- Department of Health and Pharmaceutical Sciences, School of Pharmacy, Universidad San Pablo-CEU, CEU Universities, Madrid, Spain
SAICEUPSMT strain was isolated from soils in the mining district of Almadén (Ciudad Real, Spain), subjected to a high concentration of mercury. Using the plant model of lupinus, the strain was inoculated into the rhizosphere of the plant in a soil characterized by a high concentration of mercury (1,710 ppm) from an abandoned dump in the mining district of Almadén (Ciudad Real, Spain). As a control, a soil with a minimum natural concentration of mercury, from a surrounding area, was used. Under greenhouse conditions, the effect that the inoculum of the SAICEUPSMT strain had on the antioxidant capacity of the plant was studied, through the quantification of the enzymatic activity catalase (CAT), ascorbate peroxidase (APX), superoxide dismutase (SOD), and glutathione reductase (GR). Likewise, the capacity of the plant to bioaccumulate mercury in the presence of the inoculum was studied, as well as the effect on the biometric parameters total weight (g), shoot weight (g), root weight (g), shoot length (cm), root length (cm), total number of leaves (N), and total number of secondary roots (No). Finally, in view of the results, the SAICEUPSMT strain was identified from the phenotypic and genotypic point of view (housekeeping genes and complete genome sequencing). The inoculum with the SAICEUPSMT strain in the presence of mercury produced a significant reduction in the enzymatic response to oxidative stress (CAT, APX, and SOD). It can be considered that the strain exerts a phytoprotective effect on the plant. This led to a significant increase in the biometric parameters total plant weight, root weight and the number of leaves under mercury stress, compared to the control without abiotic stress. When analyzing the mercury content of the plant with and without bacterial inoculum, it was found that the incorporation of the SAICEUPSMT strain significantly reduced the uptake of mercury by the plant, while favoring its development in terms of biomass. Given the positive impact of the SAICEUPSMT strain on the integral development of the plant, it was identified, proving to be a Gram negative bacillus, in vitro producer of siderophores, auxins and molecules that inhibit stress precursors. The most represented fatty acids were C16:0 (33.29%), characteristic aggregate 3 (22.80%) comprising C16:1 ω7c and C16: 1ω6c, characteristic aggregate 8 (13.66%) comprising C18:1 ω7c, and C18: 1 cycle ω6c and C 17:0 (11.42%). From the genotypic point of view, the initial identification of the strain based on the 16S rRNA gene sequence classified it as Pseudomonas iranensis. However, genome-wide analysis showed that average nucleotide identity (ANI, 95.47%), DNA-DNA in silico hybridization (dDDH, 61.9%), average amino acid identity (AAI, 97.13%), TETRA (0.99%) and intergenic distance (0.04) values were below the established thresholds for differentiation. The results of the genomic analysis together with the differences in the phenotypic characteristics and the phylogenetic and chemotaxonomic analysis support the proposal of the SAICEUPSMT strain as the type strain of a new species for which the name Pseudomonas mercuritolerans sp. is proposed. No virulence genes or transmissible resistance mechanisms have been identified, which reveals its safety for agronomic uses, under mercury stress conditions.
Introduction
Up to 9,000 tons of mercury are released annually into the atmosphere, water, and soils (Zhang et al., 2021). Mercury is a global pollutant that causes damage to the environment and can affect animals and people by its transmission through the food chain. Therefore, it has the potential to become a public health and environmental threat (Ballabio et al., 2021). For this reason, there is a growing scientific, technical, and social interest in reducing mercury pollution and mitigating its effects. Bioremediation techniques have proven to be an effective and environmentally friendly alternative (Bhatt et al., 2021) as well as useful for the recovery of high land extensions (Dary et al., 2010). In situ metal phytostabilization is a phytoremediation technique that uses metal-tolerant plants for the mechanical stabilization of the contaminant, preventing its transport to other environments by leaching or air transport. In addition, it reduces the accumulation of pollutants in biological systems, such as plants. Mercury is a heavy metal that has no biological role in plants or animals. For this reason, it tends to bioaccumulate, replacing other metabolically active metals in the body and triggering numerous diseases (Ballabio et al., 2021). Phytostabilization therefore sequesters contaminants in the soil environment in a more cost-effective way, especially in the case of extensive contamination. For this purpose, the use of fast-growing forage plants, such as the Lupinus genus, may be useful.
Phytostabilization process can be improved with the use of microorganisms with the ability to degrade contaminants and/or with the ability to promote plant growth (PGPB, plant growth promoting bacteria) under stress conditions (Kuiper et al., 2004; Azaroual et al., 2022). Traditionally, PGPB were used primarily to help plants absorb nutrients from the environment or to prevent plant diseases, in both cases by promoting their development.
Despite the natural potential of plants to remove heavy metals from the soil, phytoremediation is yet to become a commercially available technology. For this reason, it seems interesting to investigate beneficial plant-microorganism associations that improve the efficiency of the phytoremediation process. The Pseudomonas genus is varied and has great versatility. Many of its species can colonize different niches, due to their metabolic capacity and their ease of adaptation to different conditions (Bravakos et al., 2021). The association with plants is enhanced by the secretion of phytohormones (auxins, gibberellins, etc.), secondary metabolites (flavonoids) and enzymes (aminocyclopropane-1-carboxylate, phenylalanine ammonia-lyase) as well as siderophores, nitrogen fixation, sulfate solubilization, antibiotic production, induced systemic resistance (Sah et al., 2021; Consentino et al., 2022; Mellidou and Karamanoli, 2022), and phytopathogens control (Zhang et al., 2022). SAICEUPSMT was isolated from the rhizosphere of a Medicago sativa plant native to the Almadén mining district. Specifically, it was isolated from a dump slope, where the mercury concentration was 1,071 ppm (Millán et al., 2007). Due to its ability to promote plant growth under conditions of abiotic stress, the strain was identified, and it was verified that both bioinformatics and phenotypic analysis suggest that it may be a new strain, reason why Pseudomonas mercuritolerans is proposed as its new taxonomic classification.
The aim of this work is the evaluation of the in vivo mercury phytostabilization potential under greenhouse conditions of Lupinus albus var., Orden Dorado plants in association with mercury resistant SAICEUPSMT strain in soil substrates from the Almadén mining district (Ciudad Real, Spain).
Results
Isolation, identification, and phylogenetic analysis
Strain SAICEUPSMT isolated from the rhizosphere of a Medicago sativa plant native to the Almadén mining district was classed as a Gram negative rod-shaped bacterium forming translucent brown colonies that intensify their hue after 72 h, ≈ 1 mm ∅, with smooth borders, and creamy texture. It grows in nutritive agar at 28°C, with aerobic metabolism, oxidase catalase positive, non-endospore-forming. Its size is 624.1–778.7 μm wide and 1.679–2.489 μm long (Supplementary Figure 1). These characteristics identify SAICEUPSMT as belonging to the Pseudomonas genus.
The similarity analysis using the 16S rRNA gene showed a value of 96.60% against P. iranensis SWRI54. All other strains analyzed showed lower similarity indices (Table 1).
Whole genome sequence (WGS) analysis revealed that average nucleotides identity value (ANI) value against P. iranensis SWRI54 was 95.34%. In the same way, the average amino acids identity (AAI) offered a value of 97.04%. For this reason, it cannot be concluded that the SAICEUPSMT strain belongs to P. iranensis SWRI54. According to the of the CLSI identification criteria (Clinical and Laboratory Standards Institute) strain SAICEUPSMT could be a new species within the Pseudomonas genus. The phylogenetic activity of Genome Blast Distance Phylogeny (GBDP) (Figure 1) positioned SAICEUPSMT within the cluster of P. siliginis SWRI31, P. atacamensis M7D1, P. iranensis SWRI54 and P. triticicola SWRI88. SAICEUPSMT is segregated from the species in its cluster. The threshold used to discriminate nearby species is between 95–96% for ANI and 70% for DNA-DNA hybridization in silico (dDDH) (Richter and Rosselló-Móra, 2009). The analysis of dDDH shows that the highest value for SAICEUPSMT is below this threshold (Table 2). The ANI analysis was 95.42%. These data confirm that the SAICEUPSMT strain does not belong to any of these species (Supplementary Table 1: Calculated ANIb values, Supplementary Table 2: Calculated Tetra values, and Supplementary Table 3: Intergenomic distance).
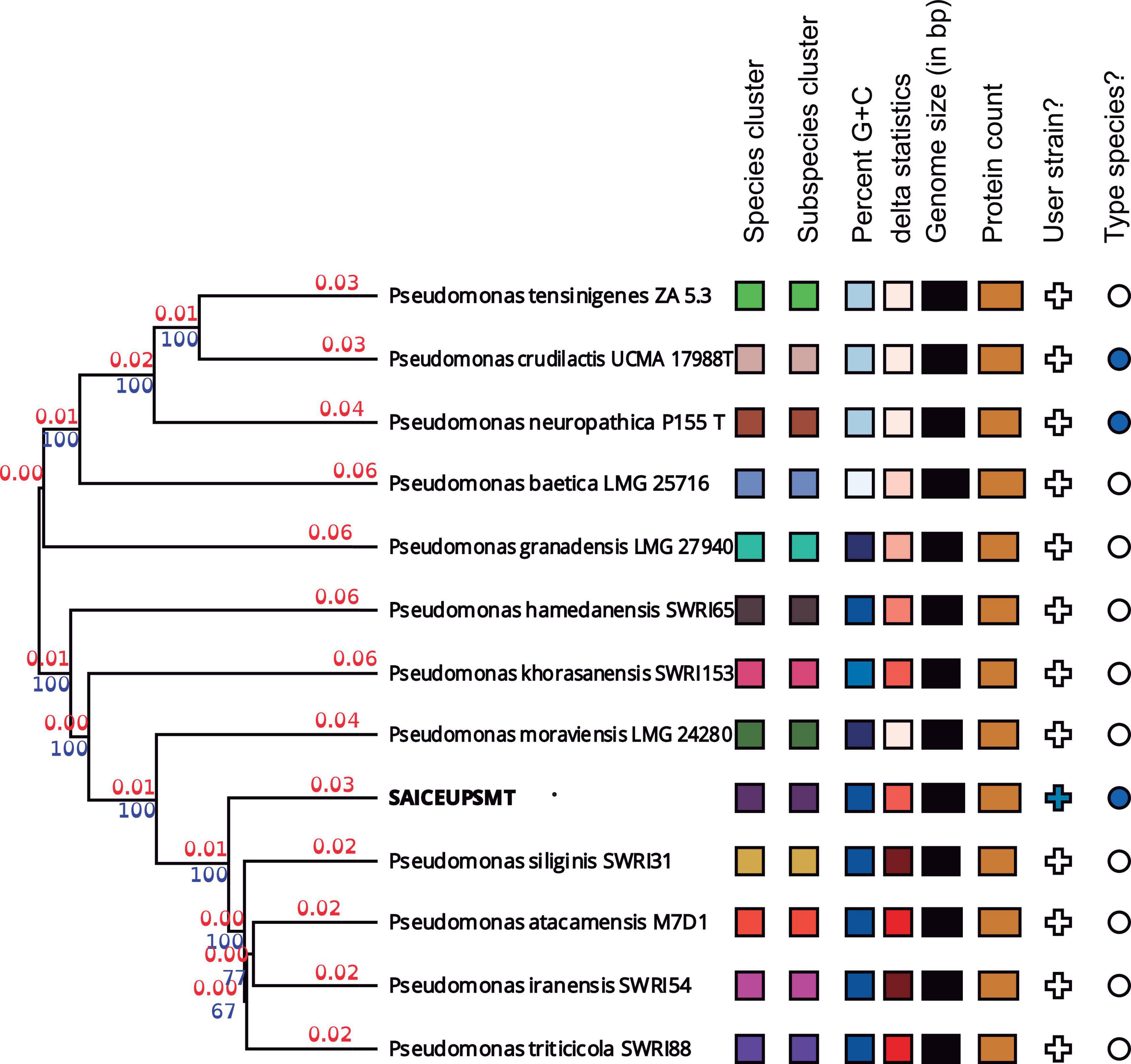
Figure 1. Phylogenetic tree from the complete genome of the species of the genus Pseudomonas. Numerical values indicate the relative distance between the analyzed species. The branch lengths are scaled in terms of Genome Blast Distance Phylogeny (GBDP) d5. The blue numbers are GBDP pseudo-bootstrap support values >60% from 100 replications, with an average branch support of 55.2%. The branch length values (in red) represent the evolutionary time between two nodes. Unit: substitutions per sequence site. The tree was rooted at the midpoint.
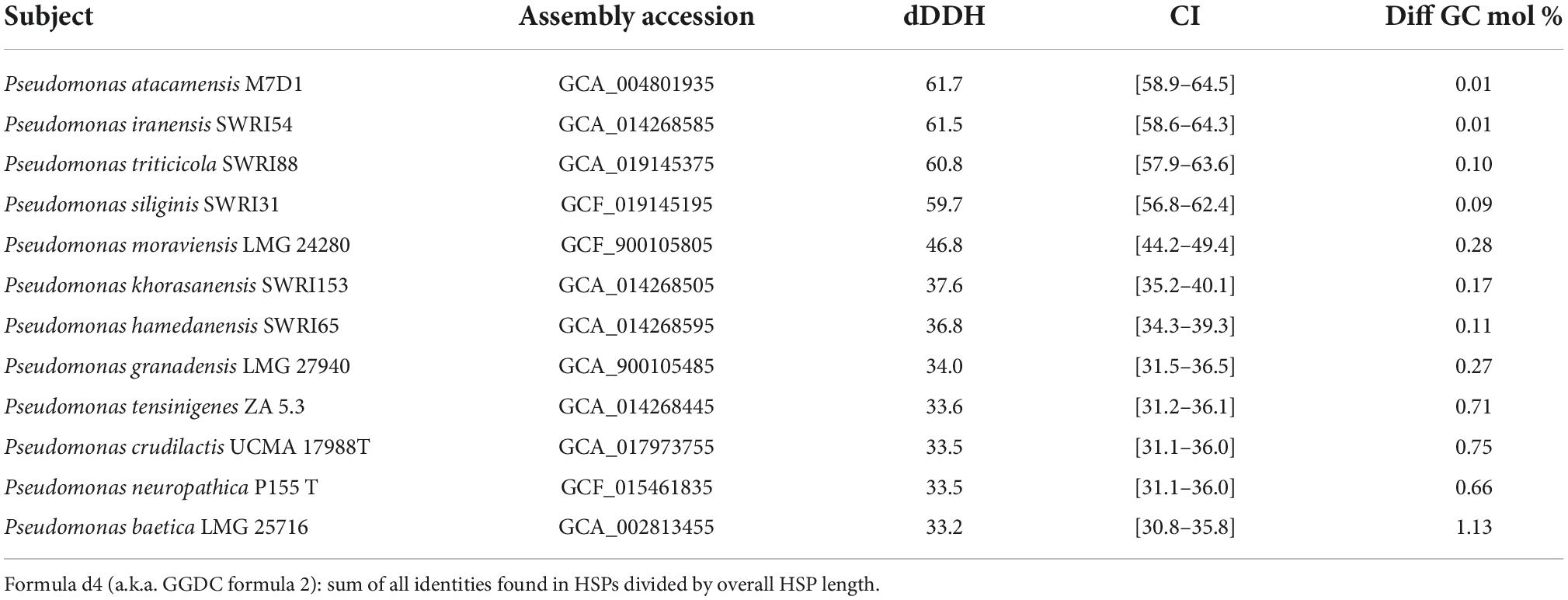
Table 2. DNA-DNA hybridization in silico (dDDH), confidence interval (CI), and GC percentage difference between SAICEUPSMT and Pseudomonas spp. closely related.
Phenotypic description and plant growth promotion activities characterization
This strain is a siderophores producer and presents the ability to degrade ethylene precursor 1-aminocyclopropane-1-carboxylate deaminase (ACC) via enzyme ACC deaminase (ACCd). Additionally, produces the auxin class phytohormone Indole-3-acetic acid (IAA, 7.72 μg.ml–1). Metabolizes citrate, L-Proline aryl amidase and hydrolyzes gelatin, beta hemolytic, and urea transformer. It was negative for the motility, production of acetoin, fermentation of dulcitol, deamination of phenylalanine, production of indole, hydrogen sulfide, decarboxylation of ornithine, lysine, arginine, sucrose, xylose, maltose, mannitol, citrate, and lactose. Table 3 shows different phenotypic characteristics compared to the species with greater phylogenetic proximity.
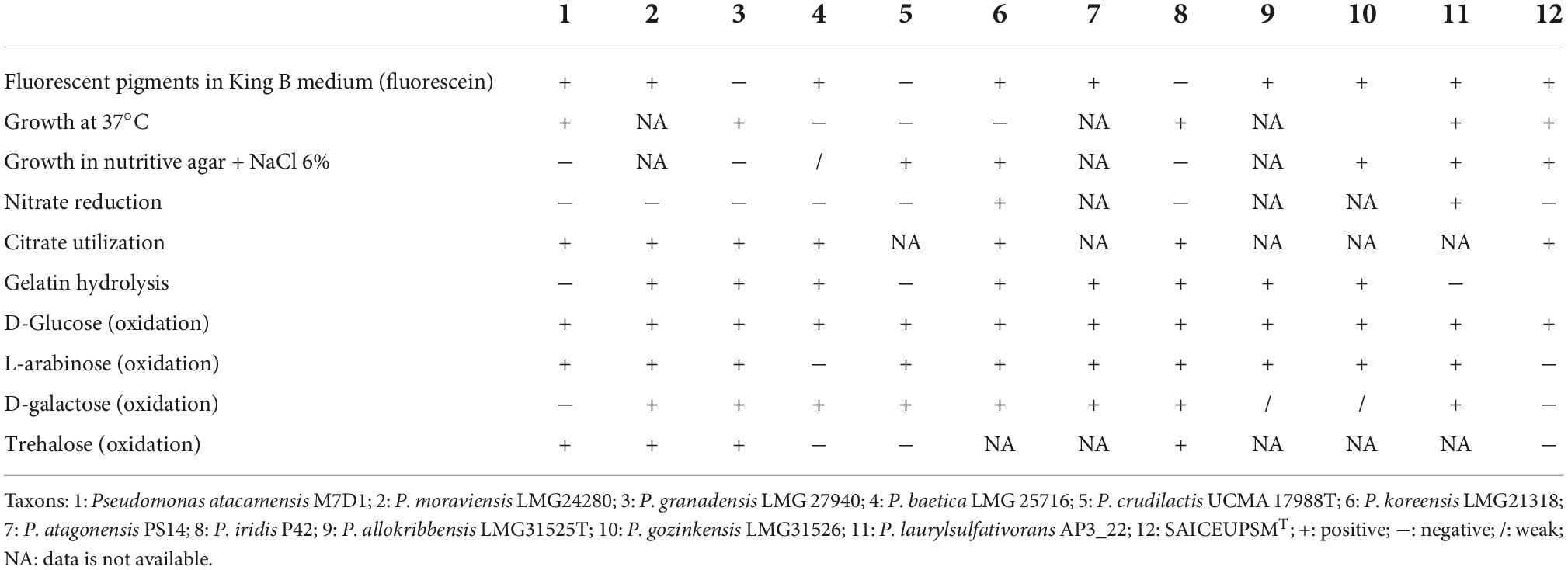
Table 3. Comparison of the phenotype of SAICEUPSMT with its closest species according to its DNA-DNA hybridization in silico (dDDH).
The bacterium can grow at concentrations between 0 and 6% (p/v) of NaCl, the optimal pH is between 5.5 and 8.0 and within the temperature range of 4 to 37°C, being 28°C its optimal growth temperature. The minimum inhibitory concentrations (MIC) against different antibiotics are shown in Supplementary Table 4.
Regarding the resistance of the strain against heavy metals, quantified by calculating the minimum bactericidal concentration (MBC), SAICEUPSMT strain was highly resistant to mercury (140 ppm), cupper (400 ppm), chrome (800 ppm), and nickel (400 ppm). In contrast, the cadmium tolerance capacity was 12.5 ppm.
Chemotaxonomic analysis
The analysis by mass spectrophotometry measured by matrix-assisted laser desorption/ionization–time of flight (MALDI-TOF) generated a list of 10 peaks based on their intensity, which allowed a peptide fingerprint and its comparison with the database (Supplementary Figure 2). The analysis of this profile showed a homologation between SAICEUPSMT with P. fluorescens.
The analysis of fatty acids showed the best represented molecules: C16:0 (35.59%), Sum in Feature 3 (18.21%), C17:0 cyclo (16.73%), and Sum in Feature 8 (10.78%). The profile described by SAICEUPSMT does not allow the identification of the strain with any nearby species (Table 4).
Genome features
The SAICEUPSMT genome was formed from 192 contigs, with a genome length of 6,312,264 bp. The GC content was 60.07% mol (Supplementary Figure 3A). A total of 5,522 CDS were identified and assigned to 27 subsystems through the Rapid using Subsystem Technology (RAST) SEED viewer (Supplementary Table 5 and Supplementary Figure 3B), achieving the score of 42% (2337) and through the KEGG analysis 44%. The most represented subsystems were amino acids and derivatives (481), carbohydrates (262), protein metabolism (220), cofactors, vitamins, protein, and pigments (206). The genome sequence project was deposited in NCBI (Bioproject PRJNA847155; Biosample SAMN28920853).
KofamKOALA analyses show that almost all the bacteria’s major metabolic pathways were found in SAICEUPSMT genome (Supplementary Figure 4A and Supplementary Table 6). An in-depth analysis of the mechanisms that could support the phenotypic result of resistance to mercury revealed that SAICEUPSMT had genes from the mercury resistance operon, finding transport genes (mercuric transport protein, merT and mercuric transport protein, merC) and reduction genes (Mercuric ion reductase, merA and Organomercurial lyase, merB) (Supplementary Figure 4B). Likewise, the existence of genes that confer resistance to other heavy metals (cadmium, cupper, chrome, nickel), was analyzed to reinforce the phenotypic observations. The results of the whole gene collection can be consulted extensively in Supplementary Table 7.
The list of genes that code for mechanisms of antibiotic resistance are shown in Supplementary Table 8. Likewise, Supplementary Tables 9, 10 show the genes involved in the main virulence factors: biosynthesis of flagella proteins, adhesion motility, endotoxin, type IV pili, adherence, contraction rate, ion uptake, antiphagocytosis, and secretion systems Type I, Type II, Type III, and Type VI. These operons are incomplete, which is why the strain is not able to express them functionally. The software of the Center for Genomic Epidemiology predicted that the organism does not present a risk of pathogenesis.
In Supplementary Table 11, genes associated with direct and indirect mechanisms of plant growth promoters in SAICEUPSMT are collected.
Plant growth promotion
The ANOVA analysis of the biometric variables of those plants inoculated with SAICEUPSMT, revealed the existence of significant differences (p-value < 0.05) in the parameters total weight (Weigth_T, Figure 2A), root weight (Weight_R, Figure 2B), and number of leaves (Leaves, Figure 2C) compared to the controls. The SAICEUPSMT strain significantly contributed to a higher plant weight, as well as a greater development of the root with respect to the control.
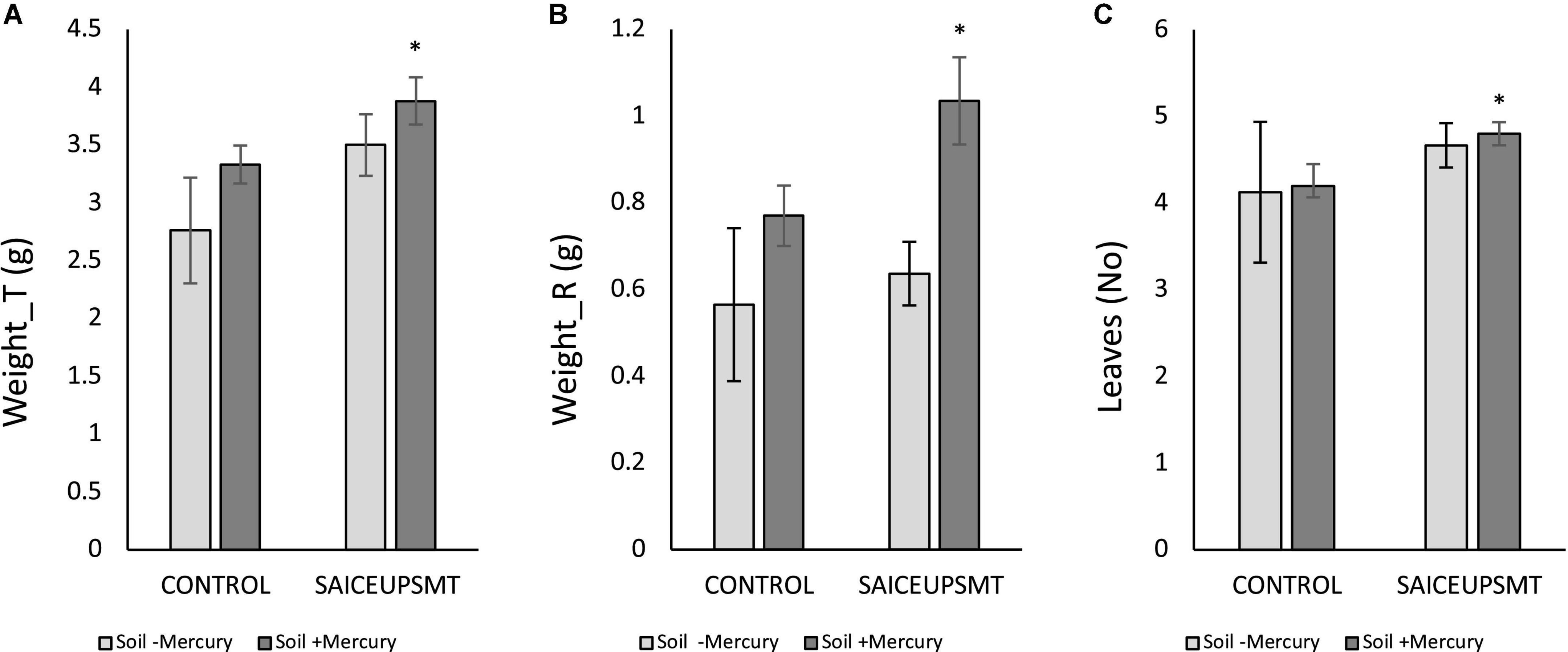
Figure 2. Results of the least significance difference (LSD) analysis of the biometric parameters of plants grown in soils with mercury. (A) Total weight, (B) root weight, and (C) total number of leaves. The bars indicate a standard error. *p-value ≤ 0.05. s/u, no units.
Phytoprotection
To verify the potential oxidative stress protection effect SAICEUPSMT strain under mercury stress conditions, the enzymatic response of Lupinus albus grown in soil with a high concentration of the heavy metal was evaluated. Figure 3 shows quantification of catalase (CAT), superoxide dismutase (SOD), ascorbate peroxidase (APX), and glutathione reductase (GR) enzymes. Incorporating the strain into the root promotes a significant reduction in enzymatic response.
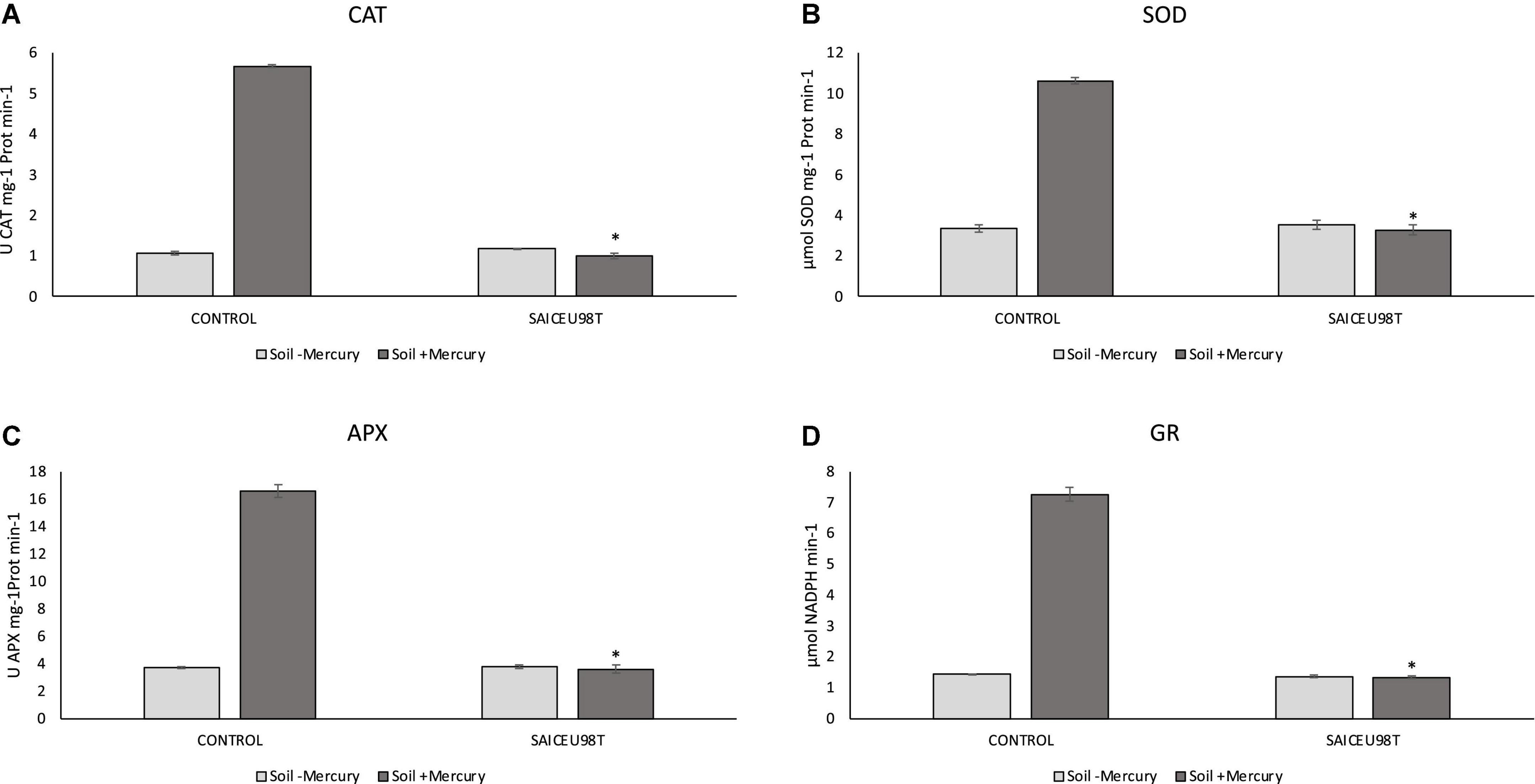
Figure 3. Results of Kruskal–Wallis for enzymatic content: (A) catalase (CAT), (B) superoxide dismutase (SOD), (C) ascorbate peroxidase (APX), and (D) glutathione reductase (GR). The bars indicate the standard error. *p-value ≤ 0.001.
Mercury accumulation in plant
To evaluate the potential mercury phytoprotective capacity of SAICEUPSMT, the physiological content of this heavy metal in the plant was analyzed (Table 5). It is observed that Lupinus albus tends to bioaccumulate mercury mainly at the root level. This accumulation is not statistically different in inoculated plants. The incorporation of SAICEUPSMT, promoted a significant decrease in the accumulation of mercury in the shoot of the plant, respect the control.
Discussion
Lupinus albus model has shown to be a plant with high tolerance to abiotic stress (Fumagalli et al., 2014) as well as having an interesting phytoextraction capacity (Zornoza et al., 2010; Godínez-Méndez et al., 2021; Quiñones et al., 2021). However, mercury is also known to induce physiological and metabolic alterations in plants, as the enzymatic response against oxidative stress (Zelles, 1999; Christakis et al., 2021; Çavus̨oğlu et al., 2022) and decreased plant growth. Conversely, the effect of PGPB inoculation may minimize these effects on plants (Heidari and Golpayegani, 2012; Morcillo and Manzanera, 2021) in mercury-contaminated substrates (Pirzadah et al., 2018). The Pseudomonas genus, precisely, has shown in previous works its important role as PGPB in stress conditions (Sandhya et al., 2010). In those plants inoculated with SAICEUPSMT, the biometric parameters total weight, root weight and number of leaves were significantly higher in soils with high mercury concentration, compared to the same growth conditions, in the absence of PGPB inoculum. The identification of genes coding for plant growth promotion (PGP) activities explains the phenotype of the bacterium. This fact has already been described in other Pseudomonas species (Chellaiah, 2018; Kang et al., 2020).
From the results of this work, we confirm that, inoculation with SAICEUPSMT strain under mercury stress produces a significant reduction in the enzymatic response to oxidative stress (CAT, APX, and SOD). SOD enzyme catalyzes the transformation of singlet oxygen to H2O2, with CAT and APX enzymes being responsible for eliminating H2O2 by transforming it into H2O and O2 (Gill and Tuteja, 2010). Similarly, GR is involved in reducing glutathione disulfide (GSSG) to glutathione (GSH) with NADPH expenditure. GSH plays a very important role in the redox regulation of the cell cycle and in the defense mechanisms against oxidative stress (Sánchez-Fernández et al., 1997). Plants grown in mercury contaminated soils and inoculated with SAICEUPSMT have also significantly lower GR production. Root colonization by SAICEUPSMT produces a phytoprotection effect against mercury stress by inducing a better antioxidant enzymatic response, compared to the non-inoculated controls.
Plants of different species, like Lupinus albus, can accumulate mercury in different tissues, but the absorption mechanism is still unknown (Zornoza et al., 2010; Quiñones et al., 2021; Robas et al., 2021). This fact can induce a reduction in the production of plant biomass. On the contrary, plants inoculated with SAICEUPSMT can increase the weight and number of leaves of plants, even in substrates with high concentration of mercury. The incorporation of SAICEUPSMT significantly reduces the absorption of mercury by the plant, while favoring its development in terms of biomass. That is why we can speak of a phytoprotective effect, which can be associated with the transformation of mercury carried out by the bacteria in the root environment, due to the expression of the genes involved in the metabolism of this heavy metal mer (operon). This fact is key for future agronomic applications of the strain, since the improvement in production can be significant, even under conditions of high concentration of mercury, and bioremediation processes. All of this, under safe (environmental and health) conditions since the innocuousness of the SAICEUPSMT strain has been demonstrated as it lacks functional virulence factors and transmissible or clinically relevant resistance genes. For all the above, the SAICEUPSMT strain is postulated as a new taxon not described to date, called P. mercuritolerans, with potential use in improving plant yield under abiotic stress conditions and in bioremediation processes of heavy metals, such as mercury.
The growing soil heavy metal pollution in natural environments has increased scientific interest in finding alternatives for the decontamination of these ecosystems. To this end, the participation of PGPB can contribute effectively, both directly on the soil and indirectly, by contributing to the phytoprotection of plants (Singh et al., 2019). The Pseudomonas genus can grow and develop under unfavorable conditions, metabolize a wide variety of organic and inorganic compounds, as well as reduce and volatilize heavy metals (Beltrán-Pineda and Gómez-Rodríguez, 2016). SAICEUPSMT strain has functional genes related to the reduction and volatilization of mercury, resistance to copper, cobalt, zinc, cadmium, as well as siderophores production capacity that help improve tolerance or transform ions of toxic metals, minimizing their toxic effects (Singh and Hiranmai, 2021), which could justify the high resistance values obtained in laboratory tests. There are few bibliographical references that review the tolerance of different species of the Pseudomonas genus to mercury. However, the MBC values found in this work highlight the unique resistance of SAICEUPSMT to said heavy metal. Specifically, phenotypically, the strain ceased to be viable at a concentration of 140 ppm (516.6 μm HgCl2). Sahu et al. (2016) isolated a strain that they considered to be highly tolerant to mercury isolated from an industrial effluent, capable of withstanding up to 200 μm HgCl2 (i.e., almost 2.5 times less than SAICEUPSMT). In this same sense, Zhang et al. (2012) isolated from a marine sample, a strain of P. putida with a tolerance to mercury like the previous authors, of 280 μm, also a much lower value than SAICEUPSMT. This makes it an excellent candidate for the design of subsequent bioremediation processes for soils contaminated by mercury. The capacity of the strain to resist other heavy metals remains uniquely high, well above values found by other authors (Matyar et al., 2010; Singh et al., 2010) in the Pseudomonas genus.
Phenotypic and genotypic characterization techniques allow a multiphase approach of the strains with biotechnological interest. SAICEUPSMT activity and the set of metabolic tests allowed it to be differentiated from nearby species, such as P. atacamensis M7D1. Fatty acid analysis has also been commonly used to identify species and describe new taxa (Cody et al., 2015; Heir et al., 2021). Unfortunately, this method does not always allow accurate identification. Despite the existence of undescribed profiles, the lack of homology makes us think of the possibility of the discovery of a new taxon.
Initially, the analysis of 16S rRNA, a basic tool for classifying bacterial species, showed a similarity of 96.60% against P. iranensis strain SWRI54. This value is below the limits for the demarcation of species, commonly accepted (98.65%). Although the comparison of housekeeping genes is very useful for the identification of bacterial species usually isolated in the clinical field, this marker does not allow discrimination in many isolated environmental strains (Saha et al., 2019). Fortunately, the use of bioinformatics tools for the analysis of the whole genome sequence and its content has been revealed as a useful tool for discrimination and taxonomic ordering (Gutierrez-Albanchez et al., 2021).
The analysis of the total genome size of SAICEUPSMT was comparable with that of its closest relatives, all of them belonging to the Pseudomonas genus. Overall genome sequence comparisons revealed an ANI value between P. atacamensis M7D1 and SAICEUPSMT strain of 95.46% and a dDDH hybridization value of 61.7%. Both values are significantly below the recommended species demarcation values (8.7 and 70%, respectively) (Chun et al., 2018). This suggests that SAICEUPSMT does not belong to any described species.
The most common mechanism of resistance to mercury in Gram negative bacteria consists of the expression and orderly participation of merA, merB, merT, and merC genes included in the mer operon. merA, has the ability to reduce Hg(II) to Hg(0), being this last one volatile. When the substrate is an organomercurial compound, such as methyl-Hg, it is merB that catalyzes the protonolysis cleavage of the C-Hg bond, reducing the Me-Hg to methane (CH4), and Hg(II) (Christakis et al., 2021). The presence in SAICEUPSMT genome of all these genes explains their mercury resilience and tolerance against. The fact that this operon does not have a repressor could also explain its high value of MCB which allows to postulate its bioremediatory application.
Antibiotics resistance genes found in SAICEUPSMT are not transmissible and are common in Pseudomonas genus (Heir et al., 2021). On the other hand, genes involved in virulence mechanisms seem to lack functional capacity.
For all the above, we confirm that, inoculation with SAICEUPSMT strain produces under mercury stress, a significant reduction in the enzymatic response to oxidative stress, significantly promotes plant fitness (increasing total weight, root weight, and number of leaves) and prevents the plant from absorbing mercury from its environment, significantly decreasing its translocation into the shoot. Therefore, SAICEUPSMT strain is postulated as a new taxon not described to date, called P. mercuritolerans, with potential use in improving plant yield under abiotic stress conditions and in heavy metals bioremediation processes, such as mercury.
Description of Pseudomonas mercuritolerans sp. nov.
Pseudomonas mercuritolerans (adj.) refers to the strain’s tolerance to mercury. It was isolated in 2014 from an autochthonous Medicago sativa rhizosphere grown in soils highly contaminated by mercury in the mining district of Almadén (Ciudad Real, Spain). Cells are Gram negative, aerobic, rod-shaped, ranging from 624.1−778.7μm width to 1,679−2,489μm length, non-endospore forming and non-motile. The colonies grow in nutritive agar at an OGT of 28°C forming translucent, round, white-beige colonies. Growth occurs from 4 to 37°C in 24 h. It grows in a pH range of 5.5–8.0 and with a NaCl concentration of 0 to 6%. The optimal temperature, pH, and growth salinity for SAICEUPSMT are 28°C, pH 7 and 0% salinity. It can’t ferment carbohydrates, but it can use urea as a nitrogen source and citrate as the only carbon source; it is catalase and oxidase positive. It produces siderophores (pyoverdine) and can hydrolyze gelatin. The main fatty acids (>81.31% of total fatty acids) were C16:0 (35.59%), sum in feature 3 (18.21%), C17:0 cyclo (16.73%), and sum in Feature 8 (10.78%). The GC genomic DNA content of the type strain is 61.10 mole%. The whole genome sequence has been deposited in the NCBI Bioproject (PRJNA access number 847155), BioSample (SAMN 28920853).
Materials and methods
Legume plant for phytoremediation
Lupinus albus var., Orden Dorado plants were used for the in vivo phytoremediation experiment. Seeds were provided by the seed bank of the Center for Scientific and Technological Research of Extremadura (Spain).
Bacteria for phytoremediation
SAICEUPSMT was selected from a larger set of isolates for its special promotion of plant growth under mercury stress conditions.
Plant growth promotion activities
To determine the in vitro production capacity of auxins (3-indoleacetic acid, IAA), a colorimetric technique was used with Van Urk Salkowski’s reagent (Ehmann, 1977). The bacterium was grown in LB medium (Texas, USA) at 28°C for 4 days, in shaking conditions. The liquid medium was then centrifuged. In total, 1 ml of the supernatant was mixed with 2 ml of Van Urk Salkowski reagent (2% FeCl3 in 35% HClO4 solution) and kept in darkness. Optical density (OD) was measured at 530 nm after 30 min and 120 min. Results were quantified in ppm (μg.ml–1). Glick protocol (Glick, 1995) was followed to differentiate the degradation of ACC by the action of the enzyme ACCd of bacteria that could fix nitrogen. The culture medium contained 1.8% Bacto-Agar (Difco Laboratories, Detroit, MI, USA), low in nitrogen content, supplemented with ACC (30 mmol). The plaques were then inoculated and cultured for 3 days at 28°C, monitoring growth daily. The results were qualitatively evaluated (presence/absence of ACCd enzyme). The production of siderophores was quantified using chrome Azurol S (CAS) agar, described by Alexander and Zuberer (1991) the interpretation was based on the quantitative analysis of the production of siderophores, manifested by the appearance of a halo around the bacteria colonies after 72 h of incubation at 28°C. The ability to solubilize phosphates was tested following the protocol described by De Freitas et al. (1997). Tricalcium phosphate agar (TPM) medium was used (Nautiyal, 1999), with a final pH set to 7 with 1 mole.l–1 with HCl. After inoculation, the plaques were incubated at 28°C for 72 h. The inorganic phosphates solubilizer colonies showed clarification halos that were evaluated qualitatively (presence/absence). All PGPB activities were analyzed in triplicate.
Determination of mercury and other heavy metals minimum bactericidal concentration
Mercury, cadmium, copper, chrome, and nickel MBC was evaluated using Müller Hinton agar (Pronadisa®, Madrid, Spain), supplemented with different concentrations of heavy metal salts [HgCl2, CdSO4, CuSO4, Cr2(SO4)3, NiSO4]: 800, 400, 350, 200, 175, 150, 100, 87.5, 75, 50, 43.75, and 25 μg.ml–1. MBC was determined to be the lowest concentration of heavy metals salts capable of visually inhibiting >99.9% of bacterial growth after 24 h incubation. All assays were carried out in triplicate.
Substrates and setup of the experiment trays
Two types of substrates were used for the tests. The first of them was a soil with a high concentration of mercury, coming from a mining product dump (called Cerro de los Buitrones) in the Almadén mining district (38° 77′ 35′′ N; 4° 85′ 07′′ O, Ciudad Real, Spain). The total mercury concentration in this first substrate is 1,710 ppm (Millán et al., 2007).
The second substrate, used as a control, was a soil with a minimum natural concentration of mercury, from the area furthest, called Fuente del Jardinillo (38° 76′ 01′′ N; 4° 76′ 79′′ O, Ciudad Real, Spain). The use of this substrate instead of one devoid of mercury was done to guarantee the physical-chemical homogeneity of the sample. In both cases, rhizosphere-free soil samples were taken (≈50 kg per soil) from the surface down to 30–35 cm. They were transported under refrigerated conditions (4°C) to the laboratory, where they were stored (4°C) until the assay (<24 h after sampling). Both soils were sieved to guarantee the elimination of the most voluminous fractions and to homogenize the granulometry in each test and replicas.
Four sterile forest trays were used (Plásticos Solanas S.L., Zaragoza, Spain), each composed of twelve alveoli, with a capacity of 300 cm3, with a light of 5.3 × 5.3 cm. Four pre-germinated Lupinus albus seeds (2.0 ± 0.5 cm emerged radicle) were sown in each alveolus.
Inoculant preparation
SAICEUPSMT strain was incubated in nutritive agar (Pronadisa®, Madrid, Spain) supplemented with 50 ppm of HgCl2 (24 h, 25°C). A bacterial suspension was prepared in 0.45% saline solution and adjusted to 0.5 McFarland (OD 108 cfu.ml–1). It was intended to avoid an increase in salinity, which could be aggravated by the incorporation of mercury salts, and which could compromise the correct development of seedlings. Each seed was inoculated with 1 ml of suspension.
Growth under greenhouse conditions
Plants were grown for 6 weeks in a phytotron equipped with white and yellow light (photoperiod of 11 h of light, light intensity 505 μmol.m–2.s–1, stable room temperature 25 ± 3°C). Irrigation was performed every 48 h by capillarity with sterile tap water, with an experimental volume of 350 ml/tray.
Evaluation of the effects of mercury on plant development
Plant biometry
For biometric parameter determination, after 6-week experiment, whole plants were harvested. Roots and shoots were washed with distilled water. With the recently harvested plants, the following parameters were measured: total weight (g), shoot weight (g), root weight (g), shoot length (cm), root length (cm), total number of leaves (No), and total number of secondary roots (No).
Plant antioxidant response
Enzymes were extracted at 4°C from 1 g of fresh sample, with a mortar and using 50 mg of polyvinylpolypyrrolidone (PVPP) and 10 ml of the following medium: 50 mm of K-phosphate buffer (pH 7.8) with 0.1 mm EDTA (for SOD, CAT, and APX). The same medium, supplemented with 10 mm of β-mercaptoethanol was used for GR. Superoxide dismutase (SOD) enzyme was measured based on SOD’S ability to inhibit the reduction of tetrazoyl nitro-blue (NBT) by photochemically generated superoxide radicals. A unit of turf is defined as the amount of enzyme needed to inhibit the rate of NBT reduction by 50% at 25°C (Burd et al., 2000). Catalase production was quantified using Aebi method (Aebi, 1984). H2O2 consumption was monitored for 1 min at 240 nm. This was carried out by mixing 50 mm of potassium phosphate buffer with 10 mm of H2O2 and 100 μl of the extract. APX content was measured in a 1 ml reaction containing 80 nm of potassium phosphate buffer, 2.5 mm of H2O2 and 1M sodium ascorbate. H2O2 was added to begin the reaction and absorbance reduction was measured for 1 min at 290 nm, to determine the oxidation ratio of ascorbate (Amako et al., 1994). Glutathione reductase (GR) enzyme was estimated spectrophotometrically, according to the Carlberg and Mannervik (1985) method at 25°C and 340 nm. To do this, the reaction mixture contained 50 mm of Tris-MgCl2 buffer, 3 mm, 1 mm of GSSG, 50 μl of enzyme, and 0.3 mm NADPH, added to initiate the reaction. Enzyme concentration was calculated with the initial rate of the reaction and the molar extinction coefficient of NADPH (ε340 = 6.22 mm–1 cm–1).
Mercury accumulation in plant
The root and shoot fraction of each replica were dried in dry heat furnaces at 60°C for 24 h. Each fraction was crushed and digested separately in acid medium (HNO3/HCl 2%/0.5% weight/volume) under pressure for the determination of trace elements according to the UNE-EN 13805 standard. The product of the digestion was then analyzed by inductively coupled plasma mass spectrometry (ICP-MS). Using a calibration curve, a correlation was established between the concentration of the standard (μg l–1) and the signal (ICP-MS) of mercury element. The element signal value in the 12 samples was interpolated on the calibration curve, resulting in the total concentration of the mercury in the sample. The mercury standard values for establishing the calibration line were as follows, expressed in μg.l–1: 0.00; 0.05; 0.10; 0.50; 1.00; 5.00; 10.00. Final units’ mg kg–1.
Cf (mg kg–1) is the metal content of the sample, X (μg l–1) corresponds to the interpolated experimental value or the extrapolated experimental value of the standard addition; D is the dilution factor; V (mL) is the volume of the flask, and W(g) to the weight of the sample.
SAICEUPSMT strain identification
Transmission electron microscopy (TEM)
To determine the size and shape of the analyzed strain, the Prism E scanning electron microscope (SEM) (Thermo Fisher Scientific Inc., Waltham, MA, USA) was used. The culture was observed in suspension. A drop of Formvar was used in transmission electron microscopy (TEM) Cu grids of mesh 200 as a sample support in the grid for TEM microscopy. The measurement conditions were working distance of 10 mm; 24 pA electron current; electron acceleration of 30 kV and size of 2 points, pressure of 375 Pa, at 4°C and a humidity of 50%. The electron microscopy images were obtained by the research support service (SAI) of “X-ray diffraction and scanning electron microscopy” (SAI-DRX-MEB) of the San Pablo CEU University (Madrid, Spain).
Biochemical tests
Oxi/Ferm Pluri Test® (Liofilchem, Italy) was used. Next, the automatic characterization was carried out with the VITEK® 2 equipment and with the VITEK®2 GN identification cards (bioMérieux, Marcy-l’Étoile, France). The motility of the bacterium was tested in Motility Test Agar, (Liofilchem, Italy). Antimicrobial sensitivity was determined using E-test in Müller Hinton agar (Pronadisa®, Madrid, Spain) using the following antibiotics: piperacillin and piperacillin with tazobactam, cefepime (bioMérieux, Marcy-l’Étoile, France); ceftazidime, imipenem, imipenem with EDTA, amikacin, gentamicin, and ciprofloxacin (Liofilchem, Italy).
Fatty acids
The analysis of cellular fatty acids was carried out in the Spanish Collection of Type Crops (CECT) at the University of Valencia, Spain. Cells were grown in M2 medium for 48 h, 30°C; extractions and determinations were carried out according to the standard protocol of the MIDI Microbial Identification System (Sasser, 1990) using a chromatograph Agilent 6850 (Agilent Technologies) following the method TSBA6 (MIDI, 2008, version 6.1. Newark, DE: MIDI Inc.).
Phylogenetic analysis
Matrix-assisted laser desorption/ionization–time of flight (MALDI-TOF) was used. It was carried out in the Vitek MS IND system (BioMérieux, Marcy-l’Étoile, France) at the Carlos III Health Institute (Majadahonda, Madrid). Slides were inoculated with a sterile handle. A total of 1 μl of the matrix solution (VITEK MS-CHCA: mixture of 3.10 g of 2.5-dihydroxy 36 benzoic acid dissolved in 100 ml of water-ethanol-acetonitrile in 1/1/1 ratio) was added to each well and allowed to dry at room temperature. the temperature. Mass spectra were generated with the Axima Assurance system (Shimadzu Corporation, Kyoto, Japan), using the Shimadzu Launchpad software program and the SARAMIS MS-ID v1 database application (AnagnosTee GmbH) for automatic measurement and identification. All strains were analyzed in duplicate. No pre-treatment was used before inoculation on the slide. High confidence identification was considered when the assessment was equal to or greater than 97%.
SAICEUPSMT genomic DNA was extracted from fresh cells. Amplification of the 16S rRNA gene was done and BLAST algorithm was used to search for similar sequences. An in silico genome analysis was carried out among the most closely related species, for this purpose the Type (Strain) Genome Server (TYGS) service was used using blast+ software. The resulting intergenic distances were used to infer a balanced evolution with branch support through FASTME 2.1.6.1. Compatibility was inferred from 100 pseudo-bootstrap replicas. Trees were rooted in the midpoint and visualized with PhyD3. For the calculation of ANI and AAI the tools available in JSpeciesWS and the ANI calculator1 were used.
Genome sequencing and bioinformatics analysis
For the extraction of genomic DNA, the QIAamp DNA Kit (QIAGEN®, Hilden, Germany) was used. SAICEUPSMT genome was obtained by whole genome sequencing using an Illumina Miseq platform. A total of 200 ng of genomic DNA were used, measured by fluorimetry (Quant-iT Picogreen, Thermo Fisher). To make libraries, the NEB Next ultra II FS DNA preparation kit was used, according to the manufacturer’s protocol (New England Biolabs). The initial fragmentation time was 7.5 min, and the final PCR amplification was done with six cycles. The resulting DNA fragments were evaluated and quantified by bioanalyzer using a 7500 DNA (Agilent) chip. The libraries were quantified by qPCR using the master mix “Kapa-SYBR FAST qPCR kit for LightCycler480.” Libraries were sequenced in Illumina’s Miseq equipment following manufacturer’s instructions, in a pair end 2 × 300 type race using “Miseq reagent kit v3 600 cycles.”
The quality of the sequenced reads was processed using FastQC v.0.11.3 (Babraham Bioinformatics) (Andrews, 2010). They were filtered for low quality reads using Prinseq (Schmieder and Edwards, 2011) and adapter regions using CUTADAPT (Martin, 2011). To eliminate duplicate reads or those that were only present in forward or reverse, FASTQCollapser and FASTQIntersect were used. The de novo assembly of the genome was performed with SPAdes v.3.13 (Center for Algorithmic Biotechnology) (Bankevich et al., 2012), the metrics of the assemblies were obtained through SeqEditor (Hafez et al., 2021) and annotated using the Prokka software, version 1.132 (Seemann, 2014) and Rapid using Subsystem Technology (RAST) version 2.03 with predetermined parameters (Aziz et al., 2012). The genomes of the related strains were obtained from the Genbank database.
Additional bioinformatic analyses were performed. tRNAscan-SE v.2.04 was used to predict tRNAs. Several clinically important antimicrobial resistance genes and virulence determinants were searched through functional annotation data generated from Rast, Prokka, and ResFinder 4.1 annotation lines. Several mercury resistance and plant growth promotion genes were searched through functional annotation data generated from Rast and Prokka annotation lines. KEGG software was used to examine the metabolic pathways of the strain, and PathogenFinder v.1.1., Rast and Prokka software were used to estimate pathogenicity.
Statistical analysis
The Kolmogorov–Smirnov test was performed to check the normality of all variables. After that, for the analysis of the biometric data, an ANOVA test was carried out to analyze the plant response in the presence of SAICEUPSMT in substrates under high and low mercury concentration. For those parameters that showed statistical significance (p-value ≤ 0.05), the multiple comparison test least significant difference (LSD) was performed, to identify if SAICEUPSMT produced any significant variation in plant biometry, compared to controls. For the analysis of the phytoprotective capacity, a Kruskal–Wallis analysis was performed. For the evaluation of mercury accumulation in plants, the normality of the sample data was verified using the Shapiro–Wilk test. An ANOVA analysis was then performed to determine the existence of significant differences (p-value ≤ 0.05) between treatments. All statistical differences refer to the comparison of the variables when the plant has been inoculated, compared to their respective non-inoculated controls. SPSS v.27.0 software was used (Version 27.0 IBM Corp., Armonk, NY, USA).
Data availability statement
The data presented in this study are deposited in the Genbank repository, accession number: JAMSHA000000000.
Author contributions
PJ, VF, and MR: conceptualization and methodology. VF: software. PJ and MR: validation and supervision. DG, VF, and LG: formal analysis. DG, VF, LG, and MR: investigation. VF and LG: resources. PJ, VF, and LG: data curation. LG and MR: writing—original draft preparation and visualization. PJ, AP, and MR: writing—review and editing. AP and PJ: project administration and funding acquisition. All authors read and agreed to the published version of the manuscript.
Funding
This research was funded by Fundación Universitaria San Pablo CEU (Microbiology SAI) and Banco Santander, grant number: FUSP-BS-PPC01/2014.
Acknowledgments
We are grateful to the Seed Bank of the Center for Scientific and Technological Research of Extremadura, for its permanent availability in the supply of seeds for trials. Special thanks to the Carlos III Health Institute, Laboratory of Bacterial Taxonomy (Majadahonda, Madrid, Spain), for helping us in sequencing the complete genome of the strain. Especially very thank to Sylvia Valdezate Ramos, María José Medina Pascual, and Gema Carrasco Diaz.
Conflict of interest
The authors declare that the research was conducted in the absence of any commercial or financial relationships that could be construed as a potential conflict of interest.
Publisher’s note
All claims expressed in this article are solely those of the authors and do not necessarily represent those of their affiliated organizations, or those of the publisher, the editors and the reviewers. Any product that may be evaluated in this article, or claim that may be made by its manufacturer, is not guaranteed or endorsed by the publisher.
Supplementary material
The Supplementary Material for this article can be found online at: https://www.frontiersin.org/articles/10.3389/fmicb.2022.1032901/full#supplementary-material
Footnotes
- ^ http://enve-omics.ce.gatech.edu/ani/, accessed 5 July 2022.
- ^ https://github.com/tseemann/prokka
- ^ https://rast.nmpdr.org/
- ^ http://lowelab.ucsc.edu/tRNAscan-SE/
References
Aebi, H. (1984). “[13] Catalase in vitro,” in Methods in Enzymology, eds J. Abelson and M. Simon (Amsterdam: Elsevier), 121–126.
Alexander, D. B., and Zuberer, D. A. (1991). Use of chrome azurol S reagents to evaluate siderophore production by rhizosphere bacteria. Biol. Fertil. Soils 12, 39–45. doi: 10.1007/BF00369386
Amako, K., Chen, G.-X., and Asada, K. (1994). Separate assays specific for ascorbate peroxidase and guaiacol peroxidase and for the chloroplastic and cytosolic isozymes of ascorbate peroxidase in plants. Plant Cell Physiol. 35, 497–504.
Andrews, S. (2010). FastQC: A quality control tool for high throughput sequence data. Available online at: http://www.bioinformatics.babraham.ac.uk/projects/fastqc
Azaroual, S. E., Kasmi, Y., Aasfar, A., El Arroussi, H., Zeroual, Y., El Kadiri, Y., et al. (2022). Investigation of bacterial diversity using 16S rRNA sequencing and prediction of its functionalities in Moroccan phosphate mine ecosystem. Sci. Rep. 12, 1–16. doi: 10.1038/s41598-022-07765-5
Aziz, R. K., Devoid, S., Disz, T., Edwards, R. A., Henry, C. S., Olsen, G. J., et al. (2012). SEED servers: high-performance access to the SEED genomes, annotations, and metabolic models. PLoS One 7:e48053. doi: 10.1371/journal.pone.0048053
Ballabio, C., Jiskra, M., Osterwalder, S., Borrelli, P., Montanarella, L., and Panagos, P. (2021). A spatial assessment of mercury content in the European Union topsoil. Sci. Total Environ. 769:144755. doi: 10.1016/j.scitotenv.2020.144755
Bankevich, A., Nurk, S., Antipov, D., Gurevich, A. A., Dvorkin, M., Kulikov, A. S., et al. (2012). SPAdes: A new genome assembly algorithm and its applications to single-cell sequencing. J. Comput. Biol. 19, 455–477.
Beltrán-Pineda, M. E., and Gómez-Rodríguez, A. M. (2016). Biorremediación de metales pesados cadmio (Cd), cromo (Cr) y mercurio (Hg), mecanismos bioquímicos e ingeniería genética: una revisión. Rev. Fac. Cienc. Básicas 12, 172–197. doi: 10.18359/rfcb.2027
Bhatt, P., Verma, A., Gangola, S., Bhandari, G., and Chen, S. (2021). Microbial glycoconjugates in organic pollutant bioremediation: recent advances and applications. Microb. Cell Fact. 20, 1–18. doi: 10.1186/s12934-021-01556-9
Bravakos, P., Mandalakis, M., Nomikou, P., Anastasiou, T. I., Kristoffersen, J. B., Stavroulaki, M., et al. (2021). Genomic adaptation of Pseudomonas strains to acidity and antibiotics in hydrothermal vents at Kolumbo submarine volcano, Greece. Sci. Rep. 11, 1–12. doi: 10.1038/s41598-020-79359-y
Burd, G. I., Dixon, D. G., and Glick, B. R. (2000). Plant growth-promoting bacteria that decrease heavy metal toxicity in plants. Can. J. Microbiol. 46, 237–245.
Carlberg, I., and Mannervik, B. (1985). “[59] Glutathione reductase,” in Methods in Enzymology, eds J. Abelson and M. Simon (Amsterdam: Elsevier), 484–490.
Çavus̨oğlu, D., Macar, O., Kalefetoğlu Macar, T., Çavus̨oğlu, K., and Yalçın, E. (2022). Mitigative effect of green tea extract against mercury(II) chloride toxicity in Allium cepa L. model. Environ. Sci. Pollut. Res. 29, 27862–27874. doi: 10.1007/s11356-021-17781-z
Chellaiah, E. R. (2018). Cadmium (heavy metals) bioremediation by Pseudomonas aeruginosa: a minireview. Appl. Water Sci. 8:154. doi: 10.1007/s13201-018-0796-5
Christakis, C. A., Barkay, T., and Boyd, E. S. (2021). Expanded diversity and phylogeny of mer genes broadens mercury resistance paradigms and reveals an origin for MerA among thermophilic Archaea. Front. Microbiol. 12:682605. doi: 10.3389/fmicb.2021.682605
Chun, J., Oren, A., Ventosa, A., Christensen, H., Arahal, D. R., da Costa, M. S., et al. (2018). Proposed minimal standards for the use of genome data for the taxonomy of prokaryotes. Int. J. Syst. Evol. Microbiol. 68, 461–466. doi: 10.1099/ijsem.0.002516
Cody, R. B., McAlpin, C. R., Cox, C. R., Jensen, K. R., and Voorhees, K. J. (2015). Identification of bacteria by fatty acid profiling with direct analysis in real time mass spectrometry. Rapid Commun. Mass Spectr. 29, 2007–2012. doi: 10.1002/rcm.7309
Consentino, B. B., Aprile, S., Rouphael, Y., Ntatsi, G., De Pasquale, C., Iapichino, G., et al. (2022). Application of PGPB combined with variable n doses affects growth, yield-related traits, n-fertilizer efficiency and nutritional status of lettuce grown under controlled condition. Agronomy 12:236.
Dary, M., Chamber-Pérez, M., Palomares, A., and Pajuelo, E. (2010). “In situ” phytostabilisation of heavy metal polluted soils using Lupinus luteus inoculated with metal resistant plant-growth promoting rhizobacteria. J. Hazardous Mater. 177, 323–330. doi: 10.1016/j.jhazmat.2009.12.035
De Freitas, J., Banerjee, M., and Germida, J. (1997). Phosphate-solubilizing rhizobacteria enhance the growth and yield but not phosphorus uptake of canola (Brassica napus L.). Biol. Fertil. Soils 24, 358–364.
Ehmann, A. (1977). The Van Urk-Salkowski reagent—a sensitive and specific chromogenic reagent for silica gel thin-layer chromatographic detection and identification of indole derivatives. J. Chromatogr. A 132, 267–276. doi: 10.1016/s0021-9673(00)89300-0
Fumagalli, P., Comolli, R., Ferrè, C., Ghiani, A., Gentili, R., and Citterio, S. (2014). The rotation of white lupin (Lupinus albus L.) with metal-accumulating plant crops: a strategy to increase the benefits of soil phytoremediation. J. Environ. Manag. 145, 35–42. doi: 10.1016/j.jenvman.2014.06.001
Gill, S. S., and Tuteja, N. (2010). Reactive oxygen species and antioxidant machinery in abiotic stress tolerance in crop plants. Plant Physiol. Biochem. 48, 909–930.
Glick, B. R. (1995). The enhancement of plant growth by free-living bacteria. Can. J. Microbiol. 41, 109–117.
Godínez-Méndez, L. A., Gurrola-Díaz, C. M., Zepeda-Nuño, J. S., Vega-Magaña, N., Lopez-Roa, R. I., Íñiguez-Gutiérrez, L., et al. (2021). In vivo healthy benefits of galacto-oligosaccharides from Lupinus albus (LA-GOS) in butyrate production through intestinal microbiota. Biomolecules 11:1658. doi: 10.3390/biom11111658
Gutierrez-Albanchez, E., García-Villaraco, A., Lucas, J. A., Horche, I., Ramos-Solano, B., and Gutierrez-Mañero, F. J. (2021). Pseudomonas palmensis sp. nov., a novel bacterium isolated from nicotiana glauca microbiome: draft genome analysis and biological potential for agriculture. Front. Microbiol. 12:672751. doi: 10.3389/fmicb.2021.672751
Hafez, A., Futami, R., Arastehfar, A., Daneshnia, F., Miguel, A., Roig, F. J., et al. (2021). SeqEditor: An application for primer design and sequence analysis with or without GTF/GFF files. Bioinformatics 37, 1610–1612.
Heidari, M., and Golpayegani, A. (2012). Effects of water stress and inoculation with plant growth promoting rhizobacteria (PGPR) on antioxidant status and photosynthetic pigments in basil (Ocimum basilicum L.). J. Saudi Soc. Agric. Sci. 11, 57–61. doi: 10.1016/j.jssas.2011.09.001
Heir, E., Moen, B., Åsli, A. W., Sunde, M., and Langsrud, S. (2021). Antibiotic resistance and phylogeny of Pseudomonas spp. isolated over three decades from chicken meat in the Norwegian food chain. Microorganisms 9:207. doi: 10.3390/microorganisms9020207
Kang, S.-M., Asaf, S., Khan, A. L., Khan, A., Mun, B.-G., Khan, M. A., et al. (2020). Complete genome sequence of Pseudomonas psychrotolerans CS51, a plant growth-promoting bacterium, under heavy metal stress conditions. Microorganisms 8:382. doi: 10.3390/microorganisms8030382
Kuiper, I., Lagendijk, E. L., Bloemberg, G. V., and Lugtenberg, B. J. (2004). Rhizoremediation: a beneficial plant-microbe interaction. Mol. Plant Microbe Interact. 17, 6–15.
Martin, M. (2011). Cutadapt removes adapter sequences from high-throughput sequencing reads. EMBnet J. 17, 10–12. doi: 10.1089/cmb.2017.0096
Matyar, F., Akkan, T., Uçak, Y., and Eraslan, B. (2010). Aeromonas and Pseudomonas: antibiotic and heavy metal resistance species from Iskenderun Bay, Turkey (northeast Mediterranean Sea). Environ. Monit. Assess. 167, 309–320. doi: 10.1007/s10661-009-1051-1
Mellidou, I., and Karamanoli, K. (2022). Unlocking PGPR-mediated abiotic stress tolerance: what lies beneath. Front. Sustain. Food Syst. 6:832896. doi: 10.3389/fsufs.2022.832896
Millán, R., Carpena, R., Schmid, T., Sierra, M., Moreno, E., Peñalosa, J., et al. (2007). Rehabilitación de suelos contaminados con mercurio: estrategias aplicables en el área de Almadén. Rev. Ecosistemas 16, 56–66.
Morcillo, R. J. L., and Manzanera, M. (2021). The effects of plant-associated bacterial exopolysaccharides on plant abiotic stress tolerance. Metabolites 11:337. doi: 10.3390/metabo11060337
Nautiyal, C. S. (1999). An efficient microbiological growth medium for screening phosphate solubilizing microorganisms. FEMS Microbiol. Lett. 170, 265–270.
Pirzadah, T. B., Malik, B., Tahir, I., Irfan, Q. M., and Rehman, R. U. (2018). Characterization of mercury-induced stress biomarkers in Fagopyrum tataricum plants. Int. J. Phytoremed. 20, 225–236. doi: 10.1080/15226514.2017.1374332
Quiñones, M. A., Fajardo, S., Fernández-Pascual, M., Lucas, M. M., and Pueyo, J. J. (2021). Nodulated white lupin plants growing in contaminated soils accumulate unusually high mercury concentrations in their nodules, roots and especially cluster roots. Horticulturae 7:302.
Richter, M., and Rosselló-Móra, R. (2009). Shifting the genomic gold standard for the prokaryotic species definition. Proc. Natl. Acad. Sci. U.S.A. 106, 19126–19131. doi: 10.1073/pnas.0906412106
Robas, M., Jiménez, P. A., González, D., and Probanza, A. (2021). Bio-mercury remediation suitability index: a novel proposal that compiles the PGPR features of bacterial strains and its potential use in phytoremediation. Int. J. Environ. Res. Public Health 18:4213. doi: 10.3390/ijerph18084213
Sah, S., Krishnani, S., and Singh, R. (2021). Pseudomonas mediated nutritional and growth promotional activities for sustainable food security. Curr. Res. Microb. Sci. 2:100084. doi: 10.1016/j.crmicr.2021.100084
Saha, J., Saha, B. K., Pal Sarkar, M., Roy, V., Mandal, P., and Pal, A. (2019). Comparative genomic analysis of soil dwelling bacteria utilizing a combinational codon usage and molecular phylogenetic approach accentuating on key housekeeping genes. Front. Microbiol. 10:2896. doi: 10.3389/fmicb.2019.02896
Sahu, S. K., Behuria, H. G., Gupta, S., Dalua, R. B., Sahoo, S., Parida, D., et al. (2016). Biochemical characterization of high mercury tolerance in a Pseudomonas spp. isolated from industrial effluent. Am. J. Curr. Microbiol. 4, 41–54.
Sánchez-Fernández, R., Fricker, M., Corben, L. B., White, N. S., Sheard, N., Leaver, C. J., et al. (1997). Cell proliferation and hair tip growth in the Arabidopsis root are under mechanistically different forms of redox control. Proc. Natl. Acad. Sci. U.S.A. 94, 2745–2750. doi: 10.1073/pnas.94.6.2745
Sandhya, V., Ali, S. K. Z., Grover, M., Reddy, G., and Venkateswarlu, B. (2010). Effect of plant growth promoting Pseudomonas spp. on compatible solutes, antioxidant status and plant growth of maize under drought stress. Plant Growth Regul. 62, 21–30. doi: 10.1007/s10725-010-9479-4
Sasser, M. (1990). Identification of Bacteria by Gas Chromatography of Cellular Fatty Acids. Newark: MIDI.
Schmieder, R., and Edwards, R. (2011). Quality control and preprocessing of metagenomic datasets. Bioinformatics 27, 863–864.
Singh, M., Singh, D., Gupta, A., Pandey, K. D., Singh, P. K., and Kumar, A. (2019). “Chapter Three - plant growth promoting rhizobacteria: application in biofertilizers and biocontrol of phytopathogens,” in PGPR Amelioration in Sustainable Agriculture, eds A. K. Singh, A. Kumar, and P. K. Singh (Sawston: Woodhead Publishing), 41–66.
Singh, S., and Hiranmai, R. (2021). Monitoring and molecular characterization of bacterial species in heavy metals contaminated roadside soil of selected region along NH 8A, Gujarat. Heliyon 7:e08284. doi: 10.1016/j.heliyon.2021.e08284
Singh, V., Chauhan, P. K., Kanta, R., Dhewa, T., and Kumar, V. (2010). Isolation and characterization of Pseudomonas resistant to heavy metals contaminants. Int. J. Pharm. Sci. Res. 30, 164–167.
Zelles, L. (1999). Fatty acid patterns of phospholipids and lipopolysaccharides in the characterisation of microbial communities in soil: a review. Biol. Fertil. Soils 29, 111–129.
Zhang, M., Li, A., Yao, Q., Xiao, B., and Zhu, H. (2022). Pseudomonas oligotrophica sp. nov., a novel denitrifying bacterium possessing nitrogen removal capability under low carbon–nitrogen ratio condition. Front. Microbiol. 13:882890. doi: 10.3389/fmicb.2022.882890
Zhang, W., Chen, L., and Liu, D. (2012). Characterization of a marine-isolated mercury-resistant Pseudomonas putida strain SP1 and its potential application in marine mercury reduction. Appl. Microbiol. Biotechnol. 93, 1305–1314. doi: 10.1007/s00253-011-3454-5
Zhang, Y., Song, Z., Huang, S., Zhang, P., Peng, Y., Wu, P., et al. (2021). Global health effects of future atmospheric mercury emissions. Nat. Commun. 12, 1–10.
Keywords: heavy metals, PGPB, oxidative stress protection, Pseudomonas mercuritolerans, phytoprotection against mercury, mercury contamination
Citation: Robas Mora M, Fernández Pastrana VM, González Reguero D, Gutiérrez Oliva LL, Probanza Lobo A and Jiménez Gómez PA (2022) Oxidative stress protection and growth promotion activity of Pseudomonas mercuritolerans sp. nov., in forage plants under mercury abiotic stress conditions. Front. Microbiol. 13:1032901. doi: 10.3389/fmicb.2022.1032901
Received: 31 August 2022; Accepted: 14 November 2022;
Published: 06 December 2022.
Edited by:
Paola Grenni, National Research Council, ItalyReviewed by:
Rakesh Kumar Sharma, Manipal University Jaipur, IndiaSavitha Santosh, Central Research Institute for Dryland Agriculture (ICAR), India
Copyright © 2022 Robas Mora, Fernández Pastrana, González Reguero, Gutiérrez Oliva, Probanza Lobo and Jiménez Gómez. This is an open-access article distributed under the terms of the Creative Commons Attribution License (CC BY). The use, distribution or reproduction in other forums is permitted, provided the original author(s) and the copyright owner(s) are credited and that the original publication in this journal is cited, in accordance with accepted academic practice. No use, distribution or reproduction is permitted which does not comply with these terms.
*Correspondence: Marina Robas Mora, marina.robasmora@ceu.es; Pedro A. Jiménez Gómez, pedro.jimenezgomez@ceu.es