2004; Coombs, 2010; Krichbaum et al., 2010; Espenshade and Stewart, 2013; O’Brien et al., 2022). However, the establishment of S. robustus in novel areas and subsequent effects on NFS populations can happen quickly. For example, Weigl et al. (1992) did not detect S. robustus in NFS in the southern Appalachians until the 1980s, despite all SFS populations examined in the region hosting the nematode during surveys in the 1960s, indicating a recent spillover from SFS to NFS within a 20-year period. The spillover event coincided with a population decline in NFS at one site that has not recovered.
5.2. Hybridization
As species ranges shift due to climate change and anthropogenic land use, interspecific reproduction barriers in novel contact zones may not have been reinforced through evolutionary selection against heterospecifics, increasing the probability that hybridization between formerly parapatric species may occur (Lamont et al., 2003; Chunco, 2014). Hybridization could contribute to reduced fitness and increased extinction probability (Muhlfeld et al., 2009; Kleindorfer et al., 2014), especially of endangered NFS subspecies which already face multiple stressors impacting their populations. Hybridization between resident species and neonatives may help facilitate range expansion of the latter (Pfenning et al., 2016). Understanding hybrid zones between NFS and SFS could help determine the impact of expanding SFS distribution on NFS persistence (Taylor et al., 2015).
Hybridization has been observed between NFS and SFS in Pennsylvania, USA and Ontario (Garroway et al., 2010). Both of the areas with documented hybridization are where SFS has expanded its range due to anthropogenically-induced forest composition change or climate change, (Bowman et al., 2005; Mahan et al., 2010). However, no hybridization events have been documented in the high-elevation spruce-fir southern Appalachian Mountain sky islands. The lack of hybridization events between NFS and SFS in the southern Appalachians may indicate tension zones in areas where parapatric populations have unstable sympatric zones (see Tension zones and habitat permeability section), whereas newly invaded areas with novel sympatry may lead to a higher probability of hybridization events, although further research is needed to confirm this.
Both species prefer conspecific den mates, however, heterospecific denning aggregations have occurred in captivity (Weigl, 1978; Olson et al., 2018) and in the wild (Coombs, 2010). In the recently colonized northern expanses of SFS range, heterospecific denning may increase winter survival of individual SFS, especially since densities of SFS and potential opportunities for conspecific denning aggregations may be lower in newly invaded areas (Garroway et al., 2010; Olson et al., 2018). Since mating in both NFS and SFS occurs in the late winter and spring (Dolan and Carter, 1977; Wells-Gosling and Heaney, 1984) when both species form the largest nesting aggregations, interspecific den sharing may facilitate hybridization.
5.3. Resource competition
In areas of sympatry, competition for resources may lead to aggressive interspecific interactions between flying squirrels, potentially influencing species survival or reproductive success. Resource overlap between NFS and SFS is most likely limited to denning sites since both species consume varied diets and overlap in major food resources is relatively minimal (Dolan and Carter, 1977; Mitchell, 2001). Certain factors may influence availability of den resources, which might result in competition for this resource in areas where SFS are newly sympatric with NFS.
Cavities used by both flying squirrel species are usually found in hardwoods or snags, whereas dreys (i.e., leaf nests) typically occur in conifers (Holloway and Malcolm, 2007; Diggins et al., 2017; O’Brien et al., 2021). Although NFS exhibits flexible use of den sites, including the use of dreys and subterranean dens (e.g., Carey et al., 1997; Diggins et al., 2015), NFS are considered dependent on tree cavities for natal dens as insecure den sites (e.g., subterranean dens) may limit reproductive success (Carey et al., 1997; Smith, 2007). Pregnant female flying squirrels defend natal den trees from conspecifics (Muul, 1968; Smith et al., 2011) and natal dens in nest boxes only contain the mother and her offspring (Weigl et al., 1992; Reynolds et al., 2009). Cavities buffer colder temperatures better compared to dreys (O’Brien et al., 2021) and cavities in live trees buffer colder temperatures better than cavities in snags (Coombs et al., 2010). In the early spring when females establish natal dens, cavities may afford a better thermal buffer from cold temperatures compared to dreys and provide better protection from terrestrial predators than subterranean dens, which could impact the survival of a mother and her dependent young in colder climates, although limited research has focused on denning selection of reproductive females.
Anthropogenic activities, such as forest harvesting, remove larger diameter trees and snags that may serve as cavity trees (Holloway et al., 2007; Vaillancourt et al., 2008), highlighting how land use may limit a resource that historically may have been more common. Areas with lower availability of cavity trees due to land use may lead to increased competition for den sites during certain times of the year. Aggressive competition from SFS could directly impact the success of NFS natal nests, wherein NFS may experience reduced availability of suitable cavities for natal nests, driving them to utilize dreys or subterranean nests instead. Limited cavities in younger forests may also moderate SFS establishment by reducing reproductive success or overwinter survival since SFS are less cold tolerant than NFS (see Thermal Tolerances section). Competition for cavities may also increase interspecific overlap use in nest sites or interspecific nest sharing, which could facilitate S. robustus transmission or hybridization. However, resource competition is difficult to determine in the field, since spatial networks of flying squirrels are most likely complex, flying squirrels use multiple den sites, and nest sharing can be difficult to ascertain unless a large number of individuals or squirrels from nesting aggregations are radio-collared or PIT-tagged (Carey et al., 1997; Garroway et al., 2013; Diggins and Ford, 2021).
6. Factors aiding SFS range expansion
6.1. Anthropogenic alteration of habitat
Within eastern North America, Euro-American (i.e., European colonists that settled in the Americas) activities (i.e., exploitative forest harvesting, shifts in fire regimes) have altered the composition, structure, and distribution of current forests (Foster et al., 1998; Nowacki and Abrams, 2008; Boucher et al., 2009; Danneyrolles et al., 2016). Montane and boreal coniferous species, such as red spruce (Picea rubens), are slow-growing compared to many northern hardwood species. During the industrial clearcut logging period (i.e., 1880s–1940s), hardwood species outcompeted montane conifers in cutover areas, effectively displacing them or causing shifts in overstory dominance at lower elevations and latitudes (Korstian, 1937; Hayes et al., 2007; Boucher et al., 2009). For example, industrial logging in the central Appalachians of West Virginia caused the reduction of red spruce dominant forests from ~600,000 ha prior to logging to ~10,000 ha today (Hopkins, 1899; Griffith and Widmann, 2003). In Quebec, one site showed 56% of coniferous forest was convert to mixed or deciduous forest due to >70 years of logging activities (Boucher et al., 2006), whereas spruce-fir-birch forests dominated the Saguenay River region, but are now confined to high-elevations and steep slopes (Dupuis et al., 2020). Even Great Lakes Region forests that were historically mixedwoods saw significant decreases in conifers like balsam fir (Abies balsamea), eastern white cedar (Thuja occidentalis), and eastern larch (Larix laricina), and increases deciduous species such as maples (Acer spp.) and poplars (Populus spp.) due to logging (Jackson et al., 2000; Schulte et al., 2007; Pinto et al., 2008). This large-scale conversion of conifer-dominant forests and reduction of importance of conifers in mixedwood forests could have led to habitat loss for NFS and increased habitat for SFS across the landscape, allowing SFS to expand their distribution to higher elevations and latitudes.
Forest harvesting may have also increased the permeability of habitat for SFS by aiding in compositional shifts in forests and alteration of habitat patches, wherein forest species composition and distribution was previously driven by elevation, topography, natural disturbance regimes, or climatic factors. While montane and boreal coniferous species associated with NFS habitat are projected to be sensitive to climate change (see Climate Change section), anthropogenic disturbances may accelerate species transitions of conifer-dominant forests to hardwood-dominant forests (Korstian, 1937; Brice et al., 2019). Logging or other types of anthropogenic land use (e.g., roads) may fragment the landscape (Smith and Person, 2007), creating stepping stones or corridors of suitable habitat allowing for SFS to invade NFS habitat at higher elevations or latitudes (Weigl, 2007).
Forest structure can also be impacted by anthropogenic activities (Sturtevant et al., 1997; Ziegler, 2004; Cyr et al., 2009), reducing potential cavity nesting sites via loss of snags or large trees (Carey, 1995). Although cavities used by flying squirrels can occur in hardwood and conifer species, conifers typically need to be larger and older compared to hardwoods before sufficient heart rot can develop natural cavities or facilitate excavation by primary cavity nesters (Bunnell et al., 2002). Density of flying squirrels are typically lower in harvested versus old-growth stands (Taulman et al., 1998; Herbers and Klenner, 2010; Holloway and Smith, 2011), possibly due to reduced denning and altered food resources, although this can vary depending on harvest method used (e.g., clearcut vs. single-tree selection). A reduction in resource availability could increase species competition for these resources, potentially aiding in species turnover.
6.2. Climate-change
Shifts in temperature regimes due to climate change are expected to alter the distributions of species (Chin et al., 2011; Wiens, 2016). Climate change is already associated with range expansion of SFS and range contraction of NFS to more northern latitudes (Myers et al., 2009; Wood et al., 2016). Shifts at the northern edge of SFS geographic range have previously been observed with subsequent contractions or local extirpations of NFS (see Observed Range Shifts section). Increasingly milder winters due to climate change may amplify parasite-mediated competition between NFS and SFS, with negative impacts on the former (see Parasite-Mediation section). Milder winters may also help SFS establish in novel areas, increasing interactions with NFS, heightening the probability of hybridization events and increasing competition for limited resources, such as natal cavity trees.
Although factors, such as microclimate and cloud immersion, may allow the persistence of some montane conifer forests within the southern proportions of NFS range in the Appalachian Mountains, the complete loss of montane conifer habitat due to climate change are projected to occur in some regions within the next century (Burns et al., 2003; Koo et al., 2015). However, anthropogenic disturbance-mediated shifts in warm-climate tree species to higher latitudinal and elevational sites may be amplified by climate change, increasing hardwood species and causing declines in cold-adapted montane and boreal conifers (Iverson et al., 2008; Brice et al., 2019; Boulanger and Puigdevall, 2021). Therefore, a combination of milder winters and vegetation shifts induced by climate change may help SFS establishment farther north, leading to NFS extirpation in the southern latitudes of their range, although the importance of these two factors and how they interact may vary regionally.
7. Factors limiting SFS range expansion
7.1. Thermal tolerances
Flying squirrels have small body sizes and high body temperatures, which may impact their ability to survive cold winters. Although NFS is adapted to colder boreal climates, extremely cold temperatures in the higher latitudes of SFS’s geographic distribution is thought to limit its northern distribution as winter is considered the most critical season to SFS survival (Muul, 1968; Stapp et al., 1991; Thomas and Weigl, 1998). Comparatively, SFS has a higher surface area-to-volume ratio and thinner pelage than NFS (Merritt et al., 2001; Olson et al., 2018), which may influence cold susceptibility between the species (Olson et al., 2017).
Behavioral strategies to deal with colder temperatures include forming winter denning aggregations, reducing foraging time, and torpor (Muul, 1968; Thomas and Weigl, 1998; Nelson and Sagot, 2018). Although flying squirrel exhibit increased body mass during the winter (Stapp, 1992; Weigl et al., 1992; Merritt et al., 2001), extremely cold winter temperatures can cause decreased body weight in individuals (Thomas and Weigl, 1998), indicating availability of particular food resources is needed to survive colder temperatures (see Absence of Resources section). Observations of range expansion in Ontario coincided with warmer winters (Bowman et al., 2005), demonstrating that a warming climate may facilitate range expansions to higher latitudes and elevations.
Social thermoregulation during cold weather may help SFS persist in colder climates. Denning aggregations reduce heat loss during the colder months of the year (Stapp et al., 1991; Merritt et al., 2001). Aggregation size can vary by season with larger non-natal aggregations of individuals typically occurring during the winter months (Stapp et al., 1991; Reynolds et al., 2009; Doty et al., 2022). Pregnant females tend to leave non-natal nesting aggregations in the late winter-early spring and den alone at a new nest site to raise their young (Stapp et al., 1991), so cavity availability for pregnant females may be a limiting factor for successful reproduction in colder regions (see Resource Competition section).
Torpor and reduced activity could help SFS deal with colder temperatures. Torpor is an adaptive behavior used to conserve energy during colder periods (Lyman et al., 1982). Some anecdotal evidence of torpor has occurred in field observations for SFS (Muul, 1968), but only limited evidence of torpor was observed for both species in laboratory settings (Olson et al., 2017). Activity of radio-collared NFS have occurred during subfreezing winter temperatures (Cotton and Parker, 2000; Ford et al., 2014), highlighting their adaptation to very cold temperatures. Although SFS are known to reduce foraging activities during cold temperatures (Thomas and Weigl, 1998), there are no studies that describe SFS winter activity patterns associated with temperature in the northern limits of their range. Colder temperatures at the northern reaches of SFS geographic range may hinder winter activity patterns, including suitable conditions for foraging, although this needs to be further explored.
Whereas colder temperatures are limiting factors for SFS, higher temperatures are not limiting factors for NFS, indicating that warmer temperatures at the southern extremes of their range may not drive range contractions in this species (Gudde, 2022). Other factors, such as shifts in the distribution of NFS preferred habitat to higher elevations and latitudes, may have narrowed the thermal envelopes where we currently observe NFS occurring as this species previously experienced contractions in some parts of their range unrelated to climate change. However, shifts in habitat may have also expanded the thermal envelope where SFS are found via land use shifts of suitable SFS habitat to higher elevations and latitudes (see Tension Zones and Habitat Permeability section). This would indicate that species turnover in areas of sympatry may not solely be driven by the direct effects of a warming climate, but the effects of a warming climate interacting with anthropogenic alterations of available resources. However, in some areas, such as Ontario, pre-industrial forests may have been suitable habitat for SFS, but thermal conditions may have restricted SFS from expanding into these sites until more recently. Therefore, climatic warming could explain why SFS has exhibited large latitudinal range shifts over short periods of time in this region as well as the partial range collapses of the SFS invasion front due to the impacts of cold winters (Bowman et al., 2005).
7.2. Absence of resources
The distribution of SFS may be limited by resource requirements (Weigl, 1978). Reduced thermal tolerance by SFS in colder climates could partially depend on the availability of resources in the northern parts of their range. Resources utilized by SFS to deal with colder winters include caching nuts and preferential use of cavities over dreys (Muul, 1968; Thomas and Weigl, 1998; O’Brien et al., 2021).
Hard mast is an important high-energy winter food source that SFS store in caches and access throughout the winter (Thomas and Weigl, 1998; Helmick et al., 2014). For example, hickory nuts are considered an important winter food for SFS (Muul, 1968; Thomas and Weigl, 1998). However, most hickory species do not occur in the northern limits of SFS range, including areas with recent range expansions. New records indicating SFS range expansion or species turnover were observed in deciduous or mixedwood forests (Bowman et al., 2005; Lazure et al., 2016; Wood et al., 2016), implying suitable habitat is most likely still needed to meet food requirements. With climate change, oaks, hickories, and American beech are predicted to expand their ranges >100–250 km northward depending on the species, although it may take centuries for these shifts to occur (Iverson and Prasad, 1998). The presence of American beech may be especially important for SFS in areas where oaks and hickories do not occur (i.e., northern latitudes, higher elevations), potentially influencing expansion into new areas (Garroway et al., 2011).
Despite their large geographic ranges, NFS and SFS may be considered habitat specialists since specific resources associated with their habitats allow for their persistence (Weigl, 2007). Observations of range expansion and establishment of SFS typically occur with high mast years coinciding with warm winters (Bowman et al., 2005; Wood et al., 2016). Even if climate change increases the occurrence of mild winters, a lack of mast availability in an area newly invaded by SFS might still hinder their winter survival and establishment at those sites. For example, the range expansion of SFS in Ontario linked to warmer winters was followed by a subsequent range contraction of 240 km associated with a mast crop failure directly followed by a colder winter (Bowman et al., 2005). This event indicates the availability of hard mast may be important to the persistence of SFS in newly colonized areas during latitudinal shifts in the northern parts of its range. However, seasonal shifts to higher elevations in the Appalachians may occur dynamically without range shifts of mast-producing tree species, since SFS can retreat a couple hundred meters downhill to access those resources. Until climate change causes shifts in forest species composition, wherein southern mast tree species expand their ranges to more northern latitudes, SFS may lack food resources to successfully establish populations at northern latitudes where hard mast species do not occur, although this will probably vary regionally.
Denning resources have been hypothesized to be a limiting factor for flying squirrels (Carey et al., 1997), although this may only be true in certain parts of a species’ range or where forest structure has been drastically altered (i.e., logged forests vs. old-growth). Both species exhibit flexibility in utilization of denning resources, using tree cavities, dreys, and subterranean dens (Muul, 1968; Campuano-Chávez-Peón et al., 2014; Diggins et al., 2015). However, SFS are thought to be more limited in their den selection compared to NFS, although this may be geographically dependent with SFS exhibiting a higher reliance on cavities in more northern parts of their range (O’Brien et al., 2021) and more denning flexibility in southern parts of their range (Campuano-Chávez-Peón et al., 2014). Since cavities in live trees buffer cold temperatures better than dreys or cavities in snags (Coombs et al., 2010; O’Brien et al., 2021) and larger cavity trees influence winter nesting aggregation formation because large diameter trees tend to have cavities compared to small diameter trees (Bunnell et al., 2002; Jacques et al., 2017), presence of large cavity trees and snags may be important for SFS winter survival. The logging history of a stand may influence the availability of these cavity trees (Holloway et al., 2007; Vaillancourt et al., 2008), making den tree availability a factor in the successful expansion of SFS’s range.
7.3. Tension zones and habitat permeability
Areas where parapatric species may be more resilient to invasions are tension zones. In hybridization areas, tension zones are considered areas that are perpetuated by an equilibrium between dispersal of and selection against hybrids (Barton and Hewitt, 1989). The concept of a tension zone can be more widely applied to encompass factors that influence that equilibrium, including resources and interspecific interactions. In areas of historically unstable sympatry, tension zones may help prevent the expansion of a neonative, especially in areas where interspecific interactions are not novel. For example, in the southern Appalachians, the spruce-northern hardwood ecotone acts as a tension zone where unstable sympatry between lower elevation populations of SFS and higher elevation populations of NFS occur (Weigl et al., 1992). This tension zone is relatively narrow (50–250 m wide) and habitat attributes suitable to both species occur in this zone. However, SFS lack suitable resources above the tension zone, whereas NFS lack suitable resources below it. For example, summer observations of SFS have occasionally occurred in red spruce forests near the spruce-northern hardwood ecotone (Urban, 1988), but SFS do not persist in these forests due to lack of food resources. Therefore, tension zones may allow more resistance to hybridization and have more restricted invasion fronts compared to novel zones of sympatry where interspecific interactions between SFS and NFS do not have precedence.
Species extirpations may be lower along elevational vs. latitudinal gradients (Wiens, 2016). Eastern hemlock (Tsuga canadensis)-dominated stands preferred by NFS in Pennsylvania are small and extremely fragmented due to historic logging and forest declines via hemlock woolly adelgid (Adelges tsugae). NFS habitat in Pennsylvania occurs across similar elevational gradients as SFS habitat in the state (Mahan et al., 2010), whereas habitat in the southern Appalachians is higher quality and strongly elevationally segregated across steep mountainous terrain (Ford et al., 2015; Diggins et al., 2017). Observations during long-term nest box monitoring and acoustic surveys only show SFS detected in hardwood-dominant forests or right along the northern hardwood-spruce ecotone, but SFS detections in the montane spruce-fir forests are extremely rare and typically only observed during the warmer months (Diggins et al., 2020a). However, SFS is present at many sites with NFS in Pennsylvania, and historic NFS sites exhibited species turnover in hemlock and mixedwood stands (Mahan et al., 1999; Diggins et al., 2020b). The lack of elevational segregation of NFS habitat from SFS habitat may make habitat permeability (i.e., the ability of neonatives to disperse into a novel habitat) greater in Pennsylvania than North Carolina, despite the latter sites occurring 700 km south of Pennsylvania.
Historic species distribution prior to industrial logging is also important to note when considering factors influencing range expansion and potential tension zones. With projected climate change, future habitat suitability and resiliency is underestimated if historical distribution data is ignored since current species distributions may represent more narrow bioclimatic envelopes than distributions that existed prior to Euro-American disturbance (Andrews et al., 2022). For example, in the southern Appalachians, exploitative harvesting led to an uphill contraction of spruce-fir forests by approximately a minimum of 200 m (Hayes et al., 2007) due to the competitive advantage faster-growing northern hardwood species had over slow-growing red spruce in clearcut stands (Korstian, 1937; Pyle and Schafale, 1988). It is reasonable to postulate if red spruce occurred at lower elevations, then NFS dependent on these forests (Ford et al., 2015; Diggins et al., 2017) may also have experienced upward range contractions after the industrial logging period. Therefore, the thermal tolerances of NFS in the southern Appalachians may be wider than their current distribution suggests. On the other hand, SFS experienced an upward range expansion as a result of landscape disturbance, potentially placing them closer to their thermal limits within the southern Appalachians. The anthropogenically-induced upward contraction of habitat and strong elevational segregation of NFS and SFS habitat could explain why there have been no observations of SFS invading NFS habitat and establishing year-round residency at the higher elevations despite long-term nest box monitoring occurring across in the southern Appalachians since the 1980s–1990s. In some forests around the Great Lakes Region, past logging increased deciduous species and reduced coniferous species (Jackson et al., 2000; Schulte et al., 2007; Pinto et al., 2008), potentially providing more suitable habitat for SFS. However, many forests in the western Great Lakes were historically mixedwood (Jackson et al., 2000; Pinto et al., 2008), so the impact of historical logging on habitat suitability for SFS may have been minimal compared to the Appalachians and eastern Canada. Additionally, recent observations of range expansions of SFS in the region may highlight that despite available habitat, thermal intolerances may have limited SFS expansion to those areas until the last several decades, indicating climate change is most likely responsible for range shifts in mixedwood forests around the Great Lakes Region. Since the northern range extent of SFS prior to historical logging is unknown, assumptions that areas of sympatry between SFS and NFS as long-term and stable could be misleading.
8. Conclusion
Species ranges are naturally dynamic and shift depending on environmental conditions over time. Overlaps between the ranges of sister species are also dynamic and natural disturbance events (e.g., stand-replacing fire, insect outbreaks) may potentially lead to shifts in secondary contact zones at smaller scales. However, historical logging and other anthropogenic activities (i.e., fire suppression) within North American occurred over large scales, most forests have been historically logged causing local to regional shifts in tree community composition and structure, and the majority of forests are second-growth stands <80 years old (Thomas et al., 1988; Davis et al., 1996; Noss et al., 2006; Nowacki and Abrams, 2008; DellaSala et al., 2022). Anthropogenic-induced shifts in wildlife species ranges due to interactions between land-use alterations in forest composition and climate change present a conservation challenge for species that are taxonomically similar, but may have had limited sympatry due to habitat specialization. Although these range shifts could be potential conservation concerns (Essl et al., 2019), they are rarely assessed as possible invasion fronts. Additionally, factors influencing species range shifts are usually thought to be associated with climatic changes and not historical land use, although the interaction of both likely contribute to species range shifts in most cases (e.g., Rowe, 2007; Wan et al., 2022). Since historical land use may restrict or provide resources that enable SFS to invade and maintain newly expanded parts of their range in a warming climate, understanding how land use interacts with climate change in influencing SFS range shifts is important for predicting effects on NFS.
Factors aiding and mitigating SFS invasion of NFS habitat are dynamic and interacting. Range shifts will most likely not be homogeneous across geographic gradients of a species even at range edges, especially when factors (e.g., historic land use, invasive forest pests) interact with temperature, producing a range of potential conditions that drive variation in species distribution shifts (Peel et al., 2017). Within areas of sympatry, hybridization, parasite-mediation, and resource competition may contribute to species turnover from NFS to SFS, although how long species turnover may take to occur may be dependent on factors at local or regional scales. Some areas could also result in stable sympatry between the species, although long-term research over multiple decades would be needed to evaluate this.
Anthropogenic land use and climate change are two major factors that may aid in the range expansion of SFS, which can influence local or regional dynamics affecting species turnover. Factors that could limit SFS range expansion include cold tolerance limits of SFS, absence of denning and food resources that improve survival in colder climates, and tension zones that stall SFS range expansion. While climate change is predicted to expand the range of SFS to higher elevations and latitudes, the interaction between past land use and availability of resources may be critical to the long-term establishment of SFS. Additionally, areas with recent establishment of SFS may have had suitable habitat prior to industrial logging or due to logging-induced forest composition shifts, but previous SFS establishment was probably hindered until climatic conditions became more suitable in more recent decades.
Although species turnover can happen in relatively short time periods in novel areas of sympatry (Myers et al., 2009; Wood et al., 2016; O’Brien et al., 2022), SFS persistence at a site may be important to facilitate that turnover. Invasion fronts can be dynamic and not all peripheral parts of a resident species’ range will face the same vulnerability of invasion depending on factors influencing tension zones between neonative and resident species. In the case of NFS, disjunct populations at the most southern Appalachians may be less susceptible to SFS invasion compared to populations in northern Appalachians and Great Lakes Region due to previous logging-induced elevational habitat shifts and the presence of more contiguous habitat patches in the southern Appalachians which limit further SFS expansion uphill, as well as current land management practices (i.e., lack of logging, active spruce restoration).
In areas where SFS range expansion may drive extirpation of NFS, especially of endangered subspecies, land management and ecological restoration may halt or weaken the SFS invasion front. For example, red spruce restoration in the central and southern Appalachians aims to increase the historic extent of spruce-fir forests by increasing conifer-dominant stands and promoting old-growth structure and decadence that could improve resource availability for NFS (Rentch et al., 2007, 2016). Restoration efforts aim to increase resilience of montane conifer forests to climate change and provide long-term habitat for endangered subspecies of NFS. Further work is needed to understand which NFS populations are vulnerable to SFS range expansion, which could help prioritize restoration efforts in these areas.
Author contributions
The author confirms being the sole contributor of this work and has approved it for publication.
Funding
Funding for the publication of this paper was provided by the Virginia Tech Open Access Subvention Fund.
Acknowledgments
I would like to thank the editors of this special research topic, Valentina La Morgia, Maria Mazzamuto, and Tim Adriaens, for inviting me to submit this paper. Mark Ford and the reviewers provided insightful comments that improved the manuscript. Figure 2 is published with BioRender Agreement Number BT23ZNL06O.
Conflict of interest
The author declares that the research was conducted in the absence of any commercial or financial relationships that could be construed as a potential conflict of interest.
Publisher’s note
All claims expressed in this article are solely those of the authors and do not necessarily represent those of their affiliated organizations, or those of the publisher, the editors and the reviewers. Any product that may be evaluated in this article, or claim that may be made by its manufacturer, is not guaranteed or endorsed by the publisher.
References
Andrews, C., Foster, J. R., Weiskitte, A., D’Amato, A. W., and Simons-Legaard, E. (2022). Integrating historical observations alters projections of eastern North American spruce-fir habitat under climate change. Ecosphere 13:e 4016. doi: 10.1002/ecs2.4016
Bartlett, C. M. (1995). Morphology, homogonic development, and lack of a free-living generation in Strongyloides robustus (Nematoda, Rhabditoidea), a parasite of North American sciurids. Folia Parasitol. 42, 102–114.
Barton, N. H., and Hewitt, G. M. (1989). Adaptation, speciation and hybrid zones. Nature 341, 497–503. doi: 10.1038/341497a0
Bellard, C., Genovesi, P., and Jeschke, J. M. (2016). Global patterns in threats to vertebrates by biological invasions. Proc. R. Soc. B 283:20152454. doi: 10.1098/rspb.2015.2454
Bendel, P. R., and Gates, J. E. (1987). Home range and microhabitat portioning of the southern flying squirrel (Glaucomys volans). J. Mammal. 68, 243–255. doi: 10.2307/1381463
Bertolino, S., di Montezmolo, N. C., Preatoni, D. G., Wauters, L. A., and Martinoli, A. (2014). A grey future for Europe: Sciurus carolinensis is replacing native red squirrels in Italy. Biol. Invasions 16, 53–62. doi: 10.1007/s10530-013-0502-3
Bleich, V. C., and Price, M. V. (1995). Aggressive behavior of Dipodomys stephensi, and endangered species, and Dipodomys agilis, a sympatric congener. J. Mammal. 76, 646–651. doi: 10.2307/1382373
Boucher, Y., Arseneault, D., and Sirois, L. (2006). Logging-induced change (1930-2002) of a preindustrial landscape at the northern range limit of northern hardwoods, eastern Canada. Can. J. For. Res. 2, 505–517. doi: 10.1139/x05-252
Boucher, Y., Arseneault, D., Sirois, L., and Blais, L. (2009). Logging pattern and landscape changes over the last century in boreal and deciduous forest transition in eastern Canada. Landsc. Ecol. 24, 171–184. doi: 10.1007/s10980-008-9294-8
Boulanger, Y., and Puigdevall, J. P. (2021). Boreal forests will be more severely affected by projected anthropogenic climate forcing than mixedwood and northern hardwood forests in eastern Canada. Landsc. Ecol. 36, 1725–1740. doi: 10.1007/s10980-021-01241-7
Bowman, J., Holloway, G. L., Malcolm, J. R., Middel, K. R., and Wilson, P. J. (2005). Northern range boundary dynamics of southern flying squirrels: evidence of an energetic bottleneck. Can. J. Zool. 83, 1486–1494. doi: 10.1139/z05-144
Brice, M. H., Cazelles, K., Legendre, P., and Fortin, M. J. (2019). Disturbances amplify tree community responses to climate change in the temperate-boreal ecotone. Glob. Ecol. Biogeogr. 11, 1668–1681. doi: 10.1111/geb.12971
Bunnell, F. L., Wind, E., Boyland, M., and Houde, I. (2002) Diameters and heights of trees with cavities: Their implications to management. General Technical Report PSW-GTR-181. U.S. Department of Agriculture, Forest Service. pp. 717–737.
Burns, C. E., Johnston, K. M., and Schmitz, O. J. (2003). Global climate change and mammalian species diversity in US national parks. Proc. Nat. Acad. Sci. 100, 11474–11477. doi: 10.1073/pnas.1635115100
Campuano-Chávez-Peón, D., Zuria, I., Castellanos, I., and Gates, J. E. (2014). Characteristics of nest-sites of the southern flying squirrel (Glaucomys volans) in a pine-oak forest of Central Mexico. Southwest. Nat. 59, 75–80. doi: 10.1894/F10-JKF-38.1
Carey, A. B. (1995). Sciurids in Pacific Northwest managed and old-growth forests. Ecol. Appl. 5, 648–661. doi: 10.2307/1941974
Carey, A. B., Wilson, T. M., Maguire, C. C., and Biswell, B. L. (1997). Dens of northern flying squirrels in the Pacific Northwest. J. Wildl. Manag. 61, 684–699. doi: 10.2307/3802176
Carlson, C. J., Burgio, K. R., Dougherty, E. R., Phillips, A. J., Bueno, V. M., Clements, C. F., et al. (2017). Parasite biodiversity faces extinction and redistribution in a changing climate. Sci. Adv. 3:e1602422. doi: 10.1126/sciadv.1602422
Chappell, M. A. (1978). Behavioral factors in the altitudinal zonation of chipmunks (Eutamias). Ecology 59, 565–579. doi: 10.2307/1936586
Chin, I. C., Hill, J. K., Ohlemüller, R., Roy, D. B., and Thomas, C. D. (2011). Rapid range shifts of species associated with high levels of climate warming. Science 333, 1024–1026. doi: 10.1126/science.1206432
Chunco, A. J. (2014). Hybridization in a warmer world. Ecol. Evol. 4, 2019–2031. doi: 10.1002/ece3.1052
Coombs, A. B. (2010) Competition between parapatric flying squirrel species: nest use, parasitism, and the implications of climate change. M.S. thesis, Trent University, Peterborough, Ontario, Canada.
Coombs, A. B., Bowman, J., and Garroway, C. J. (2010). Thermal properties of tree cavities during winter in a northern hardwood forest. J. Wildl. Manag. 74, 1875–1881. doi: 10.2193/2009-560
Cotton, C. L., and Parker, K. L. (2000). Winter activity patterns of northern flying squirrels in sub-boreal forests. Can. J. Zool. 78, 1896–1901. doi: 10.1139/z00-137
Cyr, D., Gauthier, S., Bergeron, Y., and Carcaillet, C. (2009). Forest management is driving the eastern North American boreal forest outside its natural range of variability. Front. Ecol. Environ. 7, 519–524. doi: 10.1890/080088
Danneyrolles, V., Aresenault, D., and Bergeron, Y. (2016). Pre-industrial landscape composition patterns and post-industrial changes at the temperate-boreal forest interface in western Quebec, Canada. J. Veg. Sci. 27, 470–481. doi: 10.1111/jvs.12373
Davis, M. D., Comers, S., Schaadt, C., Cook, A., Lynch, M., Seaton, K., White, R., Meier, A., Farrari, J., Means, B., and Runkle, J. (1996) Eastern old-growth forests: Prospects for rediscovery and recovery. Washington, D.C.: Island Press.
DellaSala, D. A., Mackey, B., Norman, P., Campbell, C., Comer, P. J., Kormos, C. F., et al. (2022). Mature and old-growth forests contribute to large-scale conservation targets in the conterminous United States. Front. For. Glob. Change 28:209. doi: 10.3389/ffgc.2022.979528
Diggins, C. A. (2021). Behaviors associated with vocal communication of squirrels. Ecosphere 12:e03572. doi: 10.1002/ecs2.3572
Diggins, C. A., and Ford, W. M. (2021). Spatial behavior of northern flying squirrels in the same social network. Ethology 127, 424–432. doi: 10.1111/eth.13130
Diggins, C. A., Gilley, L. M., Kelly, C. A., and Ford, W. M. (2020a). Using ultrasonic acoustics to detect cryptic flying squirrels: effects of season and habitat quality. Wildl. Soc. Bull. 44, 300–308. doi: 10.1002/wsb.1083
Diggins, C. A., Gilley, L. M., Turner, G. G., and Ford, W. M. (2020b). Ultrasonic acoustic surveys of state endangered northern flying squirrels in the Pocono Mountains, Pennsylvania. J. Fish Wildl. Manag. 11, 644–653. doi: 10.3996/JFWM-20-020
Diggins, C. A., Kelly, C. A., and Ford, W. M. (2015). Atypical den use of Carolina northern flying squirrels (Glaucomys sabrinus coloratus) in the southern Appalachian Mountains. Southeast. Nat. 14, N44–N49. doi: 10.1656/058.014.0303
Diggins, C. A., Silvis, A., Kelly, C. A., and Ford, W. M. (2017). Home range, den selection, and habitat use of Carolina northern flying squirrels (Glaucomys sabrinus coloratus). Wildl. Res. 44, 427–437. doi: 10.1071/WR16203
Doty, A. C., Connioe, M. B., and Risch, R. S. (2022). Drivers of southern flying squirrel (Glaucomys volans) aggregation size in South Carolina, U.S.A. Am. Midl. Nat. 188, 20–32. doi: 10.1674/0003-0031-188.1.20
Dupuis, S., Danneyrolles, V., Laflamme, J., Boucher, Y., and Arseneault, D. (2020). Forest transformation following European settlement in the Saguenay-Lac-St-Jean Valley in Eastern Québec, Canada. Front. Ecol. Evol. 8:257. doi: 10.3389/fevo.2020.00257
Elmhagen, B., Berteaux, D., Burgess, R. M., Ehrich, D., Gallant, D., Henttonen, H., et al. (2017). Homage to Hersteinsson and Macdonald: climate warming and resource subsidies cause red fox range expansion and Arctic fox decline. Polar Res. 36:sup1. doi: 10.1080/17518369.2017.1319109
Espenshade, J. L., and Stewart, R. L. (2013). Prevalence of Strongyloides robustus in tree squirrels (Sciuridae) in south-Central Pennsylvania and potential impacts for the endangered northern flying squirrel, Glaucomys sabrinus. J. Stud. Res. 2, 43–47. doi: 10.47611/jsr.v2i1.141
Essl, F., Dullinger, S., Genovesi, P., Hulme, P. E., Jeschke, J. M., Katsanevakis, S., et al. (2019). A conceptual framework for range-expanding species that track human-induced environmental change. Bioscience 69, 908–919. doi: 10.1093/biosci/biz101
Fleming, P. A., Anderson, H., Prendergast, A. S., Bretz, M. R., Valentine, L. E., and Hardy, G. E. S. (2013). Is the loss of Australian digging mammals contributing to a deterioration in ecosystem function? Mammal Rev. 44, 94–108. doi: 10.1111/mam.12014
Ford, W. M., Evans, A. M., Odom, R. H., Rodrigue, J. L., Kelly, C. A., Abaid, N., et al. (2015). Predictive habitat models derived from nest-box occupancy for the endangered Carolina northern flying squirrel in the southern Appalachians. Endang. Spp. Res. 27, 131–140. doi: 10.3354/esr00662
Ford, W. M., Kelly, C. A., Rodrigue, J. L., Odom, R. H., Newcomb, D., Gilley, L. M., et al. (2014). Late winter and early spring home range and habitat use of the endangered Carolina northern flying squirrel in western North Carolina. Endang. Spp. Res. 23, 73–82. doi: 10.3354/esr00561
Foster, D. R., Motzkin, G., and Slater, B. (1998). Land-use history as long-term broad-scale disturbance: regional forest dynamics in Central New England. Ecosystems 1, 96–119. doi: 10.1007/s100219900008
Garroway, C. J., Bowman, J., Cascaden, T. J., Holloway, G. L., Mahan, C. G., Malcolm, J. R., et al. (2010). Climate change induce hybridization in flying squirrels. Glob. Chang. Biol. 16, 113–121. doi: 10.1111/j.1365-2486.2009.01948.x
Garroway, C. J., Bowman, J., Holloway, G. L., Malcolm, J. R., and Wilson, P. J. (2011). The genetic signature of rapid range expansion by flying squirrels in response to contemporary climate warming. Glob. Chang. Biol. 17, 1760–1769. doi: 10.1111/j.1365-2486.2010.02384.x
Garroway, C. J., Bowman, J., and Wilson, P. J. (2013). Complex social structure of southern flying squirrels is related to spatial proximity but not kinship. Behav. Ecol. Sociobiol. 67, 113–122. doi: 10.1007/s00265-012-1431-3
Gilley, L. M., Diggins, C. A., Pearson, S. M., and Best, T. L. (2019). Vocal repertoire of captive northern and southern flying squirrels (Glaucomys sabrinus and G. volans). J. Mammal. 100, 518–530. doi: 10.1093/jmammal/gyz064
Gilmore, R. M., and Gates, J. E. (1985). Habitat use by the southern flying squirrel at a hemlock-northern hardwood ecotone. J. Wildl. Manag. 49, 703–710. doi: 10.2307/3801699
Gómez, J. M., González-Megías, A., Lorite, J., Abdelaziz, M., and Perfectti, F. (2015). The silent extinction: climate change and the potential hybridization-mediated extinction of endemic high-mountain plants. Biodivers. Conserv. 24, 1843–1857. doi: 10.1007/s10531-015-0909-5
Griffith, D. M., and Widmann, R. H. (2003) Forest statistics for West Virginia: 1989 and 2000. Resource bulletin NE-157, U.S. Department of Agriculture, Forest Service, northeastern Research Station, Newtown Square, Pennsylvania, USA.
Gudde, E. (2022) The effects of rising ambient temperatures on thermoregulation and range shifts of northern flying squirrels. M.S. Thesis, University of Maine, Bangor.
Gurnell, J., Wauters, L. A., Lurz, P. W. W., and Tosi, G. (2004). Alien species and interspecific competition: effects of introduced eastern grey squirrels on red squirrel population dynamics. J. Anim. Ecol. 73, 26–35. doi: 10.1111/j.1365-2656.2004.00791.x
Hayes, M., Moody, A., White, P. S., and Costanza, J. L. (2007). The influence of logging and topography on the distribution of spruce-fir forests near their southern limits in the Great Smoky Mountains National Park, USA. Plant Ecol. 189, 59–70. doi: 10.1007/s11258-006-9166-8
Helmick, K. R., Barrett, T. L., and Barrett, G. W. (2014). Dietary resource preference of the southern flying squirrel (Glaucomys volans). Am. Midl. Nat. 171, 371–374. doi: 10.1674/0003-0031-171.2.371
Herbers, J., and Klenner, W. (2010). Effects of logging pattern and intensity on squirrel demography. J. Wildl. Manag. 71, 2655–2663. doi: 10.2193/2004-320
HillRisLambers, J., Harsch, M. A., Ettinger, A. K., Ford, K. R., and Theobald, E. J. (2013). How will biotic interactions influence climate change-induced range shifts? Ann. N. Y. Acad. Sci. 1297, 112–125. doi: 10.1111/nyas.12182
Holloway, G. L., Caspersen, J. P., Vanderwel, M. C., and Naylor, B. J. (2007). Cavity tree occurrence in hardwood forests of Central Ontario. For. Ecol. Manag. 239, 191–199. doi: 10.1016/j.foreco.2006.12.004
Holloway, G. L., and Malcolm, J. R. (2007). Northern and southern flying squirrel use of space within home ranges in Central Ontario. For. Ecol. Manag. 242, 747–755. doi: 10.1016/j.foreco.2007.02.020
Holloway, G. L., and Smith, W. P. (2011). A meta-analysis of forest age and structure effects on northern flying squirrel densities. J. Wildl. Manag. 75, 668–674. doi: 10.1002/jwmg.77
Holm, S. R., Noon, B. R., Wiens, J. D., and Ripple, W. J. (2016). Potential trophic cascades triggered by the barred owl range expansion. Wildl. Soc. Bull. 40, 615–624. doi: 10.1002/wsb.714
Hopkins, A. D. (1899) Report on investigations to determine the cause of unhealthy conditions of the spruce and pine from 1880–1893. Bulletin 56, West Virginia Agricultural Experiment Station, Morgantown, WV, USA.
Hough, M. J., and Dieter, C. D. (2009). Resource selection habitat model of northern flying squirrels in the Black Hills, South Dakota. Am. Midl. Nat. 162, 356–372. doi: 10.1674/0003-0031-162.2.356
Iverson, L. R., and Prasad, A. M. (1998). Predicting abundance of 80 tree species following climate change in the eastern United States. Ecol. Monogr. 68, 465–485. doi: 10.1890/0012-9615(1998)068[0465:PAOTSF]2.0.CO;2
Iverson, L. R., Prasad, A. M., Matthews, S. N., and Peters, M. (2008). Estimating potential habitat for 134 eastern U.S. tree species under six climate scenarios. Ecol. Manag. 254, 390–406. doi: 10.1016/j.foreco.2007.07.023
Jackson, S. M., Pinto, F., Malcolm, J. R., and Wilson, E. R. (2000). A comparison of pre-European settlement (1857) and current (1981-1995) forest composition in Central Ontario. Can. J. For. Res. 30, 605–612. doi: 10.1139/x99-242
Jacques, C. N., Zweep, J. S., Jenkins, S. E., and Klaver, R. W. (2017). Home range use and survival of southern flying squirrels in fragmented forest landscapes. J. Mammal. 98, 1479–1488. doi: 10.1093/jmammal/gyx089
Kelly, E. G., Forsman, E. D., and Anthony, R. G. (2003). Are barred owls displacing spotted owls? Condor 105, 45–53. doi: 10.1093/condor/105.1.45
Kelt, D. A., Sollmann, R., White, A. M., Roberts, S. L., and Van Vuren, D. H. (2017). Diversity of small mammals in the Sierra Nevada: filtering by natural selection or by anthropogenic activities? J. Mammal. 98, 85–93. doi: 10.1093/jmammal/gyw158
Kleindorfer, S., O’Connor, J. A., Dudaniec, R. Y., Myers, S. A., Robertson, J., and Sulloway, F. J. (2014). Species collapse via hybridization in Darwin’s tree finches. Am. Nat. 183, 325–341. doi: 10.1086/674899
Koo, K. A., Patten, B. C., and Madden, M. (2015). Predicting effects of climate change on habitat suitability of red spruce (Picea rubens Sarg.) in the southern Appalachian Mountains of the USA: understanding complex systems mechanisms through modeling. Forests 6, 1208–1226. doi: 10.3390/f6041208
Korstian, C. F. (1937). Perpetuation of spruce on cut-over and burned lands in the higher southern Appalachian Mountains. Ecol. Monogr. 7, 125–167. doi: 10.2307/1943304
Krichbaum, K., Mahan, C. G., Steele, M. A., Turner, G., and Hudson, P. J. (2010). The potential role of Strongyloides robustus on parasite-mediated competition between two species of flying squirrel (Glaucomys). J. Wildl. Dis. 46, 229–235. doi: 10.7589/0090-3558-46.1.229
Lamont, B. B., He, T., Enright, N. J., Krauss, S. L., and Miller, B. P. (2003). Anthropogenic disturbance promotes hybridization between Banksia species altering their biology. J. Evol. Biol. 16, 551–557. doi: 10.1046/j.1420-9101.2003.00548.x
Layne, J. N., and Raymond, M. A. V. (1994). Communal nesting of southern flying squirrels in Florida. J. Mammal. 75, 110–120. doi: 10.2307/1382242
Lazure, L., Paré, P., Tessier, N., Bourgault, P., Dubois, G., Canac-Marquis, P., et al. (2016). Known range expansion and morphological variation in the southern flying squirrel (Glaucomys volans) in Quebec. Can. Field-Nat. 130, 216–221. doi: 10.22621/cfn.v130i3.1883
Lyman, C. P., Willis, J. S., Malan, A., and Wang, L. C. H. (1982) Hibernation and torpor in mammals and birds. New York: Academic Press.
M’Closkey, R. T. (1978). Niche separation and assembly in four species of Sonoran Desert rodents. Am. Nat. 112, 683–694.
Mahan, C. G., Bishop, J. A., Steele, M. A., Turner, G., and Myers, W. L. (2010). Habitat characteristics and revised gap landscape analysis for the northern flying squirrel (Glaucomys sabrinus), a state endangered species in Pennsylvania. Am. Midl. Nat. 164, 283–295. doi: 10.1674/0003-0031-164.2.283
Mahan, C. G., and Steele, M. A. (2022). “The potential role of nematode parasites in wildlife decline – Evidence from Allegheny woodrats (Neotoma magister), northern flying squirrels (Glaucomys sabrinus) and now the Eurasian red squirrel (Sciurus vulgaris)” in Parasitic Helminths and Zoonoses - from basic to applied research. eds. J. Morales-Montor, V. Río-Araiza, and R. Hernandéz-Bello (London, United Kingdom: IntechOpen)
Mahan, C. G., Steele, M. A., Patrick, M. J., and Kirkland, G. L. (1999). The status of the northern flying squirrel (Glaucomys sabrinus) in Pennsylvania. J. Penn. Acad. Sci. 73, 15–21.
Mank, J. E., Carlson, J. E., and Brittingham, M. C. (2004). A century of hybridization: decreasing genetic distance between American black ducks and mallards. Conserv. Genet. 5, 395–403. doi: 10.1023/B:COGE.0000031139.55389.b1
McCain, C. M., and King, S. R. B. (2014). Body size and activity times mediate mammalian responses to climate change. Glob. Chang. Biol. 20, 1760–1769. doi: 10.1111/gcb.12499
McCay, T. S., Lovallo, M. J., Ford, W. M., and Menzel, M. A. (2004). Assembly rules for functional groups of North American shrews: effects of geographic range and habitat partitioning. Oikos 107, 141–147. doi: 10.1111/j.0030-1299.2004.13244.x
Merritt, J. A., Zegers, D. A., and Rose, L. R. (2001). Seasonal thermogenesis of southern flying squirrels (Glaucomys volans). J. Mammal. 82, 51–64. doi: 10.1644/1545-1542(2001)082<0051:STOSFS>2.0.CO;2
Miller, T. E. X., Angert, A. L., Brown, C. D., Lee-Yaw, J. A., Lewis, M., Lutscher, F., et al. (2020). Eco-evolutionary dynamics of range expansion. Ecology 101:e03139. doi: 10.1002/ecy.3139
Mitchell, D. (2001). Spring and fall diet of the endangered West Virginia northern flying squirrel (Glaucomys sabrinus fuscus). Am. Midl. Nat. 146, 439–443. doi: 10.1674/0003-0031(2001)146[0439:SAFDOT]2.0.CO;2
Mortiz, C., Patton, J. L., Conroy, C. J., Parra, J. L., White, G. C., and Bessinger, S. R. (2008). Impact of a century of climate change on small-mammal communities in Yosemite National Park, USA. Science 322, 261–264. doi: 10.1126/science.1163428
Muhlfeld, C. C., Kalinowski, S. T., McMahon, T. E., Taper, M. L., Painter, S., Leary, R. F., et al. (2009). Hybridization rapidly reduced fitness of a native trout in the wild. Biol. Lett. 5, 328–331. doi: 10.1098/rsbl.2009.0033
Murúa, R., and González, L. A. (1982). Microhabitat selection in two Chilean cricetid rodents. Oecologia 52, 12–15. doi: 10.1007/BF00349005
Mutze, G., Cooke, B., and Jennings, S. (2016). Estimating density-dependent impacts of European rabbits on Australian tree and shrub populations. Austral. J. Botany 64, 142–152. doi: 10.1071/BT15208
Muul, I. (1968) Behavioral and physiological influences on the distribution of the flying squirrel, Glaucomys volans. Miscellaneous Publications No. 134, Museum of Zoology, University of Michigan, Ann Arbor.
Myers, P., Lundrigan, B. L., Hoffman, S. M. G., Haraminac, A. P., and Seto, S. H. (2009). Climate-induced changes in the small mammal communities of the northern Great Lakes region. Glob. Chang. Biol. 15, 1434–1454. doi: 10.1111/j.1365-2486.2009.01846.x
Nelson, M. L., and Sagot, M. (2018). Effects of temperature and day length on daily movements and home range of Glaucomys volans (southern flying squirrel) in the Northeastern United States. Northeast. Nat. 25, 383–390. doi: 10.1656/045.025.0305
Newsome, T. M., and Ripple, W. J. (2014). A continental scale trophic cascade from wolves through coyotes to foxes. J. Anim. Ecol. 84, 49–59. doi: 10.1111/1365-2656.12258
Noss, R. F., Franklin, J. F., Baker, W. L., Schoennagel, T., and Moyle, P. B. (2006). Managing fire-prone forests in the western United States. Front. Ecol. Environ. 4, 481–487. doi: 10.1890/1540-9295(2006)4[481:MFFITW]2.0.CO;2
Nowacki, G. J., and Abrams, M. D. (2008). The demise of fire and “mesophication” of forests in the eastern United States. Bioscience 58, 123–138. doi: 10.1641/B580207
O’Brien, P. P., Bowman, J., Combs, A. B., Newar, S. L., and Garroway, C. J. (2021). Winter nest trees of sympatric northern (Glaucomys sabrinus) and southern (Glaucomys volans) flying squirrels: a test of a reinforcement in a hybrid zone. Can. J. Zool. 99, 859–866. doi: 10.1139/cjz-2021-0086
O’Brien, P. P., Bowman, J., Newar, S. L., and Garroway, C. J. (2022). Testing the parasite-mediated competition hypothesis between sympatric northern and southern flying squirrels. Int. J. Parasitol. Parasites Wildl. 17, 83–90. doi: 10.1016/j.ijppaw.2021.11.001
Olson, M. N., Bowman, J., and Burness, G. (2017). Seasonal energetics and torpor use in north American flying squirrels. J. Therm. Biol. 70, 46–53. doi: 10.1016/j.jtherbio.2017.10.006
Olson, M. N., Bowman, J., and Burness, G. (2018). Social thermoregulation does not explain heterospecific nesting in North American flying squirrels. Biol. J. Linn. Soc. 123, 805–813. doi: 10.1093/biolinnean/bly014
Patterson, J. E. H., and. Patterson, S. J. (2010) Multiple annual litters in Glaucomys sabrinus (northern flying squirrel). Northeast. Nat. 17, 167–169. doi: 10.1656/045.017.0115.
Pauli, J. N., Dubay, S. A., Anderson, E. M., and Taft, S. J. (2004). Strongyloides robustus and the northern sympatric populations of northern (Glaucomys sabrinus) and southern (G. volans) flying squirrels. J. Wildl. Dis. 40, 579–582. doi: 10.7589/0090-3558-40.3.579
Peel, G. T., Araújo, M. B., Bell, J. D., Blanchard, J., Bonebrake, T. C., Chen, I. C., et al. (2017). Biodiversity redistribution under climate change: impacts on ecosystems and human well-being. Science 355:eaai9214. doi: 10.1126/science.aai9214
Pfenning, K. S., Kelly, A. L., and Pierce, A. A. (2016). Hybridization as a facilitator of species range expansion. Proc. R. Soc. B 283:20161329. doi: 10.1098/rspb.2016.1329
Pinto, F., Romaniuk, S., and Ferguson, M. (2008). Changes to preindustrial forest tree composition in central and northeastern Ontario, Canada. Can. J. For. Res. 38, 1842–1854. doi: 10.1139/X08-034
Polley, L., Hoberg, E., and Kutz, S. (2010). Climate change, parasites, and shifting boundaries. Acta Vet. Scad. 52:S1. doi: 10.1186/1751-0147-52-S1-S1
Pyle, C., and Schafale, M. P. (1988). Land use history of three spruce–fir forest sites in southern Appalachia. J. Forest Hist. 32, 4–21. doi: 10.2307/4005019
Rentch, J. S., Ford, W. M., Schuler, T. S., Palmer, J., and Diggins, C. A. (2016). Release of suppressed red spruce using canopy gap creation – ecological restoration in the Central Appalachians. Nat. Areas J. 36, 29–37. doi: 10.3375/043.036.0108
Rentch, J. S., Schuler, T. M., Ford, W. M., and Nowacki, G. J. (2007). Red spruce stand dynamics, simulations, and restoration opportunities in the Central Appalachians. Restor. Ecol. 15, 440–452. doi: 10.1111/j.1526-100X.2007.00240.x
Reynolds, R. J., Fies, M. L., and Pagels, J. F. (2009). Communal nesting and reproduction of southern flying squirrels in montane Virginia. Northeast. Nat. 16, 563–576. doi: 10.1656/045.016.n406
Romeo, C., Piscitelli, A. P., Santicchia, F., Martinoli, A., Ferrari, N., and Wauters, L. A. (2021). Invading parasites: spillover of an alien nematode reduced survival in a native species. Biol. Invasions 23, 3847–3857. doi: 10.1007/s10530-021-02611-7
Rowe, R. J. (2007). Legacies of land use and recent climatic change: the small mammal fauna in the mountains of Utah. Am. Nat. 170, 242–257. doi: 10.1086/518948
Rowe, R. J., Finarelli, J. A., and Rickart, E. A. (2009). Range dynamics of small mammals along an elevational gradient over an 80-year interval. Glob. Chang. Biol. 16, 2930–2943. doi: 10.1111/j.1365-2486.2009.02150.x
Rowe, R. J., and Terry, R. C. (2014). Small mammal response to environmental change: integrating past and present dynamics. J. Mammal. 95, 1157–1174. doi: 10.1644/13-MAMM-S-079
Roy-Dufresne, E., Lurgi, M., Brown, S. C., Wells, K., Cooke, B., Mutze, G., et al. (2019). The Australian National Rabbit Database: 50 yr of population monitoring of an invasive species. Ecology 100:e02750. doi: 10.1002/ecy.2750
Schulte, L. A., Mladenoff, D. J., Crow, T. R., Merrick, L. C., and Cleland, D. T. (2007). Homogenization of northern U.S. Great Lakes forests due to land use. Landsc. Ecol. 22, 1089–1103. doi: 10.1007/s10980-007-9095-5
Sexton, J. P., McIntyre, P. J., Angert, A. L., and Rice, K. J. (2009). Evolution and ecology of species range limits. Annu. Rev. Ecol. Evol. 40, 415–436. doi: 10.1146/annurev.ecolsys.110308.120317
Smith, W. P. (2007). Ecology of Glaucomys sabrinus: habitat, demography, and community relations. J. Mammal. 88, 862–881. doi: 10.1644/06-MAMM-S-371R1.1
Smith, M. J., Forbes, G. J., and Betts, M. G. (2011). Evidence of multiple annual litters in Glaucomys sabrinus (northern flying squirrel). Northeast. Nat. 18, 386–389. doi: 10.1656/045.018.0312
Smith, W. P., and Person, D. K. (2007). Estimated persistence of northern flying squirrel populations in temperate rain forest fragments of Southeast Alaska. Biodivers. Conserv. 137, 626–636. doi: 10.1016/j.biocon.2007.03.020
Stapp, P. (1992). Engergetic influences on the life history of Glaucomys volans. J. Mammal. 73, 914–920. doi: 10.2307/1382216
Stapp, P., Pekins, P. J., and Mautz, W. W. (1991). Winter energy expenditure and the distribution of southern flying squirrels. Can. J. Zool. 69, 2548–2555. doi: 10.1139/z91-359
Stihler, C. W., Knight, K. B., and Urban, V. K. (1987). The northern flying squirrel in West Virginia. Proc. Southeast. Nongame Endang. Wildl. Symp. 3, 176–183.
Stronen, A. V., Tessier, N., Jolicouer, H., Paquet, P. C., Hénault, M., Villemure, M., et al. (2012). Canid hybridization: contemporary evolution in human-modified landscapes. Ecol. Evol. 2, 2128–2140. doi: 10.1002/ece3.335
Sturtevant, B. R., Bissonette, J. A., Long, J. N., and Roberts, D. W. (1997). Coarse woody debris as a function of age, stand structure and disturbance in boreal Newfoundland. Ecol. Appl. 7, 702–712. doi: 10.1890/1051-0761(1997)007[0702:CWDAAF]2.0.CO;2
Taulman, J. F., and Smith, K. G. (2004). Home range and habitat selection of southern flying squirrels in fragmented forests. Mamm. Biol. 69, 11–27. doi: 10.1078/1616-5047-113
Taulman, J. F., Smith, K. G., and Thill, R. E. (1998). Demographic and behavioral responses of southern flying squirrels to experimental logging in Arkansas. Ecol. Appl. 8, 1144–1155. doi: 10.1890/1051-0761(1998)008[1144:DABROS]2.0.CO;2
Taylor, S. A., Larson, E. L., and Harrison, R. G. (2015). Hybrid zones: windows on climate change. Trends Ecol. Evol. 30, 398–406. doi: 10.1016/j.tree.2015.04.010
Thomas, J. W., Ruggiero, L. F., Mannan, R. W., Schoen, J. W., and Lancia, R. A. (1988). Management and conservation of old-growth forests in the United States. Wildl. Soc. Bull. 16, 252–262.
Thomas, R. B., and Weigl, P. D. (1998). Dynamic foraging behavior in the southern flying squirrel (Glaucomys volans): test of a model. Am. Midl. Nat. 140, 264–270. doi: 10.1674/0003-0031(1998)140[0264:DFBITS]2.0.CO;2
Thorington, K. K., Metheny, J. D., Kalcounis-Rueppell, M. C., and Weigl, P. D. (2010). Genetic relatedness in winter populations of seasonally gregarious southern flying squirrels, Glaucomys volans. J. Mammal. 91, 897–904. doi: 10.1644/09-MAMM-A-238.1
Urban, M. C. (1988). Home range, habitat utilization, and activity of the endangered northern flying squirrel. MS Thesis, West Virginia University, Morgantown, West Virginia, USA.
Vaillancourt, M. A., Drapeau, P., Gauthier, S., and Robert, M. (2008). Availability of standing trees for large cavity-nesting birds in the eastern boreal forests of Québec, Canada. For. Ecol. Manag. 255, 2272–2285. doi: 10.1016/j.foreco.2007.12.036
Valéry, L., Fritz, H., Lefeuvre, J. C., and Simberloff, D. (2008). In search of a real definition of the biological invasion phenomenon itself. Biol. Invasions 10, 1345–1351. doi: 10.1007/s10530-007-9209-7
Valéry, L., Fritz, H., Lefeuvre, J. C., and Simberloff, D. (2009). Invasive species can also be native. Trends Ecol. Evol. 24:585. doi: 10.1016/j.tree.2009.07.003
Wallingford, P. D., Morelli, T. L., Allen, J. M., Beaury, E. M., Blumenthal, D. M., Bradley, B. A., et al. (2020). Adjusting the lens of invasion biology to focus on the impacts of climate-driven range shifts. Nat. Clim. Chang. 10, 398–405. doi: 10.1038/s41558-020-0768-2
Wan, X., Yan, C., Wan, Z., and Zhang, Z. (2022). Sustained population decline of rodents is linked to accelerated climate warming and human disturbance. BCM Ecol. Evol. 22:102. doi: 10.1186/s12862-022-02056-z
Weigl, P. D. (1978). Resource overlap, interspecific interactions, and the distribution of the flying squirrels, Glaucomys volans and G. sabrinus. Am. Midl. Nat. 100, 83–96. doi: 10.2307/2424779
Weigl, P. D. (2007). The northern flying squirrel (Glaucomys sabrinus): a conservation challenge. J. Mammal. 88, 897–907. doi: 10.1644/06-MAMM-S-333RR.1
Weigl, P. D., Knowles, T. W., and Boynton, A. C. (1992) The distribution and ecology of the northern flying squirrel, Glaucomys sabrinus coloratus, in the southern Appalachians. Final Report to North Carolina Wildlife Resources Commission, Raleigh, USA.
Wetzel, E. J., and Weigl, P. D. (1994). Ecological implications for flying squirrels (Glaucomys spp.) of effects of temperature on the in vitro development and behavior of Strongyloides robustus. Am. Midl. Nat. 131, 43–54. doi: 10.2307/2426607
Wiens, J. J. (2016). Climate-related local extinctions are already widespread among plant and animal species. PLoS Biol. 14:e2001104. doi: 10.1371/journal.pbio.2001104
Wood, C. M., Witham, J. W., and Hunter, M. L. (2016). Climate-driven range shifts are stochastic processes at a local level: two flying squirrel species in Maine. Ecosphere 7:e01240. doi: 10.1002/ecs2.1240
Ziegler, S. S. (2004). A comparison of structural characteristics between old-growth and postfire second-growth hemlock-hardwood forests in Adirondack Park, New York, U.S.A. Glob. Ecol. Biogeogr. 9, 373–389. doi: 10.1046/j.1365-2699.2000.00191.x
Keywords: biological invasions, climate change, competition, parasite-mediation, hybridization, range shifts, native species
Citation: Diggins CA (2023) Anthropogenically-induced range expansion as an invasion front in native species: An example in North American flying squirrels. Front. Ecol. Evol. 11:1096244. doi: 10.3389/fevo.2023.1096244
Edited by:
Valentina La Morgia, Istituto Superiore per la Protezione e la Ricerca Ambientale (ISPRA), ItalyReviewed by:
Gillian Holloway, Ontario Government, CanadaJeff Bowman, Ontario Ministry of Natural Resources and Forestry, Canada
Claudia Romeo, Experimental Zooprophylactic Institute of Lombardy and Emilia Romagna (IZSLER), Italy
Copyright © 2023 Diggins. This is an open-access article distributed under the terms of the Creative Commons Attribution License (CC BY). The use, distribution or reproduction in other forums is permitted, provided the original author(s) and the copyright owner(s) are credited and that the original publication in this journal is cited, in accordance with accepted academic practice. No use, distribution or reproduction is permitted which does not comply with these terms.
*Correspondence: Corinne A. Diggins, ✉ cordie1@vt.edu