Therapeutic Low-Intensity Ultrasound for Peripheral Nerve Regeneration – A Schwann Cell Perspective
- Department of Neuroscience and Experimental Therapeutics, Albany Medical College, Albany, NY, United States
Peripheral nerve injuries are common conditions that can arise from trauma (e.g., compression, severance) and can lead to neuropathic pain as well as motor and sensory deficits. Although much knowledge exists on the mechanisms of injury and nerve regeneration, treatments that ensure functional recovery following peripheral nerve injury are limited. Schwann cells, the supporting glial cells in peripheral nerves, orchestrate the response to nerve injury, by converting to a “repair” phenotype. However, nerve regeneration is often suboptimal in humans as the repair Schwann cells do not sustain their repair phenotype long enough to support the prolonged regeneration times required for successful nerve regrowth. Thus, numerous strategies are currently focused on promoting and extending the Schwann cells repair phenotype. Low-intensity ultrasound (LIU) is a non-destructive therapeutic approach which has been shown to facilitate peripheral nerve regeneration following nerve injury in rodents. Still, clinical trials in humans are scarce and limited to small population sizes. The benefit of LIU on nerve regeneration could possibly be mediated through the repair Schwann cells. In this review, we discuss the known and possible molecular mechanisms activated in response to LIU in repair Schwann cells to draw support and attention to LIU as a compelling regenerative treatment for peripheral nerve injury.
Introduction
Peripheral nerve injuries refer to traumatic compression, cutting, or stretching of the peripheral nerve and cause a serious health problem that affects 2–3% of trauma patients annually (Noble et al., 1998; Taylor et al., 2008; Lad et al., 2010). Peripheral nerve injuries are classified according to their severity; grade I refers to reversible local conduction block, grades II and III refers to interruption of the axon and supporting structures, respectively, while grades IV and V refers to interruption of the nerve fascicle and all the nerve fibers, respectively (Sunderland, 1951). Because peripheral nervous system (PNS) axons can regrow, there is a frequent misbelief that neuronal damage can be repaired in the PNS without therapeutic strategies to support axon regeneration. Yet, in proximal injuries grade II and above, the axonal regrowth may occur but the long distance between the site of injury and the target organ greatly limits reinnervation. In addition, for grade IV/V injuries, when the nerve fascicle and/or fibers are separated, microsurgical repair is required to reconnect the nerve stump, but the regenerating axons fail to reinnervate their tissue targets (for review, see Menorca et al., 2013). Therefore, despite axon intrinsic regenerative potential, peripheral nerve regeneration following traumatic injury is often suboptimal, and generally results in life-long impairments, pain, and significant healthcare costs (Karsy et al., 2019; Bergmeister et al., 2020). Thus, additional therapies are explored to facilitate regeneration of all types of nerve injuries. In this review we will be focusing on the use of non-invasive therapeutic ultrasound in nerve regeneration.
An ultrasound is a sound wave above the human hearing threshold (above 20 kHz), which is commonly known for its clinical use in safe and non-invasive medical imaging. Despite being heavily used for diagnosis in imaging techniques, ultrasound can also generate mechanical energy. As the sound wave is being absorbed into biological tissue, it causes vibrations. The vibrations mediated by ultrasound are currently used in therapeutic settings through two main modalities: high intensity ultrasound and low-intensity ultrasound. High intensity ultrasound (>3 W/cm2) entails tissue molecular vibration, which converts into thermal generation (heat) and is used for the precise destruction of benign or malignant tissues. However, at low intensities (≤1 W/cm2), the thermal effect of sound waves is minimal or absent, thus causing no tissue damage, and is currently used to induce regenerative effects on biological tissues, to modulate nerve activity and to facilitate drug delivery.
Low-intensity ultrasound (LIU) is a non-thermogenic and non-destructive continuous wave with medium frequency ultrasound (1–3 MHz) and is delivered at low intensity. The frequency (i.e., the number of vibration cycles that occur in 1 s) of the LIU allows the sound wave to penetrate from 1 to 2 cm into biological tissues (1 MHz) up to 3–5 cm (3 MHz) (Takebe et al., 2014). LIU can be delivered through a pulse wave (i.e., in general ON for 20 μs and OFF for 80 μs). The pulsatile nature of ultrasound facilitates the emission of sound waves without heat generation (Grogan and Mount, 2021). Most studies thus far have used low intensity pulsed ultrasound (Tables 1, 2).
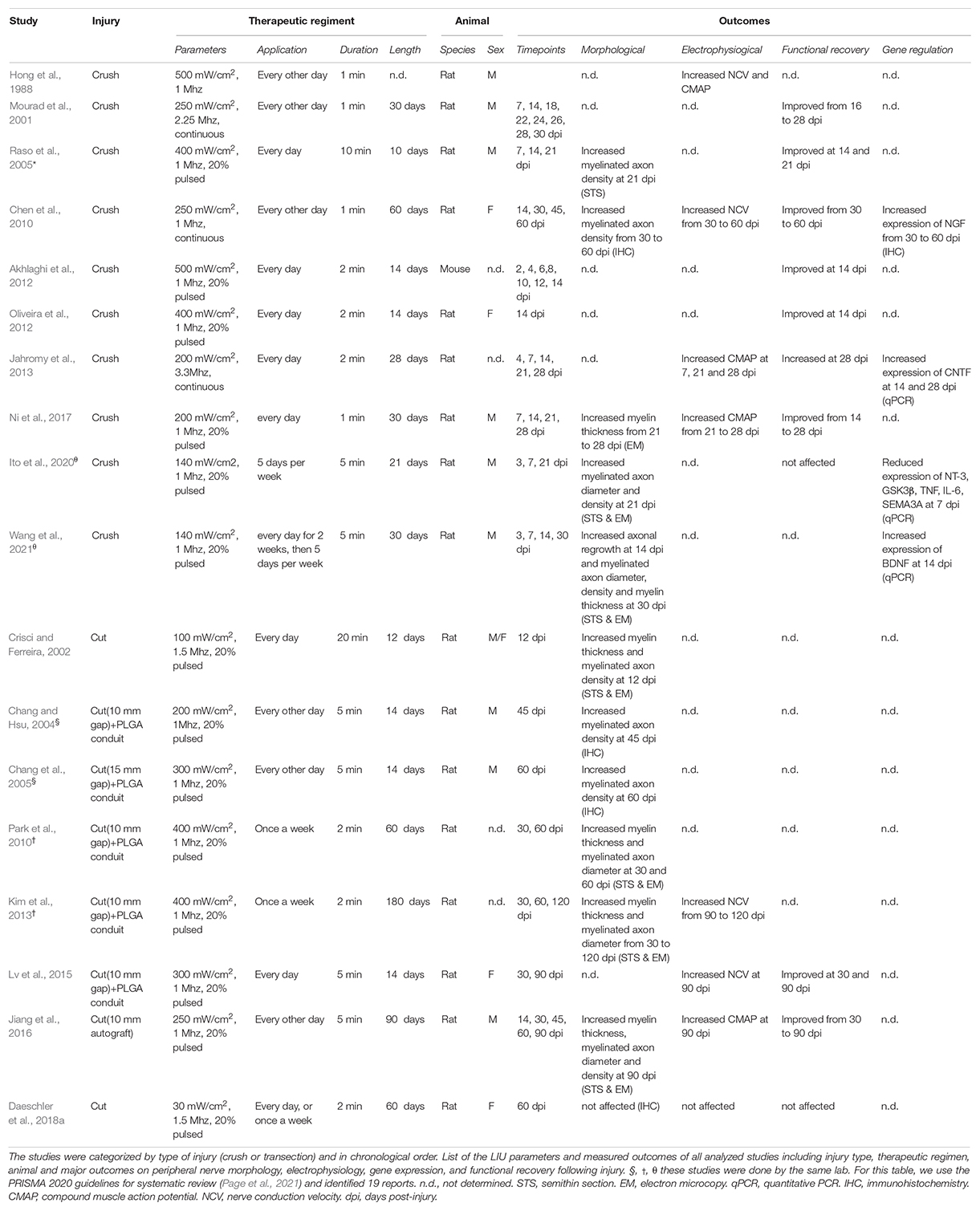
Table 1. Experimental parameters and outcomes of in vivo studies investigating the role of LIU on peripheral nerve after injury.
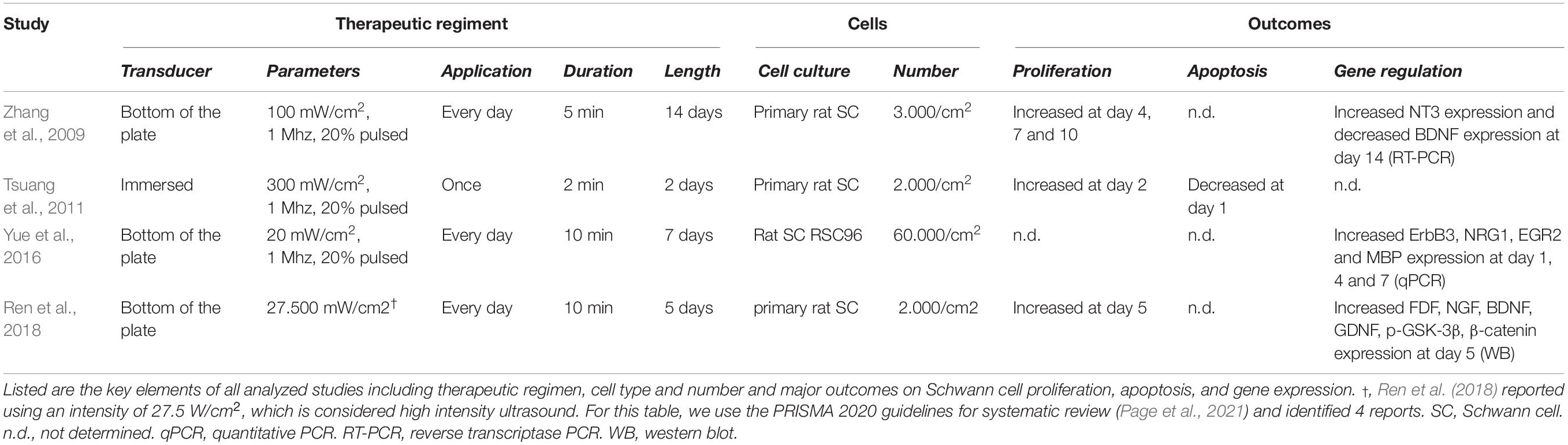
Table 2. Experimental parameters and outcomes of in vitro studies investigating the role of LIU on Schwann cells in vitro.
Low-intensity ultrasound was approved by the US FDA about 27 years ago for fracture repair. Since then, a growing amount of literature demonstrates that LIU non-invasive physical stimulus can stimulate or inhibit physiological processes and facilitate drug delivery. More specifically, LIU can accelerate soft-tissue regeneration (e.g., muscles, tendons, ligaments) (Ikai et al., 2008; Jeremias Junior et al., 2011; Ren et al., 2013), inhibits inflammatory responses (Nakao et al., 2014; Zhao et al., 2017), and modulates neuronal activity (Iwashina et al., 2006; Su et al., 2017; Zou et al., 2021). For a more comprehensive review the therapeutic applications of LIU, see (Xin et al., 2016; Jiang et al., 2019; de Lucas et al., 2020; Uddin et al., 2021). While the application of LIU could also be of great benefit to nerve repair by promoting neuromodulation, neuronal regrowth and neuromuscular rehabilitation, the clinical efficacy of LIU in neuromuscular trauma and neurodegenerative diseases is understudied. Thus, the utilization of LIU in nerve regenerative medicine is still limited. More pre-clinical and clinical studies are necessary to evaluate LIU as a suitable clinical tool in nerve repair. We review here the effect of LIU on peripheral nerve regeneration in rodent pre-clinical studies and more specifically the effect of LIU on Schwann cells, the supporting glial cells of the PNS, known for their capacity to reprogram into repair cells to promote nerve repair.
Effect of Low-Intensity Ultrasound on Peripheral Nerve Regeneration
Given the limitations of peripheral nerves to self-heal in humans, therapeutic approaches to promote nerve regeneration must be developed. Over the last 20 years, numerous groups have investigated the therapeutic effect of LIU on peripheral nerve injury to facilitate regeneration and improve function both in pre-clinical (Table 1) and clinical settings. For a recent meta-analysis of functional outcomes in pre-clinical studies, see (Daeschler et al., 2018b). For a recent meta-analysis of clinical studies, see (Haffey et al., 2020). Our review will point out how the research on the effect of LIU application in nerve repair is considerably scattered between type of injury model used, the LIU parameters applied, the experimental paradigm utilized, and the choice of the outcomes measured. Despite the divergent therapeutic regiment used in pre-clinical studies, a few LIU constants were identified for their therapeutic effect on peripheral nerve regeneration (i.e., morphological and functional improvement). First, LIU intensity needs to be between 200 and 500 mW/cm2. At lower intensity (≤100 mW/cm2), the beneficial effect of LIU is not observable (Daeschler et al., 2018a; Ito et al., 2020), while at higher intensity (≥1 W/cm2) the beneficial effect is reduced or absent (Hong et al., 1988; Mourad et al., 2001; Akhlaghi et al., 2012; Jiang et al., 2016). Second, to improve nerve regeneration, LIU should be applied repetitively, either every day or every other day and for a short period of time (between 1 and 10 min per application). Third, none of the studies have reported negative side effects resulting from LIU, such as limiting or impairing peripheral nerve regeneration (Table 1). However, because it has not been investigated yet, it is unclear if a longer and/or more repetitive application of LIU will be beneficial or become detrimental for nerve regeneration.
In summary, multiple advances made from pre-clinical studies lead to the current consensus that LIU application promotes peripheral nerve regeneration after peripheral nerve injuries (Hong et al., 1988; Mourad et al., 2001; Crisci and Ferreira, 2002; Chang and Hsu, 2004; Chang et al., 2005; Raso et al., 2005; Chen et al., 2010; Park et al., 2010; Akhlaghi et al., 2012; Oliveira et al., 2012; Jahromy et al., 2013; Kim et al., 2013; Lv et al., 2015; Jiang et al., 2016; Ni et al., 2017; Ito et al., 2020; Wang et al., 2021). More precisely, it was shown that LIU could: (i) increase the number, diameter, or the myelination of axon distal to the lesion site; (ii) improve nerve conduction velocities (NCV) and compound muscle action potentials (CMAP); and (iii) enhance functional recovery after nerve injury (Table 1) (for review, see Peng et al., 2020). In addition, a few studies have shown that application of LIU on injured nerves is sufficient to alter gene regulation of neurotrophic factors, cytokines, or promyelinating genes during peripheral nerve regeneration (Chen et al., 2010; Jahromy et al., 2013; Ito et al., 2020; Wang et al., 2021). However, despite these encouraging studies, LIU application on peripheral nerve injury is not considered, possibly because the precise cellular and molecular mechanisms supporting the therapeutic effect of LIU during peripheral nerve regeneration are still not understood.
Effect of Low-Intensity Ultrasound on Schwann Cells
During peripheral nerve regeneration, repair Schwann cells fulfill a sequence of supportive functions for injured axons to survive, regenerate and reinnervate their tissue target. These include the expression of trophic factors to prevent neuronal death, the expression of cytokines to recruit macrophages, the autophagy of myelin debris, the formation of regeneration tracks to guide axonal regrowth, and eventually the remyelination of axons. Therefore, LIU regenerative effects could be mediated through repair Schwann cells and their numerous pro-regenerative properties that are essential to the nerve repair. While all morphological and electrophysiological outcomes observed in vivo in peripheral nerves after injury suggest that LIU acts on repair Schwann cells (Table 1), specific assessments of repair Schwann cell function and their differentiation into myelinating or non-myelinating Schwann cells remains unstudied. In addition, only a few groups have looked at the effect of LIU on primary Schwann cells in vitro (Zhang et al., 2009; Tsuang et al., 2011; Yue et al., 2016; Ren et al., 2018; Table 2). A consistent effect of LIU on Schwann cells in vitro (observed in 3 out of 4 studies) is an increase of Schwann cell proliferation following the first days after LIU application (Zhang et al., 2009; Tsuang et al., 2011; Ren et al., 2018). Ren et al. (2018) proposed that, the increased Schwann cell proliferation was mediated by enhancing cyclin D1 expression, similar to what was found with LIU in other cell types (i.e., mesenchymal stem cells and chondrocytes) (Takeuchi et al., 2008; Ling et al., 2017; Xie et al., 2019). Yet, while LIU may activate mitogenic signals in Schwann cells, the increase in repair Schwann cell proliferation following LIU application has yet to be studied in vivo. In addition, it is now known that proliferation is not critical for peripheral nerve regeneration in cyclin D1-null mice (Yang et al., 2008), contrasting with Ren et al. (2018) hypothesis and implying that further studies are necessary to identify the molecular mechanisms responsible for the LIU-mediated nerve repair.
Mechanisms of Action of Low-Intensity Ultrasound on Schwann Cells
Neurotrophic Factors
Following LIU, the observed increase in the number of myelinated axons, as well as the improvement in CMAP (Jahromy et al., 2013; Jiang et al., 2016; Ni et al., 2017), suggests that LIU improves the regrowth of axons. One hypothesis is that the regrowth of axons is mediated through the secretion of neurotrophic factors (i.e., NT-3, FGF, NGF, BDNF, and GDNF) by Schwann cells. Neurotrophic factors have been shown to promote neuroprotection, axonal regrowth and even myelinogenesis following peripheral nerve injury (for review, see Li et al., 2020). Six studies, using various therapeutic regiment, have looked at the effect of LIU on neurotrophic factor expression in Schwann cells (Zhang et al., 2009; Chen et al., 2010; Jahromy et al., 2013; Ren et al., 2018; Ito et al., 2020; Wang et al., 2021). However, it remains unclear how parameter changes in LIU show contrasting effects on neurotrophic factor secretion and how neurotrophic factor secretion will be modulated by LIU after more severe injury (nerve cut). Thus, further comprehensive in vitro and in vivo studies are needed to clarify how different LIU therapeutic regimens regulate Schwann cell neurotrophic factor expression and secretion.
Pro-inflammatory Cytokines
Within a few hours following injury, repair Schwann cells release pro-inflammatory cytokines and interleukins (e.g., TNFα, IL-6) that promote the massive recruitment of macrophages distal to the injury. Macrophages, in conjunction with repair Schwann cells, clean the myelin debris and help restructure the extracellular matrix. In addition, cytokines (e.g., IL-6) have direct pro-regenerative roles; promoting axonal (Hirota et al., 1996) and blood vessel (Cattin et al., 2015) regrowth at the axonal stump. One group showed that 7 days after nerve crush injury in rats, LIU suppressed the expression of inflammatory cytokines TNFα and IL-6, suggesting that LIU attenuate the pro-inflammatory response during peripheral nerve regeneration (Ito et al., 2020). While the positive modulation of the inflammatory response could improve peripheral nerve regeneration (for review, see Dubový et al., 2013), more comprehensive studies are necessary to decipher how the effect of LIU on the inflammatory responses participates in the LIU-mediated functional reinnervation improvement.
Schwann Cell Redifferentiation and Remyelination
Low-intensity ultrasound in peripheral nerves following injury is consistently found to promote myelin thickening and increase NCV (Crisci and Ferreira, 2002; Chen et al., 2010; Park et al., 2010; Kim et al., 2013; Lv et al., 2015; Jiang et al., 2016; Ni et al., 2017). These observations suggest that application of LIU promotes repair Schwann cell redifferentiation into myelinating Schwann cells and/or remyelination. Yue et al. showed that in vitro application of LIU on Schwann cells increases the expression of proteins involved in Schwann cells myelination: ErbB3; a receptor of juxtracrine and autocrine promyelinating neuregulin 1, EGR2; a transcriptional regulator for Schwann cell myelination, and MBP; a major myelin protein (Yue et al., 2016). However, considering this study was performed on Schwann cells in culture in non-myelinating conditions, the promyelinating effect of LIU on myelin wrapping remains to be demonstrated either in vivo or in Schwann cell/neuron myelinating co-culture.
Mechanisms of Action of Low-Intensity Ultrasound Independent of Schwann Cells
While most data support that the therapeutic effect of LIU on peripheral nerve regeneration is mediated by a direct effect on repair Schwann cells, an alternative hypothesis is that the improvement following LIU application is mediated directly through axons. Ventre et al. (2018, 2021) demonstrated in vitro that application of LIU on dorsal root ganglia neurons increased neurite outgrowth by two-fold compared to untreated controls, possibly by activating the Netrin-1/DCC pathway (Wen et al., 2021). In addition, it was suggested that LIU promoted axonal regrowth through the decrease of axonal semaphorin 3A expression, an inhibitor of axonal regeneration, and the decrease of GSK-3β, a potential inhibitor of axonal regrowth (Ito et al., 2020). However, because of conflicting reports on the role of GSK-3β signaling as either a beneficial or detrimental pathway for axon regeneration (Ogata et al., 2004; Zhou et al., 2004; Dill et al., 2008; Kim and Snider, 2011), the modulation of GSK-3β signaling by LIU and it contribution to nerve repair remains unclear. Following peripheral nerve injury, LIU could modulate myeloid cells (innate immune responses) (Xu et al., 2021) or vascular endothelial cells (angiogenesis) (de Lucas et al., 2020). The effect of LIU on these cells during peripheral nerve regeneration context have not been studied.
New Avenues of Research on Identifying the Low-Intensity Ultrasound-Mediated Sensing Mechanisms in Schwann Cells
A major gap of knowledge in the current field is how LIU is sensed by Schwann cells. In this review, we analyzed the known effects of LIU in other cellular systems as they may be translated to Schwann cells. LIU application was initially implicated for the treatment of bone fracture and arthritis, thus most of the known LIU-sensitive pathways were established in vitro from articular joint cell types. In cartilage and synovial cells, application of LIU increases the expression of extracellular matrix (ECM) components which in turns activates ECM-bound receptor responses such as the integrin/FAK/PI3K/AKT pathway (Choi et al., 2007; Takeuchi et al., 2008; Naito et al., 2010; Whitney et al., 2012; Cheng et al., 2014; Sato et al., 2014; Xia et al., 2015; Zhang et al., 2016; Ding et al., 2020). LIU was found to activate similar pathways in other cell types, such as fibroblasts, keratinocytes and mesenchymal stem cells (Bohari et al., 2012; Leng et al., 2018; Chen et al., 2019; Hormozi-Moghaddam et al., 2021) which strongly suggest that the integrin/FAK/PI3K/AKT pathway activation by LIU may not be limited to certain cell-type. Macromolecules of the ECM and basal lamina, such as collagen, laminin, and fibronectin, constitute the microenvironment of Schwann cells. Schwann cells harbor receptors for the ECM, including Integrins, GPR126, and dystroglycan. The ECM interact with their receptors which activates cascades of phosphorylation in part through RhoGTPases, Focal Adhesion Kinase (FAK) and Integrin-Linked Kinase (ILK). These receptors and kinases contribute to the transmission of mechanical signals from the ECM to the nucleus (for review, see Martino et al., 2018), and are critical for Schwann cell development and myelination (for review, see Monk et al., 2015; Feltri et al., 2016; Wilson et al., 2021). Importantly, those same receptors and kinases have also been shown to be required for peripheral nerve regeneration following injury (Werner et al., 2000; Akassoglou et al., 2002; Chen and Strickland, 2003; Van der Zee et al., 2008; Pereira et al., 2009; Chen et al., 2015; Mogha et al., 2016; Atherton et al., 2017; Zainul et al., 2018). In addition, recent studies have shown that stimuli from the ECM can lead to the reorganization of the actin cytoskeleton, which induces the activation of transcriptional coactivators YAP/TAZ (Dupont et al., 2011; Zhao et al., 2012; Aragona et al., 2013; Totaro et al., 2017), and Rho GTPase (Aragona et al., 2013; Reginensi et al., 2013). Two studies have shown that LIU application leads to the activation of YAP/TAZ in endothelial cells and retinal ganglion cells (Xu et al., 2018; Zhou et al., 2018). This suggests that LIU application could modulate diverse pathways in Schwann cells through changes in ECM composition, architecture, and the alteration of the interactions between the ECM and ECM-bound receptors. Yet, it is likely that in response to LIU, mechanotransduction would initiate multiple signaling pathways at once, and these pathways can have significant crosstalk and overlap, making it difficult to associate the observed improvement in peripheral nerve regeneration to one specific pathway.
Conclusion
Over the last 20 years, both pre-clinical and clinical studies have attempted to characterize the effect of LIU on peripheral nerve regeneration. There are many compelling evidence that application of LIU increase the number, diameter, or the myelination of axon distal to the lesion site, improve functional outcomes and globally enhance peripheral nerve regeneration after nerve injury. Yet, there is still a need for studies with comprehensive mechanistic results to understand how LIU sound waves affect the regenerative processes following peripheral nerve injury. While it is likely that the effect of LIU is mediated through repair Schwann cells, which are the central hub for peripheral nerve regeneration, the demonstration is still lacking. It is still unclear how LIU affects ECM composition, ECM-mediated signaling pathways; which could mediate repair Schwann cells’ fate during peripheral nerve regeneration. In addition, independently from Schwann cells, the effect of LIU on immune and vascular cells, known to contribute to nerve repair is currently unknown. Future studies should also evaluate the effect of LIU on neuropathic pain and investigate LIU in sensory nerves, as all functional studies have focused on motor outcomes so far. There are a few reports indicating that peripheral nerve regeneration may be sexually dimorphic as axonal regrowth seems to be more efficient in males (Stenberg and Dahlin, 2014), while remyelination post-injury is more efficient in females (Kovacic et al., 2004; Tong et al., 2015). Thus, future research will need to carefully evaluate how peripheral nerve regeneration mediated by LIU may differ between sexes. Further understanding of LIU’s modus operandi on peripheral nerve injury would likely support further clinical trial assessing the therapeutic effect of LIU during peripheral nerve regeneration in humans.
Author Contributions
JA, SB, and YP wrote the manuscript. SB, SS, and YP revised the manuscript. YP created the tables. All authors approved the final version of the manuscript for publication.
Funding
YP is supported by the National Institutes of Health (NS110627). SS is supported by the National Institutes of Health (HD092894).
Conflict of Interest
The authors declare that the research was conducted in the absence of any commercial or financial relationships that could be construed as a potential conflict of interest.
Publisher’s Note
All claims expressed in this article are solely those of the authors and do not necessarily represent those of their affiliated organizations, or those of the publisher, the editors and the reviewers. Any product that may be evaluated in this article, or claim that may be made by its manufacturer, is not guaranteed or endorsed by the publisher.
References
Akassoglou, K., Yu, W. M., Akpinar, P., and Strickland, S. (2002). Fibrin inhibits peripheral nerve remyelination by regulating Schwann cell differentiation. Neuron 33, 861–875. doi: 10.1016/s0896-6273(02)00617-7
Akhlaghi, Z., Mobarakeh, J. I., Mokhtari, M., Behnam, H., Rahimi, A. A., Khajeh Hosseini, M. S., et al. (2012). The effects of altered ultrasound parameters on the recovery of sciatic nerve injury. Iran. Biomed. J. 16, 107–112. doi: 10.6091/ibj.942.2012
Aragona, M., Panciera, T., Manfrin, A., Giulitti, S., Michielin, F., Elvassore, N., et al. (2013). A mechanical checkpoint controls multicellular growth through YAP/TAZ regulation by actin-processing factors. Cell 154, 1047–1059. doi: 10.1016/j.cell.2013.07.042
Atherton, P., Lausecker, F., Harrison, A., and Ballestrem, C. (2017). Low-intensity pulsed ultrasound promotes cell motility through vinculin-controlled Rac1 GTPase activity. J. Cell Sci. 130, 2277–2291. doi: 10.1242/jcs.192781
Bergmeister, K. D., Grosse-Hartlage, L., Daeschler, S. C., Rhodius, P., Bocker, A., Beyersdorff, M., et al. (2020). Acute and long-term costs of 268 peripheral nerve injuries in the upper extremity. PLoS One 15:e0229530. doi: 10.1371/journal.pone.0229530
Bohari, S. P., Grover, L. M., and Hukins, D. W. (2012). Pulsed-low intensity ultrasound enhances extracellular matrix production by fibroblasts encapsulated in alginate. J. Tissue Eng. 3:2041731412454672. doi: 10.1177/2041731412454672
Cattin, A. L., Burden, J. J., Van Emmenis, L., Mackenzie, F. E., Hoving, J. J., Garcia Calavia, N., et al. (2015). Macrophage-Induced Blood Vessels Guide Schwann Cell-Mediated Regeneration of Peripheral Nerves. Cell 162, 1127–1139. doi: 10.1016/j.cell.2015.07.021
Chang, C. J., and Hsu, S. H. (2004). The effects of low-intensity ultrasound on peripheral nerve regeneration in poly(DL-lactic acid-co-glycolic acid) conduits seeded with Schwann cells. Ultrasound Med. Biol. 30, 1079–1084. doi: 10.1016/j.ultrasmedbio.2004.06.005
Chang, C. J., Hsu, S. H., Lin, F. T., Chang, H., and Chang, C. S. (2005). Low-intensity-ultrasound-accelerated nerve regeneration using cell-seeded poly(D,L-lactic acid-co-glycolic acid) conduits: an in vivo and in vitro study. J. Biomed. Mater. Res. B Appl. Biomater. 75, 99–107. doi: 10.1002/jbm.b.30269
Chen, J., Jiang, J., Wang, W., Qin, J., Chen, J., Chen, W., et al. (2019). Low intensity pulsed ultrasound promotes the migration of bone marrow- derived mesenchymal stem cells via activating FAK-ERK1/2 signalling pathway. Artif. Cells Nanomed. Biotechnol. 47, 3603–3613. doi: 10.1080/21691401.2019.1657878
Chen, P., Cescon, M., Zuccolotto, G., Nobbio, L., Colombelli, C., Filaferro, M., et al. (2015). Collagen VI regulates peripheral nerve regeneration by modulating macrophage recruitment and polarization. Acta Neuropathol. 129, 97–113. doi: 10.1007/s00401-014-1369-9
Chen, W. Z., Qiao, H., Zhou, W., Wu, J., and Wang, Z. B. (2010). Upgraded nerve growth factor expression induced by low-intensity continuous-wave ultrasound accelerates regeneration of neurotometicly injured sciatic nerve in rats. Ultrasound Med. Biol. 36, 1109–1117. doi: 10.1016/j.ultrasmedbio.2010.04.014
Chen, Z. L., and Strickland, S. (2003). Laminin gamma1 is critical for Schwann cell differentiation, axon myelination, and regeneration in the peripheral nerve. J. Cell Biol. 163, 889–899. doi: 10.1083/jcb.200307068
Cheng, K., Xia, P., Lin, Q., Shen, S., Gao, M., Ren, S., et al. (2014). Effects of low-intensity pulsed ultrasound on integrin-FAK-PI3K/Akt mechanochemical transduction in rabbit osteoarthritis chondrocytes. Ultrasound Med. Biol. 40, 1609–1618. doi: 10.1016/j.ultrasmedbio.2014.03.002
Choi, B. H., Choi, M. H., Kwak, M. G., Min, B. H., Woo, Z. H., and Park, S. R. (2007). Mechanotransduction pathways of low-intensity ultrasound in C-28/I2 human chondrocyte cell line. Proc. Inst. Mech. Eng. H 221, 527–535. doi: 10.1243/09544119JEIM201
Crisci, A. R., and Ferreira, A. L. (2002). Low-intensity pulsed ultrasound accelerates the regeneration of the sciatic nerve after neurotomy in rats. Ultrasound Med. Biol. 28, 1335–1341. doi: 10.1016/s0301-5629(02)00576-8
Daeschler, S. C., Harhaus, L., Bergmeister, K. D., Boecker, A., Hoener, B., Kneser, U., et al. (2018a). Clinically available low intensity ultrasound devices do not promote axonal regeneration after peripheral nerve surgery-a preclinical investigation of an FDA-approved device. Front. Neurol. 9:1057. doi: 10.3389/fneur.2018.01057
Daeschler, S. C., Harhaus, L., Schoenle, P., Boecker, A., Kneser, U., and Bergmeister, K. D. (2018b). Ultrasound and shock-wave stimulation to promote axonal regeneration following nerve surgery: a systematic review and meta-analysis of preclinical studies. Sci. Rep. 8:3168. doi: 10.1038/s41598-018-21540-5
de Lucas, B., Perez, L. M., Bernal, A., and Galvez, B. G. (2020). Ultrasound therapy: experiences and perspectives for regenerative medicine. Genes 11:1086. doi: 10.3390/genes11091086
Dill, J., Wang, H., Zhou, F., and Li, S. (2008). Inactivation of glycogen synthase kinase 3 promotes axonal growth and recovery in the CNS. J. Neurosci. 28, 8914–8928. doi: 10.1523/JNEUROSCI.1178-08.2008
Ding, W., Du, D., and Chen, S. (2020). LIPUS promotes synthesis and secretion of extracellular matrix and reduces cell apoptosis in human osteoarthritis through upregulation of SOX9 expression. Int. J. Clin. Exp. Pathol. 13, 810–817.
Dubový, P., Jančálek, R., and Kubek, T. (2013). Role of inflammation and cytokines in peripheral nerve regeneration. Int. Rev. Neurobiol. 108, 173–206. doi: 10.1016/B978-0-12-410499-0.00007-1
Dupont, S., Morsut, L., Aragona, M., Enzo, E., Giulitti, S., Cordenonsi, M., et al. (2011). Role of YAP/TAZ in mechanotransduction. Nature 474, 179–183. doi: 10.1038/nature10137
Feltri, M. L., Poitelon, Y., and Previtali, S. C. (2016). How schwann cells sort axons: new concepts. Neuroscientist 22, 252–265. doi: 10.1177/1073858415572361
Grogan, S. P., and Mount, C. A. (2021). Ultrasound Physics and Instrumentation. Treasure Island, FL: StatPearls.
Haffey, P. R., Bansal, N., Kaye, E., Ottestad, E., Aiyer, R., Noori, S., et al. (2020). The regenerative potential of therapeutic ultrasound on neural tissue: a pragmatic review. Pain Med. 21, 1494–1506. doi: 10.1093/pm/pnaa090
Hirota, H., Kiyama, H., Kishimoto, T., and Taga, T. (1996). Accelerated nerve regeneration in mice by upregulated expression of interleukin (IL) 6 and IL-6 receptor after trauma. J. Exp. Med. 183, 2627–2634. doi: 10.1084/jem.183.6.2627
Hong, C. Z., Liu, H. H., and Yu, J. (1988). Ultrasound thermotherapy effect on the recovery of nerve conduction in experimental compression neuropathy. Arch. Phys. Med. Rehabil. 69, 410–414.
Hormozi-Moghaddam, Z., Mokhtari-Dizaji, M., Nilforoshzadeh, M.-A., and Bakhshandeh, M. (2021). Low-intensity ultrasound to induce proliferation and collagen I expression of adipose-derived mesenchymal stem cells and fibroblast cells in co-culture. Measurement 167:108280. doi: 10.1016/j.measurement.2020.108280
Ikai, H., Tamura, T., Watanabe, T., Itou, M., Sugaya, A., Iwabuchi, S., et al. (2008). Low-intensity pulsed ultrasound accelerates periodontal wound healing after flap surgery. J. Periodontal Res. 43, 212–216. doi: 10.1111/j.1600-0765.2007.01016.x
Ito, A., Wang, T., Nakahara, R., Kawai, H., Nishitani, K., Aoyama, T., et al. (2020). Ultrasound therapy with optimal intensity facilitates peripheral nerve regeneration in rats through suppression of pro-inflammatory and nerve growth inhibitor gene expression. PLoS One 15:e0234691. doi: 10.1371/journal.pone.0234691
Iwashina, T., Mochida, J., Miyazaki, T., Watanabe, T., Iwabuchi, S., Ando, K., et al. (2006). Low-intensity pulsed ultrasound stimulates cell proliferation and proteoglycan production in rabbit intervertebral disc cells cultured in alginate. Biomaterials 27, 354–361. doi: 10.1016/j.biomaterials.2005.06.031
Jahromy, F. Z., Behnam, H., Mansoori, K., Rahimi, A. A., Edalat, R., and Mobarake, J. I. (2013). The effect of ultrasound on the expression of CNTF gene, a possible cause of ultrasound influence on the rate of injured peripheral nerve regeneration. Australas. Phys. Eng. Sci. Med. 36, 323–329. doi: 10.1007/s13246-013-0216-y
Jeremias Junior, S. L., Camanho, G. L., Bassit, A. C., Forgas, A., Ingham, S. J., and Abdalla, R. J. (2011). Low-intensity pulsed ultrasound accelerates healing in rat calcaneus tendon injuries. J. Orthop. Sports Phys. Ther. 41, 526–531. doi: 10.2519/jospt.2011.3468
Jiang, W., Wang, Y., Tang, J., Peng, J., Wang, Y., Guo, Q., et al. (2016). Low-intensity pulsed ultrasound treatment improved the rate of autograft peripheral nerve regeneration in rat. Sci. Rep. 6:22773. doi: 10.1038/srep22773
Jiang, X., Savchenko, O., Li, Y., Qi, S., Yang, T., Zhang, W., et al. (2019). A review of low-intensity pulsed ultrasound for therapeutic applications. IEEE Trans. Biomed. Eng. 66, 2704–2718. doi: 10.1109/TBME.2018.2889669
Karsy, M., Watkins, R., Jensen, M. R., Guan, J., Brock, A. A., and Mahan, M. A. (2019). Trends and Cost Analysis of Upper Extremity Nerve Injury Using the National (Nationwide) Inpatient Sample. World Neurosurg 123, e488–e500. doi: 10.1016/j.wneu.2018.11.192
Kim, J. R., Oh, S. H., Kwon, G. B., Namgung, U., Song, K. S., Jeon, B. H., et al. (2013). Acceleration of peripheral nerve regeneration through asymmetrically porous nerve guide conduit applied with biological/physical stimulation. Tissue Eng. Part A 19, 2674–2685. doi: 10.1089/ten.TEA.2012.0735
Kim, W. Y., and Snider, W. D. (2011). Functions of GSK-3 signaling in development of the nervous system. Front. Mol. Neurosci. 4:44. doi: 10.3389/fnmol.2011.00044
Kovacic, U., Zele, T., Osredkar, J., Sketelj, J., and Bajrovic, F. F. (2004). Sex-related differences in the regeneration of sensory axons and recovery of nociception after peripheral nerve crush in the rat. Exp. Neurol. 189, 94–104. doi: 10.1016/j.expneurol.2004.05.015
Lad, S. P., Nathan, J. K., Schubert, R. D., and Boakye, M. (2010). Trends in median, ulnar, radial, and brachioplexus nerve injuries in the United States. Neurosurgery 66, 953–960. doi: 10.1227/01.neu.0000368545.83463.91
Leng, X., Shang, J., Gao, D., and Wu, J. (2018). Low-intensity pulsed ultrasound promotes proliferation and migration of HaCaT keratinocytes through the PI3K/AKT and JNK pathways. Braz. J. Med. Biol. Res. 51, e7862. doi: 10.1590/1414-431X20187862
Li, R., Li, D. H., Zhang, H. Y., Wang, J., Li, X. K., and Xiao, J. (2020). Growth factors-based therapeutic strategies and their underlying signaling mechanisms for peripheral nerve regeneration. Acta Pharmacol. Sin. 41, 1289–1300. doi: 10.1038/s41401-019-0338-1
Ling, L., Wei, T., He, L., Wang, Y., Feng, X., Zhang, W., et al. (2017). Low-intensity pulsed ultrasound activates ERK1/2 and PI3K-Akt signalling pathways and promotes the proliferation of human amnion-derived mesenchymal stem cells. Cell Prolif. 50:e12383. doi: 10.1111/cpr.12383
Lv, Y., Nan, P., Chen, G., Sha, Y., Xia, B., and Yang, L. (2015). In vivo repair of rat transected sciatic nerve by low-intensity pulsed ultrasound and induced pluripotent stem cells-derived neural crest stem cells. Biotechnol. Lett. 37, 2497–2506. doi: 10.1007/s10529-015-1939-5
Martino, F., Perestrelo, A. R., Vinarsky, V., Pagliari, S., and Forte, G. (2018). Cellular mechanotransduction: from tension to function. Front. Physiol. 9:824. doi: 10.3389/fphys.2018.00824
Menorca, R. M., Fussell, T. S., and Elfar, J. C. (2013). Nerve physiology: mechanisms of injury and recovery. Hand Clin. 29, 317–330. doi: 10.1016/j.hcl.2013.04.002
Mogha, A., Harty, B. L., Carlin, D., Joseph, J., Sanchez, N. E., Suter, U., et al. (2016). Gpr126/Adgrg6 has schwann cell autonomous and nonautonomous functions in peripheral nerve injury and repair. J. Neurosci. 36, 12351–12367. doi: 10.1523/JNEUROSCI.3854-15.2016
Monk, K. R., Feltri, M. L., and Taveggia, C. (2015). New insights on Schwann cell development. Glia 63, 1376–1393. doi: 10.1002/glia.22852
Mourad, P. D., Lazar, D. A., Curra, F. P., Mohr, B. C., Andrus, K. C., Avellino, A. M., et al. (2001). Ultrasound accelerates functional recovery after peripheral nerve damage. Neurosurgery 48, 1136–1140. doi: 10.1097/00006123-200105000-00035
Naito, K., Watari, T., Muta, T., Furuhata, A., Iwase, H., Igarashi, M., et al. (2010). Low-intensity pulsed ultrasound (LIPUS) increases the articular cartilage type II collagen in a rat osteoarthritis model. J. Orthop. Res. 28, 361–369. doi: 10.1002/jor.20995
Nakao, J., Fujii, Y., Kusuyama, J., Bandow, K., Kakimoto, K., Ohnishi, T., et al. (2014). Low-intensity pulsed ultrasound (LIPUS) inhibits LPS-induced inflammatory responses of osteoblasts through TLR4-MyD88 dissociation. Bone 58, 17–25. doi: 10.1016/j.bone.2013.09.018
Ni, X. J., Wang, X. D., Zhao, Y. H., Sun, H. L., Hu, Y. M., Yao, J., et al. (2017). The effect of low-intensity ultrasound on brain-derived neurotropic factor expression in a rat sciatic nerve crushed injury model. Ultrasound Med. Biol. 43, 461–468. doi: 10.1016/j.ultrasmedbio.2016.09.017
Noble, J., Munro, C. A., Prasad, V. S., and Midha, R. (1998). Analysis of upper and lower extremity peripheral nerve injuries in a population of patients with multiple injuries. J. Trauma 45, 116–122. doi: 10.1097/00005373-199807000-00025
Ogata, T., Iijima, S., Hoshikawa, S., Miura, T., Yamamoto, S., Oda, H., et al. (2004). Opposing extracellular signal-regulated kinase and Akt pathways control Schwann cell myelination. J. Neurosci. 24, 6724–6732. doi: 10.1523/JNEUROSCI.5520-03.2004
Oliveira, F. B., Pereira, V. M., da Trindade, A. P., Shimano, A. C., Gabriel, R. E., and Borges, A. P. (2012). Action of therapeutic laser and ultrasound in peripheral nerve regeneration. Acta Ortop. Bras. 20, 98–103. doi: 10.1590/S1413-78522012000200008
Page, M. J., McKenzie, J. E., Bossuyt, P. M., Boutron, I., Hoffmann, T. C., Mulrow, C. D., et al. (2021). The PRISMA 2020 statement: an updated guideline for reporting systematic reviews. BMJ 372:n71. doi: 10.1136/bmj.n71
Park, S. C., Oh, S. H., Seo, T. B., Namgung, U., Kim, J. M., and Lee, J. H. (2010). Ultrasound-stimulated peripheral nerve regeneration within asymmetrically porous PLGA/Pluronic F127 nerve guide conduit. J. Biomed. Mater. Res. B Appl. Biomater. 94, 359–366. doi: 10.1002/jbm.b.31659
Peng, D. Y., Reed-Maldonado, A. B., Lin, G. T., Xia, S. J., and Lue, T. F. (2020). Low-intensity pulsed ultrasound for regenerating peripheral nerves: potential for penile nerve. Asian J. Androl. 22, 335–341. doi: 10.4103/aja.aja_95_19
Pereira, J. A., Benninger, Y., Baumann, R., Goncalves, A. F., Ozcelik, M., Thurnherr, T., et al. (2009). Integrin-linked kinase is required for radial sorting of axons and Schwann cell remyelination in the peripheral nervous system. J. Cell Biol. 185, 147–161. doi: 10.1083/jcb.200809008
Raso, V. V., Barbieri, C. H., Mazzer, N., and Fasan, V. S. (2005). Can therapeutic ultrasound influence the regeneration of peripheral nerves? J. Neurosci. Methods 142, 185–192. doi: 10.1016/j.jneumeth.2004.08.016
Reginensi, A., Scott, R. P., Gregorieff, A., Bagherie-Lachidan, M., Chung, C., Lim, D. S., et al. (2013). Yap- and Cdc42-dependent nephrogenesis and morphogenesis during mouse kidney development. PLoS Genet. 9:e1003380. doi: 10.1371/journal.pgen.1003380
Ren, C., Chen, X., Du, N., Geng, S., Hu, Y., Liu, X., et al. (2018). Low-intensity pulsed ultrasound promotes Schwann cell viability and proliferation via the GSK-3beta/beta-catenin signaling pathway. Int. J. Biol. Sci. 14, 497–507. doi: 10.7150/ijbs.22409
Ren, L., Yang, Z., Song, J., Wang, Z., Deng, F., and Li, W. (2013). Involvement of p38 MAPK pathway in low intensity pulsed ultrasound induced osteogenic differentiation of human periodontal ligament cells. Ultrasonics 53, 686–690. doi: 10.1016/j.ultras.2012.10.008
Sato, M., Nagata, K., Kuroda, S., Horiuchi, S., Nakamura, T., Karima, M., et al. (2014). Low-intensity pulsed ultrasound activates integrin-mediated mechanotransduction pathway in synovial cells. Ann. Biomed. Eng. 42, 2156–2163. doi: 10.1007/s10439-014-1081-x
Stenberg, L., and Dahlin, L. B. (2014). Gender differences in nerve regeneration after sciatic nerve injury and repair in healthy and in type 2 diabetic Goto-Kakizaki rats. BMC Neurosci. 15:107. doi: 10.1186/1471-2202-15-107
Su, W. S., Wu, C. H., Chen, S. F., and Yang, F. Y. (2017). Low-intensity pulsed ultrasound improves behavioral and histological outcomes after experimental traumatic brain injury. Sci. Rep. 7:15524. doi: 10.1038/s41598-017-15916-2
Sunderland, S. (1951). A classification of peripheral nerve injuries producing loss of function. Brain 74, 491–516. doi: 10.1093/brain/74.4.491
Takebe, H., Nakanishi, Y., Hirose, Y., and Ochi, M. (2014). Effect of low intensity pulsed ultrasound stimulation on sinus augmentation in rabbits. Clin. Oral Implants Res. 25, 735–741. doi: 10.1111/clr.12136
Takeuchi, R., Ryo, A., Komitsu, N., Mikuni-Takagaki, Y., Fukui, A., Takagi, Y., et al. (2008). Low-intensity pulsed ultrasound activates the phosphatidylinositol 3 kinase/Akt pathway and stimulates the growth of chondrocytes in three-dimensional cultures: a basic science study. Arthritis Res. Ther. 10:R77. doi: 10.1186/ar2451
Taylor, C. A., Braza, D., Rice, J. B., and Dillingham, T. (2008). The incidence of peripheral nerve injury in extremity trauma. Am. J. Phys. Med. Rehabil. 87, 381–385. doi: 10.1097/PHM.0b013e31815e6370
Tong, L. L., Ding, Y. Q., Jing, H. B., Li, X. Y., and Qi, J. G. (2015). Differential motor and sensory functional recovery in male but not female adult rats is associated with remyelination rather than axon regeneration after sciatic nerve crush. Neuroreport 26, 429–437. doi: 10.1097/WNR.0000000000000366
Totaro, A., Castellan, M., Battilana, G., Zanconato, F., Azzolin, L., Giulitti, S., et al. (2017). YAP/TAZ link cell mechanics to Notch signalling to control epidermal stem cell fate. Nat. Commun. 8:15206. doi: 10.1038/ncomms15206
Tsuang, Y. H., Liao, L. W., Chao, Y. H., Sun, J. S., Cheng, C. K., Chen, M. H., et al. (2011). Effects of low intensity pulsed ultrasound on rat Schwann cells metabolism. Artif. Organs 35, 373–383. doi: 10.1111/j.1525-1594.2010.01086.x
Uddin, S. M. Z., Komatsu, D. E., Motyka, T., and Petterson, S. (2021). Low-Intensity continuous ultrasound therapies-A systemic review of current state-of-the-art and future perspectives. J. Clin. Med. 10:2698. doi: 10.3390/jcm10122698
Van der Zee, C. E., Kreft, M., Beckers, G., Kuipers, A., and Sonnenberg, A. (2008). Conditional deletion of the Itgb4 integrin gene in Schwann cells leads to delayed peripheral nerve regeneration. J. Neurosci. 28, 11292–11303. doi: 10.1523/JNEUROSCI.3068-08.2008
Ventre, D., Puzan, M., Ashbolt, E., and Koppes, A. (2018). Enhanced total neurite outgrowth and secondary branching in dorsal root ganglion neurons elicited by low intensity pulsed ultrasound. J. Neural Eng. 15:046013. doi: 10.1088/1741-2552/aabeba
Ventre, D. M., Cluff, A., Gagnon, C., Diaz Vera, D., Koppes, R. A., and Koppes, A. N. (2021). The effects of low intensity focused ultrasonic stimulation on dorsal root ganglion neurons and Schwann cells in vitro. J. Neurosci. Res. 99, 374–391. doi: 10.1002/jnr.24700
Wang, T., Ito, A., Xu, S., Kawai, H., Kuroki, H., and Aoyama, T. (2021). Low-Intensity Pulsed Ultrasound Prompts Both Functional and Histologic Improvements While Upregulating the Brain-Derived Neurotrophic Factor Expression after Sciatic Crush Injury in Rats. Ultrasound Med. Biol. 47, 1586–1595. doi: 10.1016/j.ultrasmedbio.2021.02.009
Wen, J., Deng, X., Huang, C., An, Z., and Liu, M. (2021). Low-Intensity Pulsed Ultrasound Enhanced Neurite Guidance Growth through Netrin-1/DCC Signal Pathway in Primary Cultured Cortical Neurons of Rats. ACS Chem. Neurosci. 12, 1931–1939. doi: 10.1021/acschemneuro.1c00020
Werner, A., Willem, M., Jones, L. L., Kreutzberg, G. W., Mayer, U., and Raivich, G. (2000). Impaired axonal regeneration in alpha7 integrin-deficient mice. J. Neurosci. 20, 1822–1830. doi: 10.1523/JNEUROSCI.20-05-01822.2000
Whitney, N. P., Lamb, A. C., Louw, T. M., and Subramanian, A. (2012). Integrin-mediated mechanotransduction pathway of low-intensity continuous ultrasound in human chondrocytes. Ultrasound Med. Biol. 38, 1734–43. doi: 10.1016/j.ultrasmedbio.2012.06.002
Wilson, E. R., Della-Flora Nunes, G., Weaver, M. R., Frick, L. R., and Feltri, M. L. (2021). Schwann cell interactions during the development of the peripheral nervous system. Dev. Neurobiol. 81, 464–489. doi: 10.1002/dneu.22744
Xia, P., Ren, S., Lin, Q., Cheng, K., Shen, S., Gao, M., et al. (2015). Low-intensity pulsed ultrasound affects chondrocyte extracellular matrix production via an integrin-mediated p38 MAPK signaling pathway. Ultrasound Med. Biol. 41, 1690–1700. doi: 10.1016/j.ultrasmedbio.2015.01.014
Xie, S., Jiang, X., Wang, R., Hua, Y., Zhou, S., Yang, Y., et al. (2019). Low-intensity pulsed ultrasound promotes the proliferation of human bone mesenchymal stem cells by activating PI3K/AKt signaling pathways. J. Cell. Biochem. 120, 15823–15833. doi: 10.1002/jcb.28853
Xin, Z., Lin, G., Lei, H., Lue, T. F., and Guo, Y. (2016). Clinical applications of low-intensity pulsed ultrasound and its potential role in urology. Transl. Androl. Urol. 5, 255–266. doi: 10.21037/tau.2016.02.04
Xu, M., Wang, L., Wu, S., Dong, Y., Chen, X., Wang, S., et al. (2021). Review on experimental study and clinical application of low-intensity pulsed ultrasound in inflammation. Quant. Imaging Med. Surg. 11, 443–462. doi: 10.21037/qims-20-680
Xu, X. M., Xu, T. M., Wei, Y. B., Gao, X. X., Sun, J. C., Wang, Y., et al. (2018). Low-intensity pulsed ultrasound treatment accelerates angiogenesis by activating YAP/TAZ in human umbilical vein endothelial cells. Ultrasound Med. Biol. 44, 2655–2661. doi: 10.1016/j.ultrasmedbio.2018.07.007
Yang, D. P., Zhang, D. P., Mak, K. S., Bonder, D. E., Pomeroy, S. L., and Kim, H. A. (2008). Schwann cell proliferation during Wallerian degeneration is not necessary for regeneration and remyelination of the peripheral nerves: axon-dependent removal of newly generated Schwann cells by apoptosis. Mol. Cell. Neurosci. 38, 80–88. doi: 10.1016/j.mcn.2008.01.017
Yue, Y., Yang, X., Zhang, L., Xiao, X., Nabar, N. R., Lin, Y., et al. (2016). Low-intensity pulsed ultrasound upregulates pro-myelination indicators of Schwann cells enhanced by co-culture with adipose-derived stem cells. Cell Prolif. 49, 720–728. doi: 10.1111/cpr.12298
Zainul, Z., Heikkinen, A., Koivisto, H., Rautalahti, I., Kallio, M., Lin, S., et al. (2018). Collagen XIII is required for neuromuscular synapse regeneration and functional recovery after peripheral nerve injury. J. Neurosci. 38, 4243–4258. doi: 10.1523/JNEUROSCI.3119-17.2018
Zhang, H., Lin, X., Wan, H., Li, J. H., and Li, J. M. (2009). Effect of low-intensity pulsed ultrasound on the expression of neurotrophin-3 and brain-derived neurotrophic factor in cultured Schwann cells. Microsurgery 29, 479–485. doi: 10.1002/micr.20644
Zhang, X., Hu, Z., Hao, J., and Shen, J. (2016). Low Intensity Pulsed Ultrasound Promotes the Extracellular Matrix Synthesis of Degenerative Human Nucleus Pulposus Cells Through FAK/PI3K/Akt Pathway. Spine 41, E248–E254. doi: 10.1097/BRS.0000000000001220
Zhao, B., Li, L., Wang, L., Wang, C. Y., Yu, J., and Guan, K. L. (2012). Cell detachment activates the Hippo pathway via cytoskeleton reorganization to induce anoikis. Genes Dev. 26, 54–68. doi: 10.1101/gad.173435.111
Zhao, X., Zhao, G., Shi, Z., Zhou, C., Chen, Y., Hu, B., et al. (2017). Low-intensity pulsed ultrasound (LIPUS) prevents periprosthetic inflammatory loosening through FBXL2-TRAF6 ubiquitination pathway. Sci. Rep. 7:45779. doi: 10.1038/srep45779
Zhou, F. Q., Zhou, J., Dedhar, S., Wu, Y. H., and Snider, W. D. (2004). NGF-induced axon growth is mediated by localized inactivation of GSK-3beta and functions of the microtubule plus end binding protein APC. Neuron 42, 897–912. doi: 10.1016/j.neuron.2004.05.011
Zhou, J. X., Liu, Y. J., Chen, X., Zhang, X., Xu, J., Yang, K., et al. (2018). Low-intensity pulsed ultrasound protects retinal ganglion cell from optic nerve injury induced apoptosis via yes associated protein. Front. Cell. Neurosci. 12:160. doi: 10.3389/fncel.2018.00160
Keywords: ultrasound, peripheral nerve regeneration, Schwann cells, LIU, LIPUS
Citation: Acheta J, Stephens SBZ, Belin S and Poitelon Y (2022) Therapeutic Low-Intensity Ultrasound for Peripheral Nerve Regeneration – A Schwann Cell Perspective. Front. Cell. Neurosci. 15:812588. doi: 10.3389/fncel.2021.812588
Received: 10 November 2021; Accepted: 09 December 2021;
Published: 05 January 2022.
Edited by:
Antje Kroner, Medical College of Wisconsin, United StatesReviewed by:
Víctor Carriel, University of Granada, SpainCopyright © 2022 Acheta, Stephens, Belin and Poitelon. This is an open-access article distributed under the terms of the Creative Commons Attribution License (CC BY). The use, distribution or reproduction in other forums is permitted, provided the original author(s) and the copyright owner(s) are credited and that the original publication in this journal is cited, in accordance with accepted academic practice. No use, distribution or reproduction is permitted which does not comply with these terms.
*Correspondence: Sophie Belin, belins@amc.edu; Yannick Poitelon, poitely@amc.edu