Trophic Structure of Neuston Across Tropical and Subtropical Oceanic Provinces Assessed With Stable Isotopes
- 1Departamento de Biologia, CESAM – Centro de Estudos do Ambiente e do Mar, Universidade de Aveiro, Aveiro, Portugal
- 2Instituto Español de Oceanografía, Centro Oceanográfico de A Coruña, A Coruña, Spain
- 3Instituto Universitario de Investigación Marina (INMAR), Campus de Puerto Real, Universidad de Cádiz, Cádiz, Spain
- 4Red Sea Research Center (RSRC) and Computational Bioscience Research Center (CBRC), King Abdullah University of Science and Technology, Thuwal, Saudi Arabia
The marine neuston, organisms living in the vicinity of the ocean surface, is one of the least studied zooplankton groups. Neuston occupies a restricted ecological niche and is affected by a wide range of endogenous and exogenous processes while also being a food source to zooplankton fish migrating from the deep layers and seabirds. In this study, the neustonic communities were characterized along the Malaspina global expedition sampling tropical and subtropical oceanic provinces using stable carbon and nitrogen isotopes to explore their trophic structure and relationships with environmental variables. The differences in stable isotopes mirrored the patterns in environmental characteristics of each province. High δ13C values were associated with atmospheric carbon inputs, while the presence of dinoflagellates, coccolithophorids, and upwelling influence is related to low δ13C values. Similarly, provinces presenting high δ15N values were associated with denitrification and nitrate diffusive fluxes, whereas the presence of low δ15N is attributable to nitrogen supplied through N2 fixation by diazotrophs. Neuston showed a large overlap among the isotopic niches of four functional groups, with chaetognaths and detritivores generally exhibiting a smaller degree of overlap compared to carnivores and omnivores/herbivores. These results support the hypothesis of a common trophic structure in the neuston community across the ocean. However, the size of the niche, small in coastal areas and those influenced by upwelling and large in oligotrophic regions, and their overlap, low in more productive provinces and high in oligotrophic provinces, may be associated with food availability. Small trophic niches are associated with a dominance of specialized over-opportunistic feeding in productive environments.
Introduction
The neuston, one of the less described and known aquatic ecological groups, is paradoxically the closest to our sampling platforms as it inhabits the upper centimeters of the ocean. The term neuston was coined in 1972 (Hempel and Weikert, 1972) to define the pelagic organisms that occupy the vicinity of the surface layer, albeit often in a temporally-restricted and variable manner. Neuston occupies a delimited ecological niche and is generally grouped into three ecological categories: (a) euneuston: organisms with maximum abundance in the vicinity of the surface on which they reside day and night; (b) facultative neuston: organisms concentrating at the surface only during certain hours of the day, usually during darkness; and (c) pseudoneuston: organisms with maximum concentrations at deeper layers but reaching the surface layer at least during certain hours (Marshall and Burchardt, 2005). The neustonic community structure is conditioned by sunlight and an array of endogenous (organic matter, respiratory, photosynthetic, decompositional processes) and exogenous (atmospheric deposition, inorganic matter, winds, wave action, precipitation, UV radiation, oceanic currents, surface temperature) variables and processes affecting nutrient inputs and recycling (Marshall and Burchardt, 2005; Rawlinson et al., 2005; Rezai et al., 2019). Furthermore, the neuston provides a food source to the zooplankton migrating from deeper layers to the surface (Hempel and Weikert, 1972), as well as to seabirds roaming over the oceans (Cheng et al., 2010). For these reasons, the neustonic community is believed to play a critical role on the structure and function of marine food webs. Yet, research on neuston communities to date focused predominantly on geographically-limited regions of the ocean (Zaitsev, 1971; Hempel and Weikert, 1972; Holdway and Maddock, 1983; Ebberts and Wing, 1997; Rezai et al., 2019) or coastal areas (Brodeur, 1989; Le Fevre and Bourget, 1991; Padmavati and Goswami, 1996). Consequently, neuston complexity is still poorly understood as studies on the community structure and the taxonomical composition of organisms inhabiting this ecological niche remain few (Rezai et al., 2019), and global scale analyses are yet lacking.
The neustonic animals form a subset of the zooplankton community, which plays a pivotal role in the functioning of marine ecosystems. Zooplankton are partially responsible for the active energy flux between superficial and deep layers of the ocean (Turner, 2002; Jónasdóttir et al., 2015; Hernández-León et al., 2020). Zooplankton species composition, biomass, and secondary production influence a wide range of trophic levels in marine communities, as they constitute a link between primary production and secondary consumers (Litchman et al., 2013; Benedetti et al., 2016; de Oliveira Sodré and Bozelli, 2019). Copepods constitute the most abundant zooplankton taxon in terms of biomass and diversity worldwide (Kiørboe, 2011; Neumann-Leitão et al., 2018); therefore, changes in their community composition can thus impact the biogeochemical cycles (Bianchi and Mislan, 2016) and might be indicative of climate variability impacts on ecosystem functioning (Hooff and Peterson, 2006).
Historically, zooplankton assemblages research has focused mainly on taxonomic studies and those related to community structure (Pomerleau et al., 2015). However, recently, research has veered toward an alternative trait-based approach (Pomerleau et al., 2015; Benedetti et al., 2016; Campos et al., 2017), providing a perspective more focused on groups of species with analogous functional traits. This allows individuals to be classified into types characterized by the presence/absence of certain alleles of a gene, into size classes, ecological guilds, or functional groups (FGs; Tuomisto, 2010). Functional traits are phenotypes affecting organism fitness, growth, survival, and reproductive ability (Violle et al., 2007; de Oliveira Sodré and Bozelli, 2019). These are regulated by the expression of genes within species, and the expression of traits regulate, in turn, the species fitness under contrasting biotic and abiotic circumstances (Barton et al., 2013). Moreover, a specific functional trait can also develop from the interactions between other traits and environmental conditions (Kiørboe, 2011), leading to a given trait grouping being favored under certain conditions. Zooplankton traits can be classified in accordance to ecological functions – feeding, growth, reproduction, survival, and other characteristics such as morphology, physiology, behavior, or life history (Litchman et al., 2013; Hunt et al., 2015; Brun et al., 2016). Particularly, feeding strategies and trophic groups are relevant to ascertain feeding efficiency and associated predation risk (Brun et al., 2017). Additionally, they facilitate the understanding of ecosystem services associated with zooplankton, such as the distribution of fisheries or biogeochemical cycling (Prowe et al., 2019) while also allowing the positioning of zooplankton taxa in the food web (Benedetti et al., 2016, 2018).
Stable isotope analysis (SIA) has been widely used to explore the food web structure; to identify an organism’s trophic position; to quantify carbon, nitrogen, and energy fluxes; and to characterize trophic niches (Fry, 2006; Bouillon et al., 2011; Middelburg, 2014). Generally, stable isotopes undergo a predictable trophic enrichment between prey and consumer (Minagawa and Wada, 1984) and reflect the organism’s diet over a considerable period of time (Vander Zanden et al., 2015). Ratios of carbon isotopes (δ13C) generally have a low trophic enrichment and are commonly used to identify carbon sources (DeNiro and Epstein, 1978; Vander Zanden and Rasmussen, 2001; Post, 2002a). Nitrogen isotope ratios (δ15N) show progressive enrichment between prey and consumers (DeNiro and Epstein, 1978; Minagawa and Wada, 1984; Post, 2002b; McCutchan et al., 2003) and were thus employed to estimate trophic positions (Fry and Sherr, 1984; Minagawa and Wada, 1984; Peterson and Fry, 1987; Owens, 1988; Post, 2002b). Thus, by measuring the ratios of δ13C and δ15N, it is possible to infer the trophic structure of marine food webs (Fry, 2006).
Recently, SIA has been increasingly employed for the characterization of trophic niche (Layman et al., 2007a,b, 2012; Hunt et al., 2015). The trophic niche of a single species, community, or ecosystem is the aggregate of the interactions between its constituents and the ecosystem (Elton, 1927), hence representative of the characteristics of its habitat and trophic position (Leibold, 1995). The trophic niche can be inferred from the isotopic niche (e.g., the δ13C–δ15N bi-plot space) to uncover relevant aspects of trophic structure. For instance, niche-based quantitative metrics can be used to test ecological theory and trophic responses to anthropogenic impacts (Layman et al., 2007a; Schmidt et al., 2007), including the degree of overlap between distinct trophic niches (Jackson et al., 2011).
To our knowledge, the use of isotopic niche metrics on the study of the neustonic zooplankton community has never been attempted before. Here, we bridge this gap by exploring the variations in the size of the trophic niche of neustonic zooplankton across subtropical and tropical oceanic provinces. Our main hypothesis is that the trophic structure of the neustonic community is preserved in spite of differences in nutrient sources and productivity along oceanic provinces. More specifically, in this study we (1) characterize ratios of C and N for neuston across subtropical and tropical oceanic provinces, (2) determine trophic structure similarities across oceanic provinces, and (3) analyze the relationships between selected environmental variables and the trophic structure among oceanic provinces. We do so based on the samples collected along the Malaspina Circumnavigation Expedition, which circumnavigated the subtropical and tropical ocean in 2010–2011 (Duarte, 2015).
Materials and Methods
Sample Collection
The neuston samples were collected along the Malaspina 2010 Expedition, which circumnavigated the globe and was carried out between December 2010 and July 2011 across tropical, subtropical, and temperate regions of the Atlantic, Indian, and Pacific Oceans between 35° N and 40° S (Duarte, 2015). Sampling stations were distributed to characterize pelagic communities across regions of the open ocean in the northern and southern hemisphere (Duarte, 2015). In order to allow for the intercomparability of the observations and avoid adverse weather during sampling, the cruise was scheduled to visit most regions during their spring-summer months. The sampling locations were assigned to Longhurst Biogeochemical provinces (Longhurst, 2007). There, four biomes (Polar, Westerlies, Trades and Coastal) and a total of 56 provinces were identified, based on the characterization of primary production, mixed depth layer, nutrients availability, photic depth, algal biomass, Brunt-Väisälä frequency, and the Rossby radius of internal deformation. Specifically, the neuston samples reported here included 10 oceanic Longhurst provinces (Longhurst, 2007). Four provinces in the Atlantic [Northeast Atlantic subtropical gyral (NASE; n = 16); North Atlantic tropical gyral (NATR; n = 14); Western tropical Atlantic (WTRA; n = 15); South Atlantic gyral (SATL; n = 12)], four in the Pacific [South Pacific gyre (SPSG; n = 18); Pacific equatorial divergence (PEQD; n = 12); North Pacific tropical gyre (NPTG; n = 14); North Pacific equatorial counter current (PNEC; n = 15)], and two in the Indian Ocean [Indian South subtropical gyre (ISSG; n = 18); South subtropical convergence (SSTC; n = 16); Figure 1; Supplementary Table S1]. Between three and four stations were sampled within each province. Samples were collected twice a day at 12 pm and 4 am, by towing a neuston sampler with a mouth opening of 80 × 30 cm and a mesh size of 200 μm, at 2–3 knots for 10–15 min, which sampled the first 15 cm of the water at a distance of 5 m from the starboard of the vessel (González-Gordillo et al., 2012). The content of each sample was stored in 4% formaldehyde until analysis. Environmental data were acquired using CTD casts deployed at each station from the surface to 4,000 m or 100 m above the seafloor when this was shallower than 4,000 m.
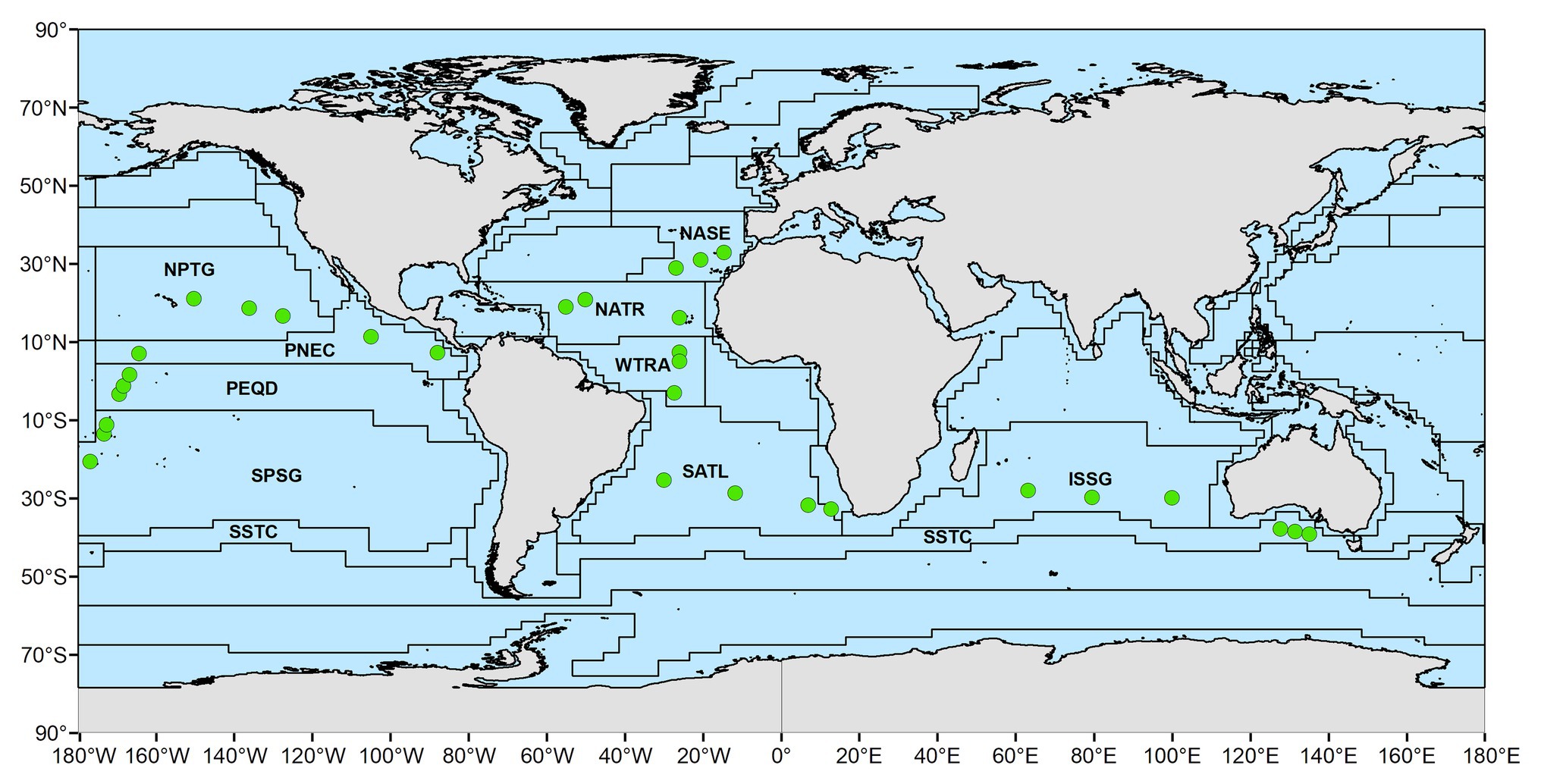
Figure 1. Location of the stations sampled in this study, indicated by green circles. Stations were grouped in distinct biogeochemical provinces according to Longhurst (2007) with acronyms depicted. Shapefile adapted from Flanders Marine Institute (2009).
Sample Processing, Taxonomical Identification, and Functional Grouping
Samples were stained with Bengal Rose in order to facilitate the identification of the organisms to taxonomic level of a family which, whenever possible, was determined under a stereomicroscope (Olympus SZX16) using appropriate guides (Boltovskoy, 1999; Castellani and Edwards, 2017). The most abundant and representative taxa selected for SIA were copepods of the families Acartiidae, Calanidae, Corycaeidae, Oncaeidae, and Pontellidae, and Phylum Chaetognatha. The latter were included as a representative of top planktonic predators. Each of these taxa have specific trophic roles, according to the literature. For instance, copepod families were assigned to FGs according to Benedetti et al. (2016, 2018). Four FGs were considered and taxa were assigned as follows: FG1 = detritivores (Oncaeidae); FG2 = herbivores/omnivores (Acartiidae and Calanidae); FG3 = carnivores (Corycaeidae and Pontellidae); and FG4 = predators (Chaetognatha). All specimens selected for analysis were adults according to their morphological aspect under the microscope.
Stable Isotope Analysis
Analysis of the natural abundance of carbon and nitrogen isotopes were performed on previously dried (50°C, 48 h) neuston samples. Even when specimens were classified at genus or species level, we pooled individuals from the same sample station, including day and night samples, at family (Acartiidae, Calanidae, Corycaeidae, Oncaeidae, and Pontellidae) or phylum (Chaetognatha) level in order to obtain ca. 1 mg dry weight for isotopic determination and to achieve a minimum of three data points for each functional group and minimize the constraints of small sample size (Jackson et al., 2011). Dried samples were packed into tin capsules and measured in an elemental analyzer (Carlo Erba CHNSO 1108) coupled to an isotope-ratio mass spectrometer (Finnigan Matt Delta Plus). Isotopic analyses were performed by the Servicio de Análisis Instrumental of the Universidade da Coruña (Spain). Samples were not acidified in order to remove carbonates since the selected taxa were only slightly calcified, and it has been demonstrated that this procedure could impact nitrogen measurements (Mateo et al., 2008). No corrections were made for the possible effect of formaldehyde on the stable isotope composition as most studies specifically made on marine zooplankton samples point out to no significant effects in δ15N and generally a decrease of less than 2‰ in δ13C after several years of storage (e.g., Mullin et al., 1984; Bicknell et al., 2011; de Lecea et al., 2011). Taking into consideration that all samples have been preserved similarly and that the time between collection and SIA largely exceeded 1 year, we assumed that differences in stable isotope values reflect genuine variations in the provinces and selected FGs.
Values of natural abundance of stable isotopes were expressed as δ13C and δ15N (‰) relative to Vienna Pee Dee Belemnite and atmospheric nitrogen, respectively (Coplen, 2011). Certified isotope standards (USGS40 and L-alanine) were analyzed along with internal acetanilide and sample standards with standard deviation (SD) between certified and measured values <0.1‰. The precision [standard error (SE)] of replicate determinations of standards and samples was <0.05‰ for both isotopes (n = 4). As the C:N mass ratio of most samples exceeded 3.5 (thus suggesting a significant and variable lipid content), δ13C values were normalized using an empirical linear regression with the sample C:N value determined for aquatic organisms (Post et al., 2007). This procedure aimed at removing the effect of the low δ13C associated to lipids and was preferred due to low biomass constraints that hindered the use of lipid removal methodologies such as dichloromethane and an accelerated solvent extraction system (Bodin et al., 2009).
Environmental Variables
Environmental attributes of each of the sampled stations were characterized by a number of variables collected in situ or satellite-derived. (Supplementary Table S2). These variables were already employed in a previous analysis of Malaspina 2010 cruise, where methodological details can be found (Mompeán et al., 2013, 2016b; Fernández-Castro et al., 2015). In brief, the stratification of the water column was represented by the depth of the mixing layer (MLD, m), the mean squared Brunt–Väisälä frequency (N2, s−2), and the depth of the chlorophyll maximum (DCM, m), all estimated from vertical profiles of a CTD equipped with a fluorescence sensor (Fernández-Castro et al., 2015). Similarly, nutrient inputs from deep layers were estimated by diffusivity due to turbulence (KT, m2 s−1), determined from vertical casts of a microstructure turbulence profiler (Fernández-Castro et al., 2015). Phytoplankton biomass was represented by surface and photic-zone integrated chlorophyll-a (Chlas and Chlai, mg m−3 and mg m−2, respectively), determined from acetonic extracts of phytoplankton (Estrada et al., 2016). Microplankton (40–200 μm) characteristics were indicated by an abundance of the nitrogen-fixer Trichodesmium (Tricho, cells ml−1), carbon biomass (C40–200, mg C m−3), and natural abundance of nitrogen isotopes (δ15N40–200, ‰) measured in samples collected by vertical tows of a plankton net between the surface and 200 m depth (Mompeán et al., 2013, 2016b). Satellite derived variables were considered to represent conditions prevailing over larger spatial and temporal scales than those considered during the specific sampling of each station and included annual averages of primary production (PP) for 2010 (mg C m−2 d−1) and mean monthly atmospheric dust deposition (MDU, g m−2 month−1). The former represented regional productivity and was derived from the data provided by the Ocean Productivity website (http://www.science.oregonstate.edu/ocean.productivity/index.php) in a grid of 0.17° × 0.17° including each station position. Dust deposition, a proxy for atmospheric inputs of key nutrients as Fe or P, was estimated from Aqua-MODIS Aerosol Optical Depth at 550 nm and Aerosol Small Mode Fraction data provided by the Giovanni online data system (NASA Goddard Earth Sciences) from a grid of 1° × 1° near each station (Mompeán et al., 2016b). In depth information on sampling and analytical methodology employed throughout the Malaspina 2010 cruise can be found in Moreno-Ostos (2012).
Statistical Analysis
Differences in δ13C and δ15N ratios of Oncaeidae among oceanic provinces were tested with ANOVA and Bonferroni post hoc test on Statistica 12 (StatSoft, Inc., Tulsa, OK, USA). Analysis of the differences in stable isotope values among oceanic provinces was assessed using a permutational multivariate analysis of variance (PERMANOVA) and pairwise tests on PRIMER 6.0 (Clarke and Gorley, 2006) and the add-on package PERMANOVA+ (Anderson et al., 2008; Supplementary Table S3). For subsequent analysis, a representative primary consumer was used to normalize stable isotope values according to Clark and Fritz (1997) and Stasko et al. (2018) to account for spatial heterogeneity in carbon and nitrogen ratios. To this end, consumer δ13C values were normalized relative to a pelagic baseline (Δ13Cpel) as: , were δC is the consumer δ13C value and δW is the province-specific mean δ13C value of the copepod family Oncaeidae, considered as the reference baseline. Consumer δ15N values were normalized by subtracting the province-specific mean δ15N value of the copepod family Oncaeidae from the consumer δ15N, represented thereafter as δ15Nn. The use of Oncaeidae as baseline is not intended to reflect the base of the food web but rather to set a homogeneous starting point along the δ13C and δ15N continuum. All statistical analyses were from this point on performed on Oncaeidae-normalized values.
Analysis of the isotopic niche size were made in the isotopic space defined by Δ13Cpel–δ15Nn of each province/functional group combination with the package SIBER (Jackson et al., 2011). Bayesian estimates of the standard ellipse area (SEAb) were calculated for each combination to characterize the full variability in foraging habits and used resources (Layman et al., 2007a, 2012). These estimates accounted for uncertainty due to the number of samples. A minimum of three samples was required for these estimations. SEAb statistics were computed from 104 simulations per province/functional group arrangement, although only the maximum likelihood ellipses were used for graphical representations. Pairwise comparisons of SEAb (Supplementary Table S4) were performed by calculating the proportion of ellipse size that differed between two given combinations being interpreted as a direct proxy for the probability that one combination is different from the other (Jackson et al., 2011). Because of the limitations imposed by the available sample biomass for isotopic analyses, data of some FGs could not be obtained for all provinces.
Principal component analysis (PCA) was used to investigate the relationships between environmental variables and the niche size (as a proxy for trophic structure) of the FGs among oceanic provinces using PRIMER 6.0 (Clarke and Gorley, 2006). First, normalized environmental variables measured on each sampling site were employed to compute the eigenvalues, eigenvectors, and principal component (PC) coordinates. Then, Pearson’s correlation coefficient between SEAb average and 95% credible intervals (CI) range for each functional group/province combination and the environmental PC coordinates were computed using the corr() function on R 3.6.3 (R Core Team, 2020). Finally, the correlation coefficients between the niche metrics and the PCA axes were plotted on the variable space of the PCA first two principal component coordinates axis.
Results
δ13C and δ15N Ratios
The mean δ13C for Oncaeidae varied significantly among provinces (Figure 2A). Bonferroni post hoc test revealed three distinct groups (a, b, and c) of decreasing mean values (−21.2, −21.8, and −22.3‰, respectively). The highest values were found in NATR and SPSG provinces, while the lowest values occurred in NASE. Similarly, significant differences in mean values of δ15N between provinces were also found (Figure 2B). All δ15N values were positive, with maximum values in SSTC province, followed by those in PNEC and NPTG, and minimum values (<2‰) in NATR. Bonferroni post hoc tests also revealed three groups (d, e, and f) of decreasing mean δ15N (10.1, 5.4, and 3.5 ‰, respectively).
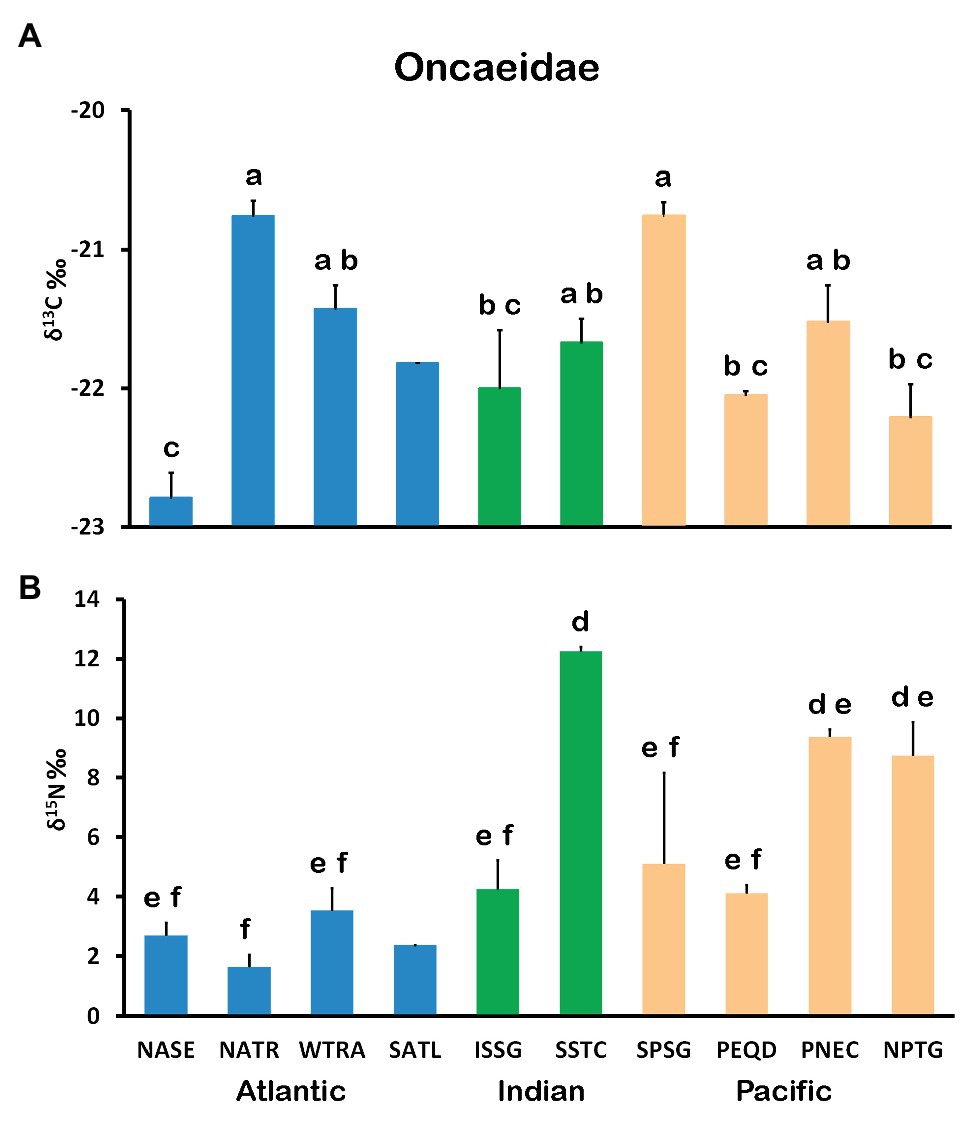
Figure 2. Mean and SE values of δ13C (A) and δ15N (B) for Oncaeidae by Longhurst Provinces. Groups of significantly different means (ANOVA and Bonferroni post hoc test, p < 0.05) are indicated by different letters (a, b, c, d, e, and f).
Isotopic Niche Size of Functional Groups
The analysis of the isotopic niches revealed differences between provinces and FGs in all oceans. The maximum likelihood ellipses were distinct from each other in all provinces and FGs but showed large overlaps between them in some provinces/functional group arrangements.
Atlantic Provinces
There was a clear separation of the ellipses of top predators (FG4) and detritivores (FG1) in the provinces where these groups were analyzed (Figure 3). In contrast, ellipses for herbivores/omnivores (FG2) and carnivores (FG3) showed large overlaps. The variability of SEAb (Bayesian standard ellipse area) was highest for FG3 and lowest for FG2 (Table 1).
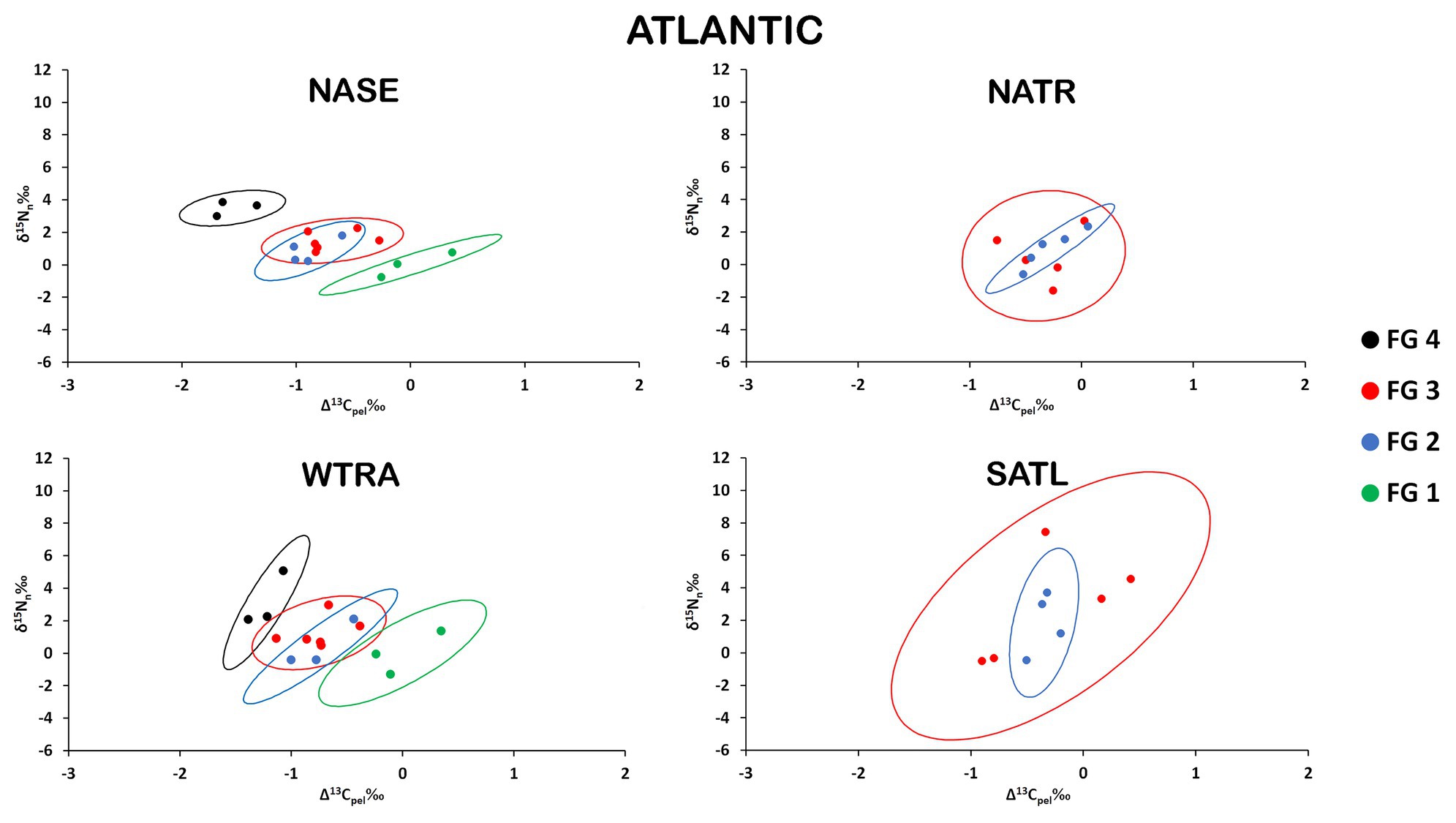
Figure 3. Isospace of Δ13Cpel and δ15Nn including individual sample normalized values of neustonic zooplankton for biogeochemical provinces in the Atlantic Ocean: NASE, NATR, WTRA, and SATL. The maximum likelihood estimates of the standard ellipse areas (thick lines) for each functional group (FG) are shown. FG 1 = detritivores; FG 2 = herbivores/omnivores; FG 3 = carnivores; FG 4 = predators. Decreases in Δ13Cpel reflect increases in raw δ13C values due to normalization.
Pairwise comparisons of the isotopic niche areas using SEAb revealed that only FG3 varied significantly from small values in NASE, intermediate in NATR and high values in SATL, while no significant differences resulted when comparing other FG among Atlantic provinces (Supplementary Table S4).
Indian Provinces
Distinct maximum likelihood ellipses were present in both province/FGs arrays; nonetheless, significant overlaps are discernible throughout the isospace (Figure 4; Table 1). SEAb was consistently larger for all FGs in the ISSG province and the largest trophic variability was found for FG2. Moreover, SEAb were the smallest for both FG1 and FG3 in SSTC.
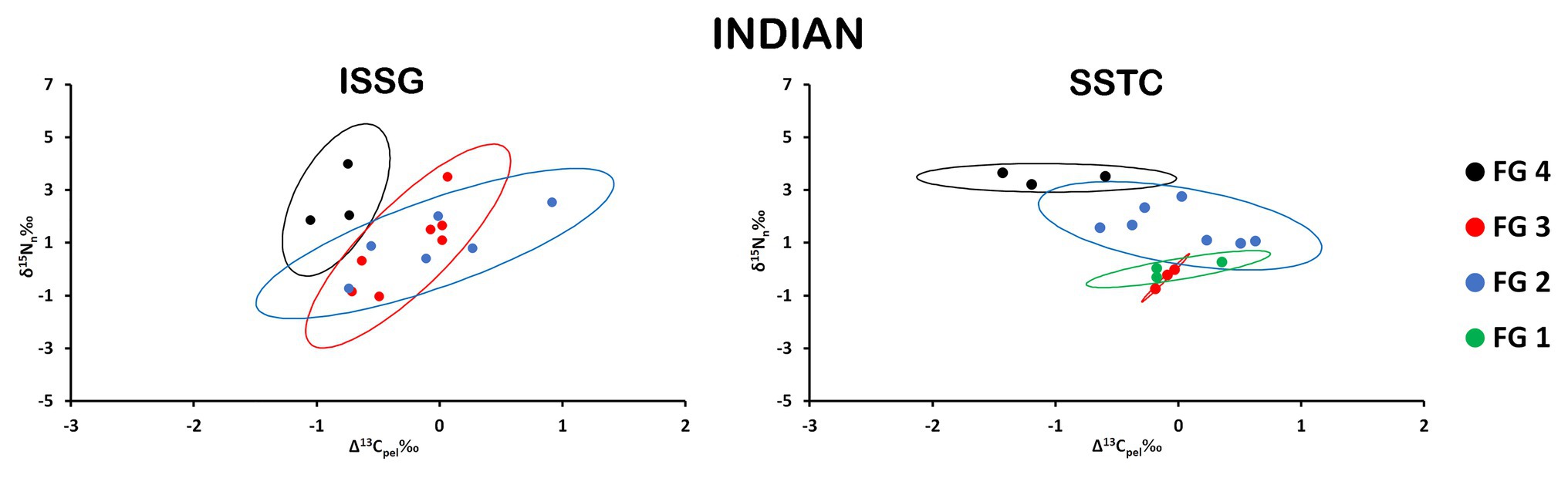
Figure 4. Isospace of Δ13Cpel and δ15Nn including individual sample normalized values of neustonic zooplankton for biogeochemical provinces in the Indian Ocean: ISSG and SSTC. The maximum likelihood estimates of the standard ellipse areas (thick lines) for each FG is shown. FG 1 = detritivores; FG 2 = herbivores/omnivores; FG 3 = carnivores; FG 4 = predators. Decreases in Δ13Cpel reflect increases in raw δ13C values due to normalization.
There were no significant differences in SEAb of equivalent FG among Indian provinces (Supplementary Table S4).
Pacific Provinces
The isospace area of the Pacific provinces follows the trend described for Atlantic and Indian provinces, with perceptible differences in the distinct provinces/FGs arrangements, but the large overlap was found in many cases, especially in PNEC and SPSG (Figure 5). The largest values of SEAb were found for FG4 in SPSG and the minimum values for FG2 in PEQD (Table 1). NPTG and PEQD presented narrower SEAb compared to SPSG and PNEC. Pairwise comparisons of SEAb indicated that the values of FG1 were significantly smaller for PNEC compared to those for SPSG and that those for FG2 in NPTG and PEQD were smaller than those in PNEC and SPSG, while those for FG3 in PNEC and SPSG were larger than their equivalent areas in NPTG and PEQD. Finally, SEAb of FG4 in PNEC and SPSG were larger than their comparable group in NPTG (Supplementary Table S4).
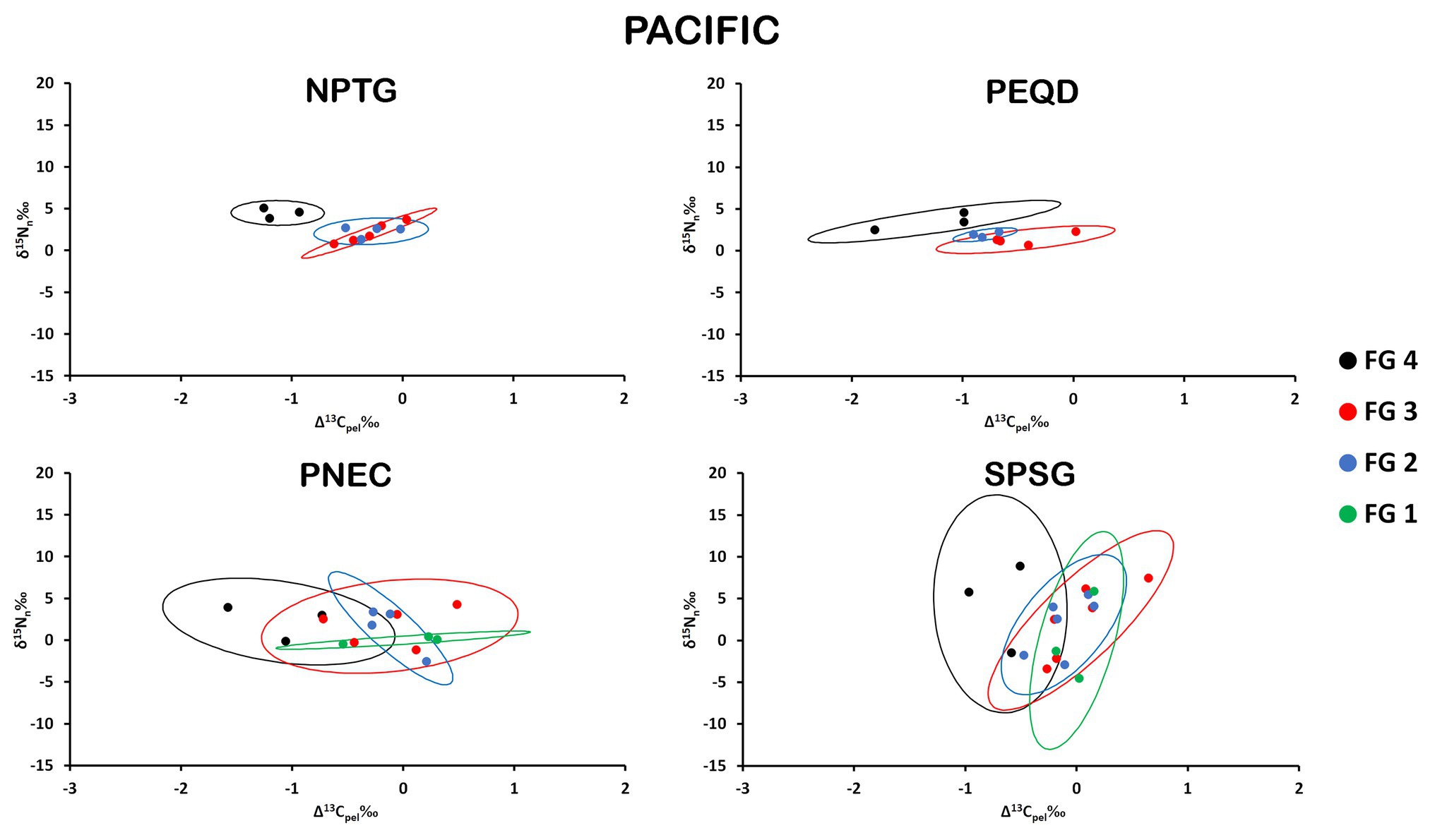
Figure 5. Isospace of Δ13Cpel and δ15Nn including individual sample normalized values of neustonic zooplankton for biogeochemical provinces in the Pacific Ocean: NPTG, PEQD, PNEC, and SPSG. The maximum likelihood estimates of the standard ellipse areas (thick lines) for each FG are shown. FG 1 = detritivores; FG 2 = herbivores/omnivores; FG 3 = carnivores; FG 4 = predators. Decreases in Δ13Cpel reflect increases in raw δ13C values due to normalization.
Environmental Variables and Trophic Structure
The PCA on the environmental variables revealed distinctive conditions among provinces, as stations are clearly clustered within each province (Figure 6). The first two principal components of the PCA accounted for 66.4% of the total variance (Table 2). Surface chlorophyll had the largest positive loadings with the first component (PC1), followed by the microplanktonic carbon biomass and turbulence diffusivity (Figure 7). Conversely, PC1 was negatively correlated with the depth of chlorophyll maximum. The second component (PC2) was positively correlated with primary production, δ15N40–200, and the mixed layer depth, while showing negative correlations with atmospheric dust deposition, Trichodesmium abundance, and photic-zone integrated Chl-a. The remaining environmental variables have a minor relevance in explaining the reported differences (Figure 7).
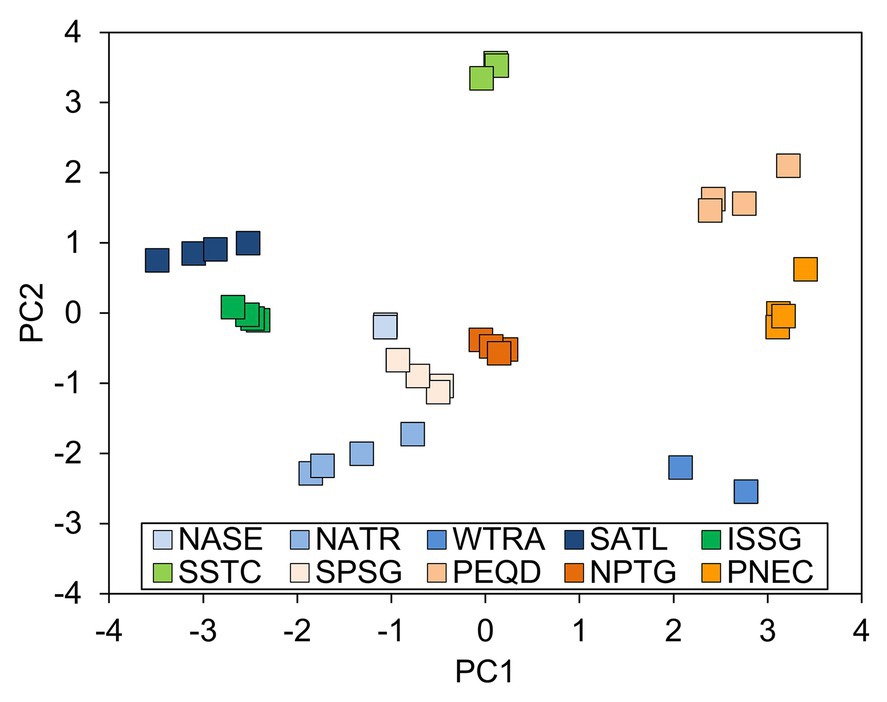
Figure 6. Projection of samples on the space of the first (PC1) and second (PC2) components of the principal component analysis (PCA) of environmental variables (DCM: depth of the chlorophyll maximum (m), δ15N40–200: natural abundance of microplankton nitrogen isotopes (‰), PP: annual mean primary production (mg C m−2 d−1), MLD: mixed layer depth (m), C40–200: microplankton (40–200 μm) carbon biomass (mg C m−3), KT: diffusivity due to turbulence (m2 s−1), Chlas: surface chlorophyll-a (mg m−3), N2: mean squared Brunt-Väisälä frequency (s−2), Chlai: photic-zone integrated chlorophyll-a (mg m−2), Tricho: abundance of Trichodesmium (cells ml−1), MDU: mean monthy atmospheric dust deposition (g m−2 month−1)) determined for neuston sampling stations. Province acronyms as in Supplementary Table S1.
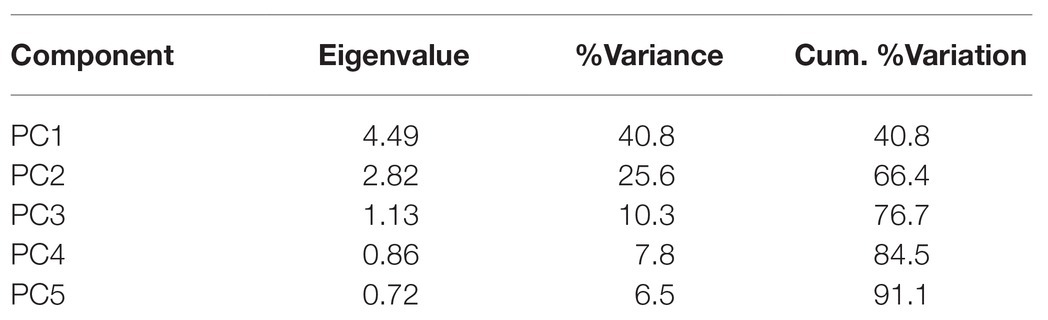
Table 2. Eigenvalues, percent of total variance (%Variance), and percent of cumulative variation (Cum. %Variation) explained of the first five principal components (PC) of the principal component analysis (PCA) on environmental variables.
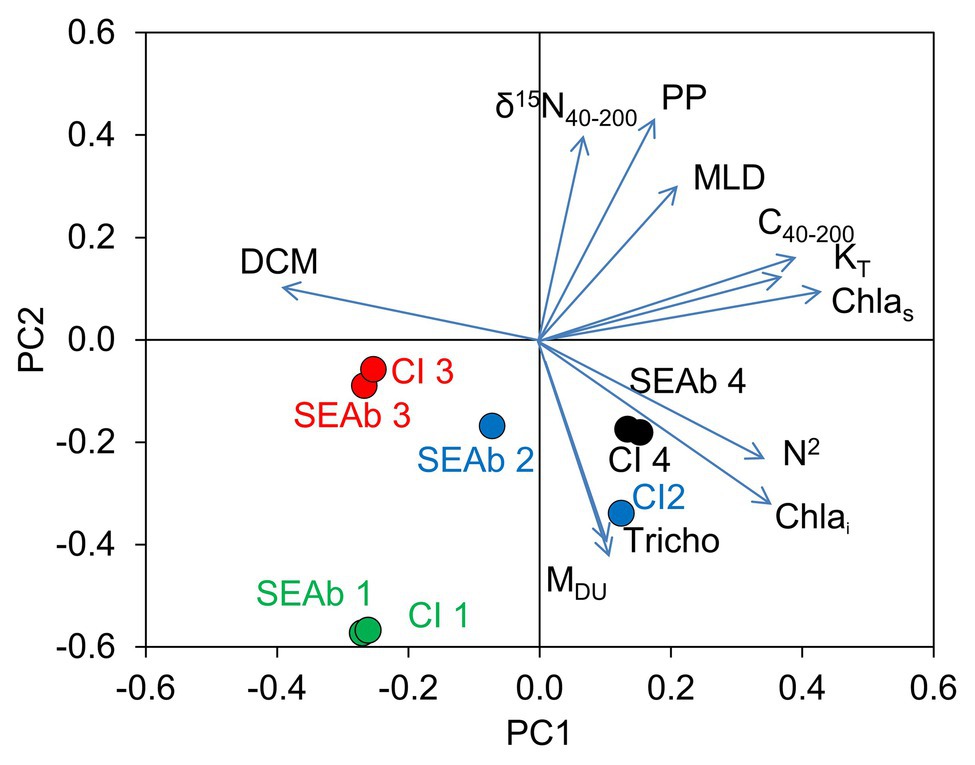
Figure 7. Loadings (vectors) of the environmental variables on the space of the first (PC1) and second (PC2) components of the PCA of environmental variables determined for neuston sampling stations and projections (dots) of median values of niche size (SEAb) and 95% credible intervals (CI) of the different FGs (indicated by numbers 1–4). DCM: depth of the chlorophyll maximum (m), δ15N40–200: natural abundance of microplankton nitrogen isotopes (‰), PP: annual mean primary production (mg C m−2 d−1), MLD: mixed layer depth (m), C40–200: microplankton (40–200 μm) carbon biomass (mg C m−3), KT: diffusivity due to turbulence (m2 s−1), Chlas: surface chlorophyll-a (mg m−3), N2: mean squared Brunt-Väisälä frequency (s−2), Chlai: photic-zone integrated chlorophyll-a (mg m−2), Tricho: abundance of Trichodesmium (cells ml−1), MDU: mean monthly atmospheric dust deposition (g m−2 month−1).
The size of the isotopic niche (indicated by median SEAb values and 95% CI) of FG4 was positively correlated with PC1, while those of FG3 showed negative correlations with this component. In turn, SEAb of FG1 and FG2 were mainly negatively correlated with PC2 (Figure 7).
Discussion
In the current study, we provide the first comparison of the trophic structure of the neustonic community in tropical and subtropical provinces of the Atlantic, Indian, and Pacific Oceans (Longhurst, 2007; Duarte, 2015). The analysis of stable carbon and nitrogen isotopes of neuston FGs allowed for the characterization of carbon and nitrogen ratios, the quantification of the size of the isotopic niche as a proxy of trophic structure, and its relationships with environmental variables across distinct oceanic provinces. Notwithstanding the limitations imposed by the analytical requirement and, thus, the small number of samples employed, as well as the samples preservation method, the results of this study allow us to establish an approximation of the trophic interactions in the neuston and their role in pelagic food webs at a global scale.
δ13C and δ15N Ratios
The differences found in carbon and nitrogen appear to reflect variations in the environmental regimes of the distinct provinces. Provided that different phytoplankton taxa favor the use of distinct carbon sources for primary production, it is plausible that it could lead to variations in δ13C that can be passed upon the food web. For instance, heavier δ13C values have been associated with higher phytoplankton fractionation of inorganic carbon, and subsequent sinking of organic matter relatively depleted in 13C from the surface waters led to high values in the upper ocean at subtropical and tropical waters (Gruber et al., 1999; Schmittner et al., 2013). Moreover, uptake of atmospheric CO2 via air-sea exchange and consequent fractionation in the conversion process to HCO− 3 can result in high δ13C-dissolved inorganic carbon (Zhang et al., 1995). Such processes could explain the enrichment in 13C (i.e., higher δ13C) found for the isotopic baseline (Oncaeidae) in the SPSG and NATR. Additionally, the enrichment in δ13C measured in SPSG could also be related to the presence of diatoms, as reported in the region during the same cruise (Estrada et al., 2016). Although they were not the dominant phytoplankton taxa (Estrada et al., 2016), they may have disproportionately contributed to the metazoan food web, while the dominant picocyanobacteria would contribute to the microbial food web. While cyanobacteria uptake of inorganic carbon occurs via direct HCO− 3 transport, diatoms are also capable of transporting CO2 derived from the catalyzed dehydration of HCO− 3 (Tortell and Morel, 2002). This implies a larger isotopic fractionation (i.e., less negative values of δ13C) in diatoms when compared with cyanobacteria or other microalgae (Fry and Wainright, 1991). Another hypothesis for the δ13C enrichment measured in NATR province is related to the abundance of Sargassum spp. on the westernmost stations (Gouvêa et al., 2020). This macroalgae has a δ13C ranging from −16‰ to −18‰, and it is conceivable that it could have influenced the δ13C baseline in NATR (Cabanillas-Teran et al., 2019). However, differences in δ13C could be the result of many factors associated with changes in phytoplankton CO2 fixation, which is known to vary in relation to temperature, concentration of aqueous CO2, phytoplankton composition, and the availability of dissolved inorganic carbon and nutrients (Wong and Sackett, 1978; Descolas-Gros and Fontugne, 1990; Francois et al., 1993; Burkhardt et al., 1999; Popp et al., 1999).
Both continental organic matter and atmospheric CO2 are characterized by having lower values of δ13C than their equivalents in oceanic (Perry et al., 1999) or upwelled waters (Gruber et al., 1999). Our results agree with the report of higher δ13C values in zooplankton from oligotrophic regions of the subtropical North Atlantic during the same cruise (Mompeán et al., 2013). In contrast, more negative δ13C values of the Oncaeidae baseline, such as those measured in NPTG and NASE, can be explained by the dominance of dinoflagellates and coccolithophores in these provinces (Estrada et al., 2016) and by the influence of upwelling in NASE (Gruber et al., 1999; Mompeán et al., 2013).
Ratios of nitrogen also varied between provinces, according to the differences found in δ15N values of Oncaeidae. Low δ15N values could be due to diazotrophy since the atmospheric nitrogen presents δ15N = 0‰, and it can be traced through the food web (McClelland et al., 2003; Mompeán et al., 2016a; Bode and Hernández-León, 2018). This process is mediated by specialized prokaryotes – diazotrophs – which thrive in usually nitrate-poor, warm and stratified waters such as the ones of the subtropical and tropical gyres (Falkowski, 1997; Zehr et al., 2003; Capone et al., 2005; Luo et al., 2012) and introduce into the ocean bioavailable nitrogen depleted in δ15N (Somes et al., 2010). Additionally, it is also known that isotopic fractionation occurs during phytoplankton uptake, and this may cause low δ15N values (<5‰) in plankton when dissolved nitrogen concentrations are high, as reported at the initial phases of blooms (Waser et al., 2000). In turn, high concentrations of 15N-enriched nitrate of marine origin are similarly reflected in the δ15N values of zooplankton (Owens, 1988; Montoya et al., 2002), as found in the SSTC samples. Our results strongly suggest that the low δ15N values measured for Oncaeidae reflect the role of N2 fixation by diazotrophs in supplying N in some provinces (mainly in NATR but also in NASE, WTRA, SATL, SPSG, and PEQD), while the high δ15N values measured in other provinces indicate inputs of nitrogen derived from denitrification processes (e.g., PNEC, NPTG, and SSTC). These assumptions are supported by the consistency with estimations of the abundance of the N-fixer Trichodesmium (Estrada et al., 2016; Mompeán et al., 2016b) and N fixation rates and NO3 − diffusive fluxes obtained for the Malaspina cruise (Fernández-Castro et al., 2015), as well as recent estimates of marine nitrogen fixation and denitrification at the scale of the global ocean (Knapp et al., 2016; Bonnet et al., 2017; Gruber, 2019; Wang et al., 2019).
Moreover, the δ15N values reported here for Oncaeidae are consistent with those of microplankton collected simultaneously during the Malaspina cruise (δ15N40–200) in the upper 200 m of the water column, thus supporting our assumption of the role of Oncaeidae as primary consumers and critical nodes in the food web. Furthermore, the general coherence between the distribution pattern of both Oncaeidae and microplankton δ15N across provinces (e.g., Mompeán et al., 2016b; Supplementary Figure S1) suggests similar turnover times of the stable isotopes (and hence growth rates) in epipelagic microplankton and, in our case, mesoneuston (>200 μm).
Spatial Differences in Isotopic Niche
The small layer of surface water the neustonic community inhabits would imply a scarce availability of food sources, particularly in more oligotrophic regions, such as most of provinces in this study. This suggests a strong competition for resources and a large overlap in the isotopic niche for the different FGs. Our results show a general overlap between the niches of the four FGs regardless of the province. More specifically, herbivores/omnivores and carnivores had large overlap between them and with other groups in most provinces, while chaetognaths and detritivores had small overlaps.
The large overlap in the isotopic niches in all provinces supports the hypothesis of a common trophic structure of the neustonic community, notwithstanding regional and local differences in nutrient sources and productivity. Such structure would be facilitated by the large plasticity of the isotopic (trophic) niche of the main FGs. Whenever present, chaetognaths occupied the highest trophic position as expected from their obligate carnivorous feeding (Pearre and Pearre, 1982; Kehayias et al., 1996; Baier and Purcell, 1997). Conversely, detritivores generally occupied the lowest end of the δ15Nn spectrum. Despite the low trophic fractionation generally associated to δ13C (e.g., Post, 2002b), the Oncaeidae baseline generally shows the lowest δ13C values, with a consistent increase in δ13C from FG1 to FG4 in most provinces. This is, in turn, reflected by a decrease in Δ13Cpel from FG1 to FG4 due to our baseline normalization. The intermediate FGs (FG2 and FG3) were particularly variable in δ15Nn, irrespective of the pre-assigned trophic category as herbivore/omnivore or carnivore, thus limiting the applicability of these classifications in the neuston (Benedetti et al., 2016, 2018). This is illustrated in the Atlantic provinces where both productive and oligotrophic regions are present. Here, the more productive regions (NASE and WTRA) show a defined hierarchical trophic structure, contrasting with the oligotrophic provinces of NATR and SATL.
Our results show the importance of omnivory in pelagic food webs, particularly in oligotrophic regions of the oceans, such as the majority of the provinces sampled in this study. This plasticity in feeding preferences increases trophic connectivity and ultimately the ecosystem resilience and stability (Madigan et al., 2012). Additionally, organisms of intermediate trophic positions generally have varied diets, hence expanding their options to feed on organisms from various trophic positions (Svanbäck et al., 2015). Therefore, a large degree of omnivory combined with opportunistic feeding would significantly increase the size of the isotopic niche, as observed in the studied FGs of neuston across the global ocean. This strategy may facilitate their survival in the oligotrophic environment of the sub-gyres and tropical gyres of the ocean and is further supported by the large niche overlap observed as a general pattern in our results.
However, the degree of niche overlap between FGs on each province was variable. For example, provinces such as NASE and SSTC presented a smaller degree of overlap compared to provinces such as WTRA and SPSG. The degree of niche overlap appears to be related with the food availability on each province, since the former provinces are in the proximity of coastal areas and under the influence of nutrient-rich upwelling waters, while the latter provinces are in oligotrophic regions of the ocean. However, large niche overlap is not a direct indication of competition for the same sources of food, as organisms may be preying on different resources that share similar isotopic signals (Layman et al., 2012). For example, Bode et al. (2015) found an important contribution of phytoplankton for some copepod species, while others showed relevant influence of bacteria, probably derived from preying upon ciliates and bacterivorous plankton. Furthermore, a study encompassing the same provinces in the Atlantic Ocean as our study observed that several of the most abundant mesopelagic fish presented comparable trophic positions but differed in their diets, the latter related to local prey availability (Olivar et al., 2019).
Environmental Variables and Trophic Structure
The results of the PCA on the environmental variables confirmed the expected singularity of samples within the biogeochemical divisions as proposed by Longhurst (2007). Furthermore, our results showed that the structure of FGs was indeed influenced by the environmental variables considered, as changes in some variables were more relevant to the size of the isotopic niches of certain FGs. The negative correlation of the size of the niche of FG1, and to a lesser extent FG2, with PP is symptomatic of trophic specialization, meaning that as PP increases the size of the niche of herbivores and detritivores decreases, since these copepods would be able to select the preferred feeding sources. Alternatively, the niche width would increase in provinces with relatively large amounts of dust deposition and Trichodesmium, an indication of diazotrophic zones (Fernández-Castro et al., 2015; Mompeán et al., 2016a), characterized by nutrient-depleted waters and lower abundance of prey (Carlotti et al., 2018). Transfer of diazotrophic nitrogen to zooplankton (including neuston) would occur not only by direct grazing of the cyanobacteria but also by indirectly preying upon organisms of the microbial loop (Sommer et al., 2006; Wannicke et al., 2013). The need for including more diverse prey would thus explain the increase in the niche size.
The size of the isotopic niche of carnivores was positively correlated with DCM depth and negatively with microplankton biomass and surface Chl-a. It has been reported that the optimum prey size of copepods ranges between 10 and 100 μm (Hansen et al., 1994; Li et al., 2007); therefore, increases in abundance of microplankton would allow for a prey selection better suited to their dietary preferences, thus reducing their isotopic niche size. In turn, the positive correlation of the niche size of chaetognaths with Trichodesmium, stratification, and dust deposition suggests an increase in the variety of their prey in diazotrophic provinces, as suggested for herbivores and omnivores.
Our results suggest a relationship between the size of the isotopic niche and their overlap with other FGs. In this way, the FGs of provinces closer to coastal areas or influenced by upwelling (e.g., NASE, SSTC, and NPTG) exhibit relatively small niches, suggesting that the abundance and diversity of available prey allows for a more specialized feeding according to the theoretical functional group of each organism. This is supported by the high importance of diffusive nitrate inputs described for Malaspina stations in these provinces, particularly in SSTC (Fernández-Castro et al., 2015). In contrast, highly oligotrophic provinces (e.g., SATL, ISSG, and SPSG) present generally broader isotopic niches.
The occurrence of opportunistic feeding on available prey would trigger variations in the isotopic signal of both prey and consumers throughout the food web, therefore resulting in larger isotopic niches in provinces characterized by low primary productivity and low plankton biomass. Similar observations have been reported for both the Atlantic Ocean and the Eastern South Pacific, where zooplankton communities of oligotrophic regions depicted larger niches compared to those of more productive regions (Bode and Hernández-León, 2018; González et al., 2019). An exception to this is the PNEC province, where two of the three stations sampled in this study were under the influence of the Costa Rica Dome, an enhanced biological productivity region, where phytoplankton and zooplankton biomass are larger than in neighboring tropical waters (Fiedler, 2002). Nonetheless, while being a highly productive system its phytoplankton community is dominated by the picophytoplankton Synechococcus (Taylor et al., 2015), and it has been reported that zooplankton are still able to match their metabolic needs by protistivory and detritivory (Stukel et al., 2018), thus increasing the variability of individual samples and consequently the estimated niche size. Comparable results have also been found in the Indian Ocean where the niche sizes of an oligotrophic seamount were smaller than those of a more productive one (Annasawmy et al., 2020). Furthermore, PNEC is affected by distinct oceanographic processes (upwelling, minimum oxygen zone), the confluence of different water masses (Lavin and Marinone, 2003; Rau et al., 2003; Fiedler and Talley, 2006), and nitrification-denitrification processes (Gruber, 2019).
Conclusion
Values of C and N isotopic ratios for the neuston are dependent on the environmental characteristics of the oceanographic regimes of each province. Uptake of atmospheric carbon were found to be associated with high δ13C values, whereas upwelling influenced regions or the presence of dinoflagellates and coccolithophores appear to be related with low δ13C. Additionally, high δ15N is a consequence of nitrogen uptake derived from denitrification processes and NO3 − diffusive fluxes, while nitrogen fixation by diazotrophs resulted in low δ15N.
The hypothesis of a common trophic structure of the neustonic community is supported by the general niche overlap between FGs regardless of the province, although chaetognaths and detritivores generally occupied the highest and lowest trophic positions, respectively. The importance of omnivory in oligotrophic regions (the majority of provinces sampled in this study) is consolidated with our results since omnivores and carnivores exhibit varying trophic positions regardless of their nominal trophic category. Environmental conditions were depicted to influence the trophic structure of the FGs, as the size and overlap of isotopic niches were related to changes in some of the variables. Typically, FGs present smaller niche size and overlap as favorable conditions increase whereas disadvantageous conditions promote the opposite. The use of isotopic niche metrics allowed the comparison of the trophic structure of the neustonic community across the tropical and subtropical ocean, which is paramount for understanding neuston interactions and its role in pelagic food web. Further studies exploring additional FGs and/or taxonomical diversity, evaluating temporal variability and impacts on the trophic structure of the community within each province, as well as estimates of neustonic biomass, are required to better understand and improve the knowledge of the trophic structure of the neustonic community in the pelagic ocean.
Data Availability Statement
The raw data supporting the conclusions of this article will be made available by the authors, without undue reservation.
Author Contributions
CD designed, coordinated, and led the Malaspina Expedition. All authors designed the research reported. RA, AB, and JG-G performed research. RA and AB analyzed data. All authors contributed to the article and approved the submitted version.
Funding
This research was supported by project Malaspina-2010 (CSD2008-00077) funded by program CONSOLIDER-INGENIO 2010 (Ministerio de Ciencia e Innovación, Spain), by grant IN607A 2018/2 of the Axencia Galega de Innovación (GAIN, Xunta de Galicia, Spain). Thanks are also due to FCT/MCTES for the financial support to CESAM (UIDP/50017/2020+UIDB/50017/2020), through national funds. RA was supported by a Ph.D. fellowship funded by FCT (PD/BD/113483/2015).
Conflict of Interest
The authors declare that the research was conducted in the absence of any commercial or financial relationships that could be construed as a potential conflict of interest.
Acknowledgments
We are grateful to all participants in the Malaspina expedition for their collaboration in plankton sampling and to A.F. Lamas for lab assistance with sample preparation. We also acknowledge the reviewers for their constructive comments and inputs which helped to improve this manuscript.
Supplementary Material
The Supplementary Material for this article can be found online at: https://www.frontiersin.org/articles/10.3389/fmars.2020.606088/full#supplementary-material
References
Anderson, M. J., Gorley, R. N., and Clarke, K. R. (2008). Permanova+ for Primer: Guide to software and statistical methods. Plymouth, UK: PRIMER-E Ltd.
Annasawmy, P., Cherel, Y., Romanov, E. V., Le Loc’h, F., Ménard, F., Ternon, J. F., et al. (2020). Stable isotope patterns of mesopelagic communities over two shallow seamounts of the South-Western Indian Ocean. Deep Sea Res. Part II Top. Stud. Oceanogr. 176:104804. doi: 10.1016/j.dsr2.2020.104804
Baier, C. T., and Purcell, J. E. (1997). Trophic interactions of chaetognaths, larval fish, and zooplankton in the South Atlantic bight. Mar. Ecol. Prog. Ser. 146, 43–53. doi: 10.3354/meps146043
Barton, A. D., Pershing, A. J., Litchman, E., Record, N. R., Edwards, K. F., Finkel, Z. V., et al. (2013). The biogeography of marine plankton traits. Ecol. Lett. 16, 522–534. doi: 10.1111/ele.12063
Benedetti, F., Gasparini, S., and Ayata, S. D. (2016). Identifying copepod functional groups from species functional traits. J. Plankton Res. 38, 159–166. doi: 10.1093/plankt/fbv096
Benedetti, F., Vogt, M., Righetti, D., Guilhaumon, F., and Ayata, S. -D. (2018). Do functional groups of planktonic copepods differ in their ecological niches? J. Biogeogr. 45, 604–616. doi: 10.1111/jbi.13166
Bianchi, D., and Mislan, K. A. S. (2016). Global patterns of diel vertical migration times and velocities from acoustic data. Limnol. Oceanogr. 61, 353–364. doi: 10.1002/lno.10219
Bicknell, A. W. J. J., Campbell, M., Knight, M. E., Bilton, D. T., Newton, J., and Votier, S. C. (2011). Effects of formalin preservation on stable carbon and nitrogen isotope signatures in calanoid copepods: implications for the use of continuous plankton recorder survey samples in stable isotope analyses. Rapid Commun. Mass Spectrom. 25, 1794–1800. doi: 10.1002/rcm.5049
Bode, A., and Hernández-León, S. (2018). Trophic diversity of plankton in the epipelagic and mesopelagic layers of the tropical and equatorial Atlantic determined with stable isotopes. Diversity 10, 1–16. doi: 10.3390/d10020048
Bode, M., Hagen, W., Schukat, A., Teuber, L., Fonseca-Batista, D., Dehairs, F., et al. (2015). Feeding strategies of tropical and subtropical calanoid copepods throughout the eastern Atlantic Ocean ‐ latitudinal and bathymetric aspects. Prog. Oceanogr. 138, 268–282. doi: 10.1016/j.pocean.2015.10.002
Bodin, N., Budzinski, H., Le Ménach, K., and Tapie, N. (2009). ASE extraction method for simultaneous carbon and nitrogen stable isotope analysis in soft tissues of aquatic organisms. Anal. Chim. Acta 643, 54–60. doi: 10.1016/j.aca.2009.03.048
Bonnet, S., Caffin, M., Berthelot, H., and Moutin, T. (2017). Hot spot of N2 fixation in the western tropical South Pacific pleads for a spatial decoupling between N2 fixation and denitrification. Proc. Natl. Acad. Sci. U. S. A. 114, E2800–E2801. doi: 10.1073/pnas.1619514114
Bouillon, S., Connolly, R. M., and Gillikin, D. P. (2011). “Use of stable isotopes to understand food webs and ecosystem functioning in estuaries” in Treatise on estuarine and coastal science. eds. E. Wolanski and D. S. McLusky (Waltham: Elsevier Inc.), 143–173.
Brodeur, R. D. (1989). Neustonic feeding by juvenile salmonids in coastal waters of the Northeast Pacific. Can. J. Zool. 67, 1995–2007. doi: 10.1139/z89-284
Brun, P., Payne, M. R., and Kiørboe, T. (2016). Trait biogeography of marine copepods – an analysis across scales. Ecol. Lett. 19, 1403–1413. doi: 10.1111/ele.12688
Brun, P., Payne, M. R., and Kiørboe, T. (2017). A trait database for marine copepods. Earth Syst. Sci. Data 9, 99–113. doi: 10.5194/essd-9-99-2017
Burkhardt, S., Riebesell, U., and Zondervan, I. (1999). Stable carbon isotope fractionation by marine phytoplankton in response to daylength, growth rate, and CO2 availability. Mar. Ecol. Prog. Ser. 184, 31–41. doi: 10.3354/meps184031
Cabanillas-Teran, N., Hernandez-Arana, H. A., Ruiz-Zarate, M. A., Vega-Zepeda, A., and Sanchez-Gonzalez, A. (2019). Sargassum blooms in the Caribbean alter the trophic structure of the sea urchin Diadema antillarum. PeerJ 2019, 1–32. doi: 10.7717/peerj.7589
Campos, C. C., Garcia, T. M., Neumann-Leitão, S., and Soares, M. O. (2017). Ecological indicators and functional groups of copepod assemblages. Ecol. Indic. 83, 416–426. doi: 10.1016/j.ecolind.2017.08.018
Capone, D. G., Burns, J. A., Montoya, J. P., Subramaniam, A., Mahaffey, C., Gunderson, T., et al. (2005). Nitrogen fixation by Trichodesmium spp.: an important source of new nitrogen to the tropical and subtropical North Atlantic Ocean. Global Biogeochem. Cycle 19, 1–17. doi: 10.1029/2004GB002331
Carlotti, F., Pagano, M., Guilloux, L., Donoso, K., Valdés, V., and Grosso, O. (2018). Meso-zooplankton structure and functioning in the western tropical South Pacific along the 20th parallel south during the OUTPACE survey (February – April 2015). Biogeosciences 15, 7273–7297. doi: 10.5194/bg-15-7273-2018
Castellani, C., and Edwards, M. (2017). Marine plankton: A pratical guide to ecology, methodology and taxonomy. Oxford: Oxford University Press.
Cheng, L., Spear, L., and Ainley, D. G. (2010). Importance of marine insects (Heteroptera: Gerridae, Halobates spp.) as prey of eastern tropical Pacific seabirds. Mar. Ornithol. 38, 91–95.
Clark, I. D., and Fritz, P. (1997). Environmental isotopes in hydrogeology. New York: Lewis Publishers.
Coplen, T. B. (2011). Guidelines and recommended terms for expression of stable-isotope-ratio and gas-ratio measurement results. Rapid Commun. Mass Spectrom. 25, 2538–2560. doi: 10.1002/rcm.5129
de Lecea, A. M., Cooper, R., Omarjee, A., and Smit, A. J. (2011). The effects of preservation methods, dyes and acidification on the isotopic values (δ15N and δ13C) of two zooplankton species from the KwaZulu-Natal bight, South Africa. Rapid Commun. Mass Spectrom. 25, 1853–1861. doi: 10.1002/rcm.5051
DeNiro, M. J., and Epstein, S. (1978). Influence of diet on the distribution of carbon isotopes in animals. Geochim. Cosmochim. Acta 42, 495–506. doi: 10.1016/0016-7037(78)90199-0
de Oliveira Sodré, E., and Bozelli, R. L. (2019). How planktonic microcrustaceans respond to environment and affect ecosystem: a functional trait perspective. Int. Aquat. Res. 11, 207–223. doi: 10.1007/s40071-019-0233-x
Descolas-Gros, C., and Fontugne, M. (1990). Stable carbon isotope fractionation by marine phytoplankton during photosynthesis. Plant Cell Environ. 13, 207–218. doi: 10.1111/j.1365-3040.1990.tb01305.x
Duarte, C. M. (2015). Seafaring in the 21st century: the Malaspina 2010 circumnavigation expedition. Limnol. Oceanogr. Bull. 24, 11–14. doi: 10.1002/lob.10008
Ebberts, B. D., and Wing, B. L. (1997). Diversity and abundance of neustonic zooplankton in the North Pacific subarctic frontal zone. U.S. Dep. Commer., NOAA Tech. Memo. NMFS-AFSC-70, 34 p: Springfield, VA. 1–34.
Elton, C. S. (1927). “The animal community” in Animal ecology. ed. J. S. Huxley (New York: The Macmillan Company), 207.
Estrada, M., Delgado, M., Blasco, D., Latasa, M., Cabello, A. M., Benítez-Barrios, V., et al. (2016). Phytoplankton across tropical and subtropical regions of the Atlantic, Indian and Pacific oceans. PLoS One 11:e0151699. doi: 10.1371/journal.pone.0151699
Falkowski, P. G. (1997). Evolution of the nitrogen cycle and its influence on the biological sequestration of CO2 in the ocean. Nature 387, 272–275. doi: 10.1038/387272a0
Fernández-Castro, B., Mouriño-Carballido, B., Marañón, E., Chouciño, P., Gago, J., Ramírez, T., et al. (2015). Importance of salt fingering for new nitrogen supply in the oligotrophic ocean. Nat. Commun. 6, 1–10. doi: 10.1038/ncomms9002
Fiedler, P. C. (2002). The annual cycle and biological effects of the Costa Rica Dome. Deep Sea Res. Part I Oceanogr. Res. Pap. 49, 321–338. doi: 10.1016/S0967-0637(01)00057-7
Fiedler, P. C., and Talley, L. D. (2006). Hydrography of the eastern tropical Pacific: a review. Prog. Oceanogr. 69, 143–180. doi: 10.1016/j.pocean.2006.03.008
Flanders Marine Institute (2009). Longhurst Provinces. Available at: https://www.marineregions.org/ (Accessed September 10, 2020).
Francois, R., Altabet, M. A., and Goericke, R. (1993). Changes in the δ13C of surface water particulate organic matter across the subtropical convergence in the SW Indian Ocean. Glob. Biogeochem. Cycles 7, 627–644.
Fry, B. (ed.) (2006). “Scanning the future” in Stable isotope ecology. New York, NY: Springer, 277–284.
Fry, B., and Sherr, E. B. (1984). δ13C measurements as indicators of carbon flow in marine and freshwater ecosystems. Contrib. Mar. Sci. 27, 13–47.
Fry, B., and Wainright, S. C. (1991). Diatom sources of 13C-rich carbon in marine food webs. Mar. Ecol. Prog. Ser. 76, 149–157. doi: 10.3354/meps076149
González, C. E., Escribano, R., Bode, A., and Schneider, W. (2019). Zooplankton taxonomic and trophic community structure across biogeochemical regions in the Eastern South Pacific. Front. Mar. Sci. 5:498. doi: 10.3389/fmars.2018.00498
González-Gordillo, J. I., Sánchez, R., and Fernández Puelles, M. L. (2012). “Muestreo de zooplancton neustónico” in Expedición de circunnavegación Malaspina 2010. Cambio global y exploración de la biodiversidad del océano. Libro blanco de métodos y técnicas de trabajo oceanográfico. ed. E. Moreno-Ostos (Madrid: Consejo Superior de Investigaciones Científicas (CSIC)), 651–656.
Gouvêa, L. P., Assis, J., Gurgel, C. F. D., Serrão, E. A., Silveira, T. C. L., Santos, R., et al. (2020). Golden carbon of Sargassum forests revealed as an opportunity for climate change mitigation. Sci. Total Environ. 729:138745. doi: 10.1016/j.scitotenv.2020.138745
Gruber, N. (2019). A diagnosis for marine nitrogen fixation. Nature 566, 191–193. doi: 10.1038/d41586-019-00498-y
Gruber, N., Keeling, C. D., Bacastow, R. B., Guenther, P. R., Lueker, T. J., Wahlen, M., et al. (1999). Spatiotemporal patterns of carbon-13 in the global surface oceans and the oceanic Suess effect. Glob. Biogeochem. Cycles 13, 307–335. doi: 10.1029/1999GB900019
Hansen, B., Bjornsen, P. K., and Hansen, P. J. (1994). The size ratio between planktonic predators and their prey. Limnol. Oceanogr. 39, 395–403. doi: 10.4319/lo.1994.39.2.0395
Hempel, G., and Weikert, H. (1972). The neuston of the subtropical and boreal North-Eastern Atlantic Ocean. A review. Mar. Biol. 13, 70–88. doi: 10.1007/BF00351141
Hernández-León, S., Koppelmann, S. R., Fraile-Nuez, E., Bode, A., Mompeán, C., Irigoien, X., et al. (2020). Large deep-sea zooplankton biomass mirrors primary production in the global ocean. Nat. Commun. 11:6048. doi: 10.1038/s41467-020-19875-7
Holdway, P., and Maddock, L. (1983). A comparative survey of neuston: geographical and temporal distribution patterns. Mar. Biol. 76, 263–270. doi: 10.1007/BF00393027
Hooff, R. C., and Peterson, W. T. (2006). Copepod biodiversity as an indicator of changes in ocean and climate conditions of the Northern California current ecosystem. Limnol. Oceanogr. 51, 2607–2620. doi: 10.4319/lo.2006.51.6.2607
Hunt, B. P. V., Allain, V., Menkes, C., Lorrain, A., Graham, B., Rodier, M., et al. (2015). A coupled stable isotope-size spectrum approach to understanding pelagic food-web dynamics: a case study from the southwest sub-tropical Pacific. Deep Sea Res. Part II Top. Stud. Oceanogr. 113, 208–224. doi: 10.1016/j.dsr2.2014.10.023
Jackson, A. L., Inger, R., Parnell, A. C., and Bearhop, S. (2011). Comparing isotopic niche widths among and within communities: SIBER ‐ stable isotope Bayesian ellipses in R. J. Anim. Ecol. 80, 595–602. doi: 10.1111/j.1365-2656.2011.01806.x
Jónasdóttir, S. H., Visser, A. W., Richardson, K., and Heath, M. R. (2015). Seasonal copepod lipid pump promotes carbon sequestration in the deep North Atlantic. Proc. Natl. Acad. Sci. U. S. A. 112, 12122–12126. doi: 10.1073/pnas.1512110112
Kehayias, G., Lykakis, J., and Fragopoulu, N. (1996). The diets of the chaetognaths Sagitta enflata, S. serratodentata atlantica and S. bipunctata at different seasons in Eastern Mediterranean coastal waters. ICES J. Mar. Sci. 53, 837–846. doi: 10.1006/jmsc.1996.0105
Kiørboe, T. (2011). How zooplankton feed: mechanisms, traits and trade-offs. Biol. Rev. 86, 311–339. doi: 10.1111/j.1469-185X.2010.00148.x
Knapp, A. N., Casciotti, K. L., Berelson, W. M., Prokopenko, M. G., and Capone, D. G. (2016). Low rates of nitrogen fixation in Eastern tropical South Pacific surface waters. Proc. Natl. Acad. Sci. U. S. A. 113, 4398–4403. doi: 10.1073/pnas.1515641113
Lavin, M. F., and Marinone, S. G. (2003). “An overview of the physical oceanography of the Gulf of California” in Nonlinear processes in geophysical fluid dynamics. eds. O. U. Velasco Fuentes, J. Sheinbaum, and J. Ochoa (Dordrecht: Springer), 173–204.
Layman, C. A., Araujo, M. S., Boucek, R., Hammerschlag-Peyer, C. M., Harrison, E., Jud, Z. R., et al. (2012). Applying stable isotopes to examine food-web structure: an overview of analytical tools. Biol. Rev. 87, 545–562. doi: 10.1111/j.1469-185X.2011.00208.x
Layman, C. A., Arrington, D. A., Montaña, C. G., and Post, D. M. (2007a). Can stable isotope ratios provide for community-wide measures of trophic structure? Ecology 88, 42–48. doi: 10.1890/0012-9658(2007)88[42:csirpf]2.0.co;2
Layman, C. A., Quattrochi, J. P., Peyer, C. M., and Allgeier, J. E. (2007b). Niche width collapse in a resilient top predator following ecosystem fragmentation. Ecol. Lett. 10, 937–944. doi: 10.1111/j.1461-0248.2007.01087.x
Le Fevre, J., and Bourget, E. (1991). Neustonic niche for cirripede larvae as a possible adaptation to long-range dispersal. Mar. Ecol. Prog. Ser. 74, 185–194. doi: 10.3354/meps075185
Leibold, M. A. (1995). The niche concept revisited: mechanistic models and community context. Ecology 76, 1371–1382. doi: 10.2307/1938141
Li, C., Sun, S., and Wang, R. (2007). An experimental study on grazing selectivity of Calanus sinicus to natural food particles. Oceanol. Limnol. Sin. 38, 529–535.
Litchman, E., Ohman, M. D., and Kiørboe, T. (2013). Trait-based approaches to zooplankton communities. J. Plankton Res. 35, 473–484. doi: 10.1093/plankt/fbt019
Luo, Y. W., Doney, S. C., Anderson, L. A., Benavides, M., Berman-Frank, I., Bode, A., et al. (2012). Database of diazotrophs in global ocean: abundance, biomass and nitrogen fixation rates. Earth Syst. Sci. Data 4, 47–73. doi: 10.5194/essd-4-47-2012
Madigan, D. J., Carlisle, A. B., Dewar, H., Snodgrass, O. E., Litvin, S. Y., Micheli, F., et al. (2012). Stable isotope analysis challenges wasp-waist food web assumptions in an upwelling pelagic ecosystem. Sci. Rep. 2, 1–10. doi: 10.1038/srep00654
Marshall, H. G., and Burchardt, L. (2005). Neuston: its definition with a historical review regarding its concept and community structure. Arch. Hydrobiol. 164, 429–448. doi: 10.1127/0003-9136/2005/0164-0429
Mateo, M. A., Serrano, O., Serrano, L., and Michener, R. H. (2008). Effects of sample preparation on stable isotope ratios of carbon and nitrogen in marine invertebrates: implications for food web studies using stable isotopes. Oecologia 157, 105–115. doi: 10.1007/s00442-008-1052-8
McClelland, J. W., Holl, C. M., and Montoya, J. P. (2003). Relating low δ15N values of zooplankton to N 2-fixation in the tropical North Atlantic: insights provided by stable isotope ratios of amino acids. Deep Sea Res. Part I Oceanogr. Res. Pap. 50, 849–861. doi: 10.1016/S0967-0637(03)00073-6
McCutchan, J. H. Jr., Lewis, W. M. Jr., Kendall, C., and McGrath, C. C. (2003). Variation in trophic shift for stable isotope ratios of carbon, nitrogen, and sulfur. Oikos 102, 378–390. doi: 10.1034/j.1600-0706.2003.12098.x
Middelburg, J. J. (2014). Stable isotopes dissect aquatic food webs from the top to the bottom. Biogeosciences 11, 2357–2371. doi: 10.5194/bg-11-2357-2014
Minagawa, M., and Wada, E. (1984). Stepwise enrichment of 15N along food chains: further evidence and the relation between δ15N and animal age. Geochim. Cosmochim. Acta 48, 1135–1140. doi: 10.1016/0016-7037(84)90204-7
Mompeán, C., Bode, A., Benítez-Barrios, V. M., Domínguez-Yanes, J. F., Escánez, J., and Fraile-Nuez, E. (2013). Spatial patterns of plankton biomass and stable isotopes reflect the influence of the nitrogen-fixer Trichodesmium along the subtropical North Atlantic. J. Plankton Res. 35, 513–525. doi: 10.1093/plankt/fbt011
Mompeán, C., Bode, A., Gier, E., and McCarthy, M. D. (2016a). Bulk vs. amino acid stable N isotope estimations of metabolic status and contributions of nitrogen fixation to size-fractionated zooplankton biomass in the subtropical N Atlantic. Deep Sea Res. Part I Oceanogr. Res. Pap. 114, 137–148. doi: 10.1016/j.dsr.2016.05.005
Mompeán, C., Bode, A., Latasa, M., Fernández-Castro, B., Mouriño-Carballido, B., and Irigoien, X. (2016b). The influence of nitrogen inputs on biomass and trophic structure of ocean plankton: a study using biomass and stable isotope size-spectra. J. Plankton Res. 38, 1163–1177. doi: 10.1093/plankt/fbw052
Montoya, J. P., Carpenter, E. J., and Capone, D. G. (2002). Nitrogen fixation and nitrogen isotope abundances in zooplankton of the oligotrophic North Atlantic. Limnol. Oceanogr. 47, 1617–1628. doi: 10.4319/lo.2002.47.6.1617
Moreno-Ostos, E. (2012). Expedición de circunnavegación MALASPINA 2010. Madrid: Consejo Superior de Investigaciones Científicas (CSIC).
Mullin, M. M., Rau, G. H., and Eppley, R. W. (1984). Stable nitrogen isotopes in zooplankton: some geographic and temporal variations in the North Pacific. Limnol. Oceanogr. 29, 1267–1273.
Neumann-Leitão, S., Melo, P. A. M. C., Schwamborn, R., Diaz, X. F. G., Figueiredo, L. G. P., Silva, A. P., et al. (2018). Zooplankton from a reef system under the influence of the Amazon River plume. Front. Microbiol. 9:355. doi: 10.3389/fmicb.2018.00355
Olivar, M. P., Bode, A., López-Pérez, C., Hulley, P. A., Hernández-León, S., and Anderson, E. (2019). Trophic position of lanternfishes (Pisces: Myctophidae) of the tropical and equatorial Atlantic estimated using stable isotopes. ICES J. Mar. Sci. 76, 649–661. doi: 10.1093/icesjms/fsx243
Owens, N. J. P. (1988). Natural variations in 15N in the marine environment. Adv. Mar. Biol. 24, 389–451. doi: 10.1016/S0065-2881(08)60077-2
Padmavati, G., and Goswami, S. C. (1996). Zooplankton distribution in neuston and water column along west coast of India from Goa to Gujarat. Indian J. Mar. Species 25, 85–90.
Pearre, S., and Pearre, J. S. (1982). Feeding by Chaetognatha: aspects of inter‐ and intra-specific predation. Mar. Ecol. Prog. Ser. 7, 33–45.
Perry, R. I., Thompson, P. A., Mackas, D. L., Harrison, P. J., and Yelland, D. R. (1999). Stable carbon isotopes as pelagic food web tracers in adjacent shelf and slope regions off British Columbia, Canada. Can. J. Fish. Aquat. Sci. 56, 2477–2486. doi: 10.1139/cjfas-56-12-2477
Peterson, B. J., and Fry, B. (1987). Stable isotopes in ecosystem studies. Source Annu. Rev. Ecol. Syst. 18, 293–320. doi: 10.1146/annurev.ecolsys.18.1.293
Pomerleau, C., Sastri, A. R., and Beisner, B. E. (2015). Evaluation of functional trait diversity for marine zooplankton communities in the Northeast subarctic Pacific Ocean. J. Plankton Res. 37, 712–726. doi: 10.1093/plankt/fbv045
Popp, B. N., Hanson, K. L., Dore, J. E., Bidigare, R. R., Laws, E. A., and Wakeham, S. G. (1999). “Controls on the carbon isotopic composition of phytoplankton” in Reconstructing Ocean history: A window into the future. eds. F. Abrantes and A. C. Mix (New York, NY: Springer), 381–398.
Post, D. M. (2002a). The long and short of food-chain length. Trends Ecol. Evol. 17, 269–277. doi: 10.1016/S1097-2765(02)00657-3
Post, D. M. (2002b). Using stable isotopes to estimate trophic position: models, methods, and assumptions. Ecology 83, 703–718. doi: 10.2307/3071875
Post, D. M., Layman, C. A., Arrington, D. A., Takimoto, G., Quattrochi, J., and Montaña, C. G. (2007). Getting to the fat of the matter: models, methods and assumptions for dealing with lipids in stable isotope analyses. Oecologia 152, 179–189. doi: 10.1007/s00442-006-0630-x
Prowe, A. E. F., Visser, A. W., Andersen, K. H., Chiba, S., and Kiørboe, T. (2019). Biogeography of zooplankton feeding strategy. Limnol. Oceanogr. 64, 661–678. doi: 10.1002/lno.11067
Rau, G. H., Ohman, M. D., and Pierrot-Bults, A. (2003). Linking nitrogen dynamics to climate variability off Central California: a 51 year record based on 15N/14N in CalCOFI zooplankton. Deep Sea Res. Part II Top. Stud. Oceanogr. 50, 2431–2447. doi: 10.1016/S0967-0645(03)00128-0
Rawlinson, K. A., Davenport, J., and Barnes, D. K. A. (2005). Temporal variation in diversity and community structure of a semi-isolated neuston community. Biol. Environ. Proc. R. Irish Acad. 105B, 107–122. doi: 10.3318/bioe.2005.105.2.107
R Core Team (2020). R: A Language and Environment for Statistical Computing. R Foundation for Statistical Computing, Vienna, Austria. Available at: https://www.R-project.org/ (Accessed September 10, 2020).
Rezai, H., Kabiri, K., Arbi, I., and Amini, N. (2019). Neustonic zooplankton in the northeastern Persian Gulf. Reg. Stud. Mar. Sci. 26:100473. doi: 10.1016/j.rsma.2018.100473
Schmidt, S. N., Olden, J. D., Solomon, C. T., and Vander Zanden, M. J. (2007). Quantitative approaches to the analysis of stable isotope food web data. Ecology 88, 2793–2802. doi: 10.1890/07-0121.1
Schmittner, A., Gruber, N., Mix, A. C., Key, R. M., Tagliabue, A., and Westberry, T. K. (2013). Biology and air-sea gas exchange controls on the distribution of carbon isotope ratios (δ13C) in the ocean. Biogeosciences 10, 5793–5816. doi: 10.5194/bg-10-5793-2013
Somes, C. J., Schmittner, A., Galbraith, E. D., Lehmann, M. F., Altabet, M. A., Montoya, J. P., et al. (2010). Simulating the global distribution of nitrogen isotopes in the ocean. Glob. Biogeochem. Cycles 24, 1–16. doi: 10.1029/2009GB003767
Sommer, F., Hansen, T., and Sommer, U. (2006). Transfer of diazotrophic nitrogen to mesozooplankton in Kiel Fjord, Western Baltic Sea: a mesocosm study. Mar. Ecol. Prog. Ser. 324, 105–112. doi: 10.3354/meps324105
Stasko, A. D., Bluhm, B. A., Michel, C., Archambault, P., Majewski, A., Reist, J. D., et al. (2018). Benthic-pelagic trophic coupling in an Arctic marine food web along vertical water mass and organic matter gradients. Mar. Ecol. Prog. Ser. 594, 1–19. doi: 10.3354/meps12582
Stukel, M. R., Décima, M., Landry, M. R., and Selph, K. E. (2018). Nitrogen and isotope flows through the Costa Rica Dome upwelling ecosystem: the crucial mesozooplankton role in export flux. Glob. Biogeochem. Cycles 32, 1815–1832. doi: 10.1029/2018GB005968
Svanbäck, R., Quevedo, M., Olsson, J., and Eklöv, P. (2015). Individuals in food webs: the relationships between trophic position, omnivory and among-individual diet variation. Oecologia 178, 103–114. doi: 10.1007/s00442-014-3203-4
Taylor, A. G., Landry, M. R., Freibott, A., Selph, K. E., and Gutierrez-Rodríguez, A. (2015). Patterns of microbial community biomass, composition and HPLC diagnostic pigments in the Costa Rica upwelling dome. J. Plankton Res. 38, 183–198. doi: 10.1093/plankt/fbv086
Tortell, P. D., and Morel, F. M. M. (2002). Sources of inorganic carbon for phytoplankton in the eastern subtropical and equatorial Pacific Ocean. Limnol. Oceanogr. 47, 1012–1022. doi: 10.4319/lo.2002.47.4.1012
Tuomisto, H. (2010). A diversity of beta diversities: straightening up a concept gone awry. Part 1. Defining beta diversity as a function of alpha and gamma diversity. Ecography 33, 2–22. doi: 10.1111/j.1600-0587.2009.05880.x
Turner, J. T. (2002). Zooplankton fecal pellets, marine snow and sinking phytoplankton blooms. Aquat. Microb. Ecol. 27, 57–102. doi: 10.3354/ame027057
Vander Zanden, M. J., Clayton, M. K., Moody, E. K., Solomon, C. T., and Weidel, B. C. (2015). Stable isotope turnover and half-life in animal tissues: a literature synthesis. PLoS One 10:e0116182. doi: 10.1371/journal.pone.0116182
Vander Zanden, M. J., and Rasmussen, J. B. (2001). Variation in δ15N and δ13C trophic fractionation: implications for aquatic food web studies. Limnol. Oceanogr. 46, 2061–2066. doi: 10.4319/lo.2001.46.8.2061
Violle, C., Navas, M. -L., Vile, D., Kazakou, E., Fortunel, C., Hummel, I., et al. (2007). Let the concept of trait be functional! Oikos 116, 882–892. doi: 10.1111/j.2007.0030-1299.15559.x
Wang, W. L., Moore, J. K., Martiny, A. C., and Primeau, F. W. (2019). Convergent estimates of marine nitrogen fixation. Nature 566, 205–211. doi: 10.1038/s41586-019-0911-2
Wannicke, N., Korth, F., Liskow, I., and Voss, M. (2013). Incorporation of diazotrophic fixed N2 by mesozooplankton — case studies in the Southern Baltic Sea. J. Mar. Syst. 117–118, 1–13. doi: 10.1016/j.jmarsys.2013.03.005
Waser, N. A. D., Harrison, W. G., Head, E. J. H., Nielsen, B., Lutz, V. A., Calvert, E., et al. (2000). Geographic variations in the nitrogen isotope composition of surface particulate nitrogen and new production across the North Atlantic Ocean. Deep Sea Res. Part I Oceanogr. Res. Pap. 47, 1207–1226. doi: 10.1016/S0967-0637(99)00102-8
Wong, W. W., and Sackett, W. M. (1978). Fractionation of stable carbon isotopes by marine phytoplankton. Geochim. Cosmochim. Acta 42, 1809–1815. doi: 10.1016/0016-7037(78)90236-3
Zaitsev, Y. P. (1971). Marine Neustonology. ed. K. A. Vinogradov (Jerulasem: Israel program for scientific translations).
Zehr, J. P., Jenkins, B. D., Short, S. M., and Steward, G. F. (2003). Nitrogenase gene diversity and microbial community structure: a cross-system comparison. Environ. Microbiol. 5, 539–554. doi: 10.1046/j.1462-2920.2003.00451.x
Keywords: stable isotopes, trophic groups, neuston, biogeochemical provinces, niche size
Citation: Albuquerque R, Bode A, González-Gordillo JI, Duarte CM and Queiroga H (2021) Trophic Structure of Neuston Across Tropical and Subtropical Oceanic Provinces Assessed With Stable Isotopes. Front. Mar. Sci. 7:606088. doi: 10.3389/fmars.2020.606088
Edited by:
Xavier Pochon, Cawthron Institute, New ZealandReviewed by:
Christopher Somes, GEOMAR Helmholtz Center for Ocean Research Kiel, GermanyEleonora Puccinelli, Université de Bretagne Occidentale, France
Copyright © 2021 Albuquerque, Bode, González-Gordillo, Duarte and Queiroga. This is an open-access article distributed under the terms of the Creative Commons Attribution License (CC BY). The use, distribution or reproduction in other forums is permitted, provided the original author(s) and the copyright owner(s) are credited and that the original publication in this journal is cited, in accordance with accepted academic practice. No use, distribution or reproduction is permitted which does not comply with these terms.
*Correspondence: Rui Albuquerque, rui.ferreira.albuquerque@gmail.com
†ORCID: Antonio Bode orcid0000-0002-9535-254