Memory consolidation in honey bees is enhanced by down-regulation of Down syndrome cell adhesion molecule and changes its alternative splicing
- 1School of Biosciences, College of Life and Environmental Sciences, University of Birmingham, Birmingham, United Kingdom
- 2Birmingham Centre for Genome Biology, University of Birmingham, Birmingham, United Kingdom
- 3Research Center on Animal Cognition (CRCA), Center for Integrative Biology (CBI), CNRS, UPS, Toulouse University, Toulouse, France
- 4Department of Life Science, Faculty of Health, Education and Life Sciences, Birmingham City University, Birmingham, United Kingdom
- 5College of Medical and Dental Sciences, Institute of Cancer and Genomics Sciences, University of Birmingham, Birmingham, United Kingdom
- 6Institut Universitaire de France (IUF), Paris, France
Down syndrome cell adhesion molecule (Dscam) gene encodes a cell adhesion molecule required for neuronal wiring. A remarkable feature of arthropod Dscam is massive alternative splicing generating thousands of different isoforms from three variable clusters of alternative exons. Dscam expression and diversity arising from alternative splicing have been studied during development, but whether they exert functions in adult brains has not been determined. Here, using honey bees, we find that Dscam expression is critically linked to memory retention as reducing expression by RNAi enhances memory after reward learning in adult worker honey bees. Moreover, alternative splicing of Dscam is altered in all three variable clusters after learning. Since identical Dscam isoforms engage in homophilic interactions, these results suggest a mechanism to alter inclusion of variable exons during memory consolidation to modify neuronal connections for memory retention.
Introduction
Memory consolidation is the process through which memories are stabilized and stored into long-term memory (Kandel et al., 2014). Gene expression through transcription of genes plays pivotal roles in this process (Alberini et al., 1994; Clayton, 2000; Alberini, 2009; Kandel et al., 2014). During transcription, splicing out of introns allows to alter coding content through alternative splicing, which is particularly abundant in the brain in genes coding for ion channels and cell adhesion molecules (Soller, 2006; Vuong et al., 2016; Ule and Blencowe, 2019). From some of these genes, hundreds of different proteins can be made leading to a substantial increase of the coding capacity of the genome. However, the functional consequences of diversity generated by alternative splicing remain poorly understood.
In honey bees, an invertebrate model species for the study of learning and memory processes, requirement of transcription and the neuronal RNA binding protein ELAV during memory consolidation further suggest important roles for alternative splicing (Lefer et al., 2012; Villar et al., 2020; Ustaoglu et al., 2021). Accordingly, alternative splicing events in a number of cell adhesion molecules and ion channels among other genes have been linked to neuronal excitability, learning and memory (Beffert et al., 2005; Demares et al., 2013; Demares et al., 2014; Poplawski et al., 2016; Sengar et al., 2019; Torres-Mendez et al., 2019; Ustaoglu et al., 2021; Torres-Méndez et al., 2022). Likewise, ELAV itself is substantially alternatively spliced in honey bee mushroom bodies (brain centers for learning and memory) and its expression pattern differs between individuals, likely reflecting differences in individual experiences (Ustaoglu et al., 2021).
The Down syndrome cell adhesion molecule (Dscam) gene encodes one of the largest immunoglobulin (Ig) domain superfamily cell adhesion molecule and contains 10 Ig domains and six fibronectin type III (FN) repeats. This overall protein structure of Dscam is evolutionary conserved between vertebrates and invertebrates with an overall amino acid identity and similarity of 29 and 46% between humans and Drosophila, respectively, (Crayton et al., 2006; Schmucker and Chen, 2009; Hu et al., 2011). In arthropods, Dscam is massively alternatively spliced to generate tens of thousands of different protein isoforms from four clusters of mutually exclusive variable exons that vary in numbers between different species (Graveley et al., 2004; Hemani and Soller, 2012; Zhang et al., 2019). In Drosophila, the Dscam gene contains 95 alternative exons in variable cluster 4 (12 exons), 6 (48 exons), 9 (33 exons), and 17 (2 exons) to generate 36’016 different mRNAs (Schmucker et al., 2000).
Drosophila Dscam is expressed in the nervous system where its variability is used during development for neuronal wiring, and in the immune system as a pattern recognition receptor for pathogen clearance by phagocytosis (Watson et al., 2005; Hemani and Soller, 2012). The main roles in the nervous system comprise establishment of overlapping dendritic fields in the peripheral nervous system and to branch neurites in neuronal tracts (Hughes et al., 2007; Matthews et al., 2007; Soba et al., 2007). Key to these functions of Dscam are homophilic repulsive properties of individual identical Dscam isoforms, but not to other splice variants (Wojtowicz et al., 2004; Wojtowicz et al., 2007). Accordingly, dendrites of neurons can overlap if they express different Dscam isoforms and neuronal tracts such as the Drosophila mushroom body containing hundreds of neurons can split into two lobes (Wang et al., 2002; Matthews et al., 2007). Between Drosophila and honey bees, Dscam protein is highly conserved indicated by 66% identity and 76% similarity, respectively, (Hu et al., 2011).
Mechanistically, it has been proposed in Drosophila that individual neurons adopt a unique set of variables stochastically to comply with their fate in branching in the peripheral nervous system and in the mushroom bodies (Neves et al., 2004; Miura et al., 2013). This model further implies that Dscam alternative splicing is maintained in individual neurons. In the immune system of mosquitoes, however, exposure to different pathogens altered inclusion of variables to generate variants with higher affinity (Dong et al., 2006). Accordingly, both stochastic as well as deterministic rules have been found to govern dendritic branching in Drosophila (Palavalli et al., 2021). Moreover, the homophilic interaction of identical Dscam isoforms in Drosophila could also be converted to attraction at low levels and in this way build novel connections (Wojtowicz et al., 2004).
Here, we examined its role in learning and memory in honey bees, where memory consolidation involves changes in gene expression (Lefer et al., 2012; Villar et al., 2020; Ustaoglu et al., 2021) and neuronal connectivity (Hourcade et al., 2010). Here, we discovered that down-regulated Dscam during learning enhances memory consolidation in honey bees. To characterize alternative splicing in honey bees we sequenced Dscam cDNAs encompassing the individual variable clusters to comprehensively update previous annotation from comparing genomic sequences (Graveley et al., 2004). This analysis reveals that honey bees have 9 exon 4, 52 exon 6, and 19 exon 10 variables. Unlike Drosophila, we find intra-cluster splicing in honey bee Dscam. When we analysed alternative splicing during the course of memory consolidation, we discovered that alternative splicing significantly changed within variable clusters. Our results indicate that changes in Dscam splicing can be induced upon experience likely to establish new neuronal connections associated with memory consolidation.
Results
Dscam is required for memory consolidation in honey bees after olfactory reward conditioning
To detect honey bee Dscam, we used a polyclonal anti-serum raised against Drosophila Dscam (Watson et al., 2005). In honey bees this polyclonal antibody recognizes proteins at the size of Drosophila Dscam of 222 and 270 kDa and three shorter bands of 100, 110, and 130 kDa (Supplementary Figure 1A). To validate that the larger band recognized by the antibody is indeed honey bee Dscam, we injected double stranded RNA (dsRNA) against honey bee Dscam into the central brain for knock-down by RNAi. A substantial reduction of 72 ± 5% and 79 ± 10% (n = 3 each) is achieved after 48 and 64 h of the expected bands at 222 and 270 kDa, respectively, but not of the shorter bands of 100, 110, and 130 kDa suggesting that these bands in honey bees are unspecific (Figure 1A and Supplementary Figure 1B).
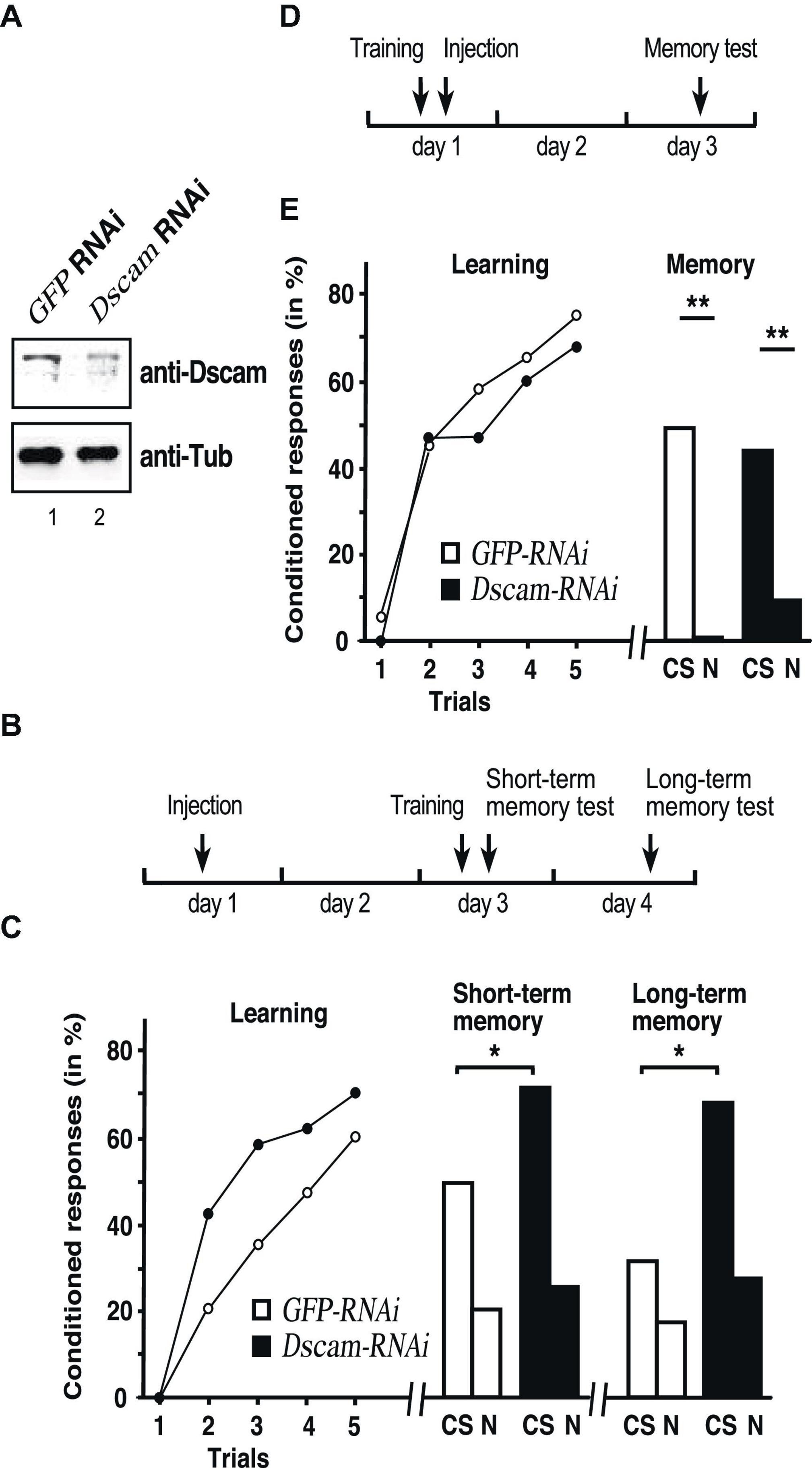
Figure 1. Down syndrome cell adhesion molecule (Dscam) is required for learning and memory consolidation. (A) Western blot detecting Dscam (top) or tubulin (bottom) in honey bee central brains of control GFP and Dscam RNAi 48 h after injection of dsRNA into worker honey bees. (B) Schematic of the treatment to test for Dscam’s role in learning and memory consolidation. (C) Learning (left), short term (middle) and long-term memory (right) performances of control GFP RNAi (white) and Dscam RNAi (black) after injection of worker honey bees with dsRNA. CS: conditioned odor, N: novel odorant. *p < 0.05. (D) Schematic of the treatment control for Dscam’s role in learning and memory consolidation. (E) Learning (left) and memory (right) performances of control GFP RNAi (white, n = 39/n = 22 at 24 h) and Dscam RNAi (black, n = 46/n = 15 at 24 h) after injection of worker honey bees with dsRNA. CS, conditioned stimulus, N, novel odorant **p < 0.01. The source date underlying this figure are available in Supplementary Data 1.
To assess whether Dscam has a role in learning and memory, honey bees were individually trained in an associative olfactory conditioning task 2 days after injection of Dscam dsRNA or GFP (control) dsRNA to then measure short-term and long-term memory 1 h and 1 day after training, respectively, (Figure 1B). Both groups showed significant learning over the successive trials (RM-ANOVA, Trial effect: F = 46.55, p < 0.001), without significant effect of the treatment (F = 2.28, p = 0.135, Figure 1C left). We then tested short-term (1 h following conditioning) and long-term (24 h) memory to ask whether Dscam downregulation could impact on memory formation despite preserved learning capacities (Figure 1C). Here, at 1 h (Figure 1C middle) honey bees from the Dscam group responded significantly more to the conditioned stimulus (CS) than those from the GFP group (Fischer’s exact test: χ2 = 4.72, p = 0.044), while the proportions of specific responses did not differ either (χ2 = 3.37, p = 0.118, Figure 1C middle and left). Yet, while both groups clearly responded more to the CS than to the novel odorant 1 h after training, the difference was not significant in either case (McNemar test, GFP: χ2 = 2.88, p = 0.090: Dscam: χ2 = 0.0588, p = 0.808). The proportions of individuals displaying specific responses (i.e., responses to the CS but not the novel odour, N) did not differ either between the groups (χ2 = 2.56, p = 0.125, Figure 1C middle). Thus, down-regulated Dscam levels enhanced memory performance without affecting its specificity.
At 24 h after conditioning, only a portion of honey bees survived and could be tested (GFP: n = 22, Dscam: n = 15). Both groups responded more to the CS than to the novel odorant, although the difference was not significant in the Dscam group due to a smaller sample size (McNemar test, GFP: χ2 = 4.84, p = 0.028; Dscam: χ2 = 0.048, p = 0.827, Figure 1C right). Again, the proportions of specific responses did not differ between the groups (χ2 = 0.3.37, p = 0.118), but honey bees from the Dscam group showed a marginally significantly higher response rate to the CS than those form the GFP group (Fischer’s exact test: χ2 = 4.36, p = 0.050). Thus, down-regulated Dscam levels enhanced CS-N specific long-term memory performance.
To reject the possibility that loss of Dscam impacts on long-term memory retrieval per se due to a prolonged downregulation of Dscam, we performed an additional experiment in which injection was done shortly after training, so that RNAi would be effective at the time of retrieval (24 h) rather than at consolidation (Figure 1D). As expected, this treatment did not affect learning (Trial effect: F = 48.48, p < 0.001; Trial x Treatment interaction: F = 0.697, p > 0.05; Figure 1E left). More importantly, long-term memory retrieval was intact and 2 days after training both groups responded similarly to the CS (Fischer’s test: χ2 = 0.729, p > 0.05) and responded significantly less to the novel odorant (GFP: χ2 = 5.23, p < 0.05; elavl2: χ2 = 7.47, p < 0.01), thus indicating a preserved memory of the CS-US association and no enhancement of memory (Figure 1E right).
These results thus argue that down-regulation of Dscam during memory consolidation enhances storage of memories.
Alternative splicing analysis of honey bee Dscam reveals novel isoforms and intra-cluster splicing
Since Dscam in arthropods is abundantly alternatively spliced, we wanted to analyze Dscam alternative splicing after training, but this required evaluation of the gene annotation. The exon-intron structure of honey bee Dscam had previously been annotated based on phylogenomic conservation and comparison to cDNAs obtained from Drosophila Dscam (Graveley et al., 2004). To directly address alternative splicing in Dscam variable clusters 4, 6, and 10 in honey bees, we RT-PCR amplified the variable clusters and Illumina sequenced the amplicons. This analysis revealed strong overlap with the previous annotation, but also novel isoforms and additional intra-cluster splicing (Decio et al., 2019).
In particular, in each of the clusters we find an additional exon (exon 4.0, 6.0, and 10.0) before the previously annotated isoforms (Figures 2A–C). Exon 4.0 and 10.0 alter the frame resulting in non-productive isoforms.
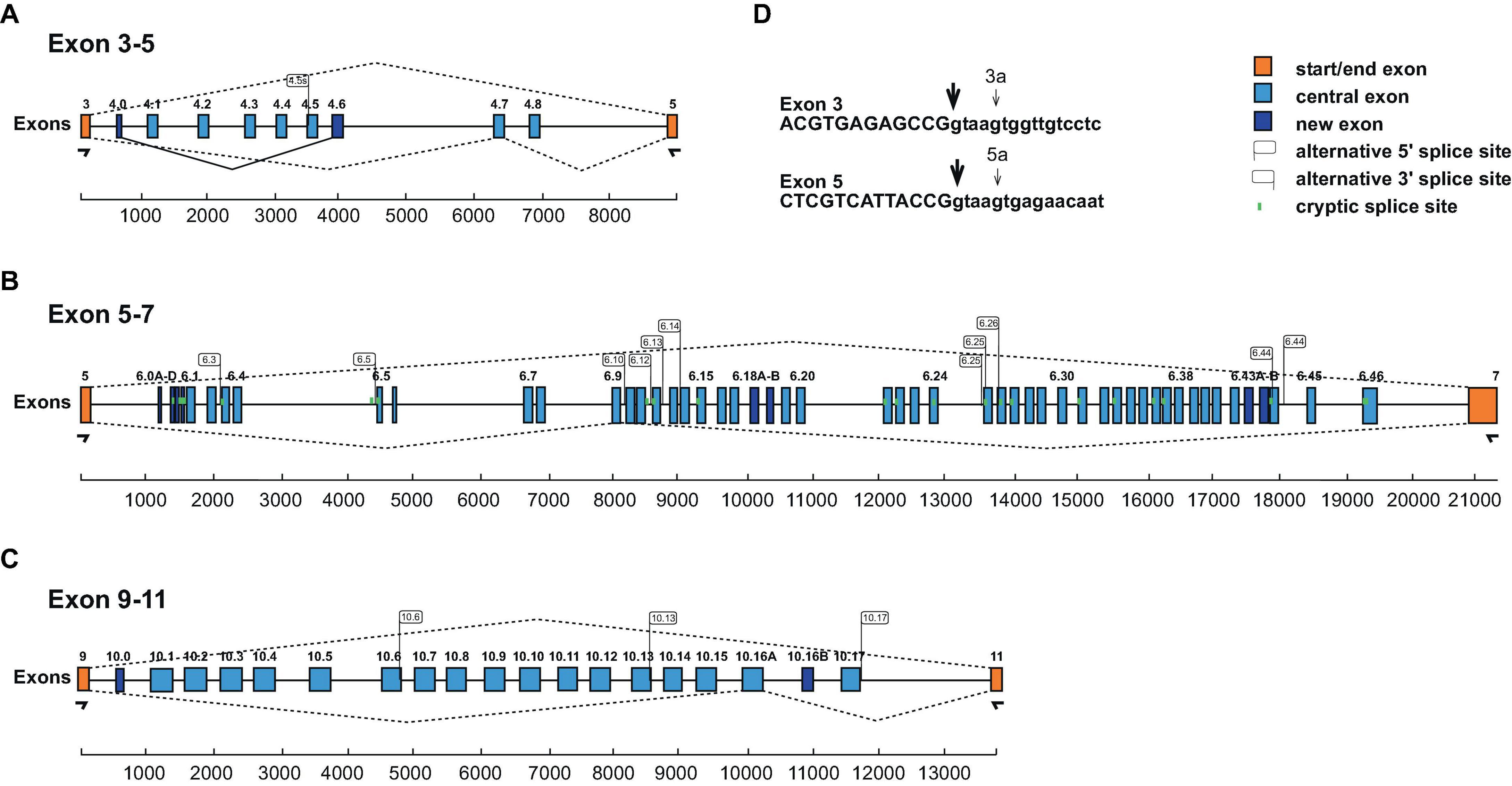
Figure 2. Schematic of the honey bee Dscam variable exon clusters 4, 6, and 10. (A–C) Gene models of Apis mellifera Dscam variable exon clusters 4 (A, top), 6 (B, middle), and 10 (C, bottom) depicting constant flanking exons in orange and variable exons in light blue boxes. New variable exons are indicated by dark blue boxes. Alternative 5’ and 3’ splice sites are indicated by right and left directed flags, respectively. Intra-cluster splicing is indicated by solid lines and dashed lines indicate representative alternative splicing. Cryptic splice sites (<1%) are indicated by vertical green lines. Exon clusters are drawn to scale with scale bars are shown at the bottom. (D) Alternative 5’ splice sites in exons 3 and 5 are shown as small arrows.
In the exon 4 cluster, we find prominent use of an alternative 5’ splice sites in exon 3 that splices to exons 4.1 and 4.3 (3a4.1 and 3a4.3, Figure 2D) that changes the open reading frame to generate non-function products. In addition, an addition 3’ splice site is present in exon 4.5 generating an in-frame shorter isoform (Figure 2A).
In the beginning of the exon 6 cluster, we find a number of 3’ splice sites, which we describe as exons 6.0A-D, but we could not detect a downstream 5’ splice site by amplicon sequencing (Figure 2B). Potentially, they could include large exons using known 5’ splice sites.
In the exon 6 and 10 clusters (Figures 2B, C), we identified additional variable exons (6.18B, 6.43B, and 10.16B), that maintain the reading frame and additional 5’ and 3’ splice sites, that are above splicing noise (>1%). In the exon 6 cluster, we find one additional 5’ splice site (6.44s2) and 13 new 3’ splice sites (exons 6.1s, 6.3s, 6.5s, 6.10s, 6.11s, 6.12s, 6.13s, 6.14s, 6.15s, 6.25s1, 6.25s2, 6.26s, 6.31s, 6.33s, and 6.44s). In the exon 10 cluster, we find three additional 5’ splice sites (exon 10.6s, 10.13s, and 10.17s). All of these new variable exons maintain the reading frame.
In addition, we find low levels of skipping of exons in the variable clusters. Skipping of exon 4 and 10 variables maintains the reading frame, while skipping of exon 6 variables leads to truncated Dscam isoforms.
In summary, our expression analysis of honey bee Dscam mutually exclusive splicing reveals 9 exon 4 variables, 52 exon 6 variables, and 19 exon 10 variables.
Alternative splicing in Dscam exon 4 cluster changes in slow learners during memory consolidation
To analyse alternative splicing of Dscam in the variable cluster 4, we used our previously established gel-based assay, that can distinguish all eight variables and exon skipping in addition to newly discovered isoforms and reliably detect changes during development and in adult morphs (Decio et al., 2019; Haussmann et al., 2019). When we analysed inclusion of exon 4 variables we noticed obvious differences in some honey bees, particularly ones that have learned faster than others (Ustaoglu et al., 2021) and we therefore split trained honey bees into two groups of fast and slow learners. As negative controls, we used a group of unpaired honey bees, which were presented the stimuli (CS: odorant, US: sucrose) as trained honey bees, except that the delay between the CS and US was increased to impede the learning of any association.
Upon learning differences appeared immediately particularly for exons 4.1 and 4.3s as well as exon skipping, but only in some honey bees (Figure 3A). When we quantified the inclusion levels (Figures 3B–D), significant changes were observed in exons 4.0 and 4.6s 2 h after training in the slow learners. A total of 4 h after training, significant changes were detected for exons 4.2, 4.3, 4.6s and skipped exons in slow learners (Figure 3D).
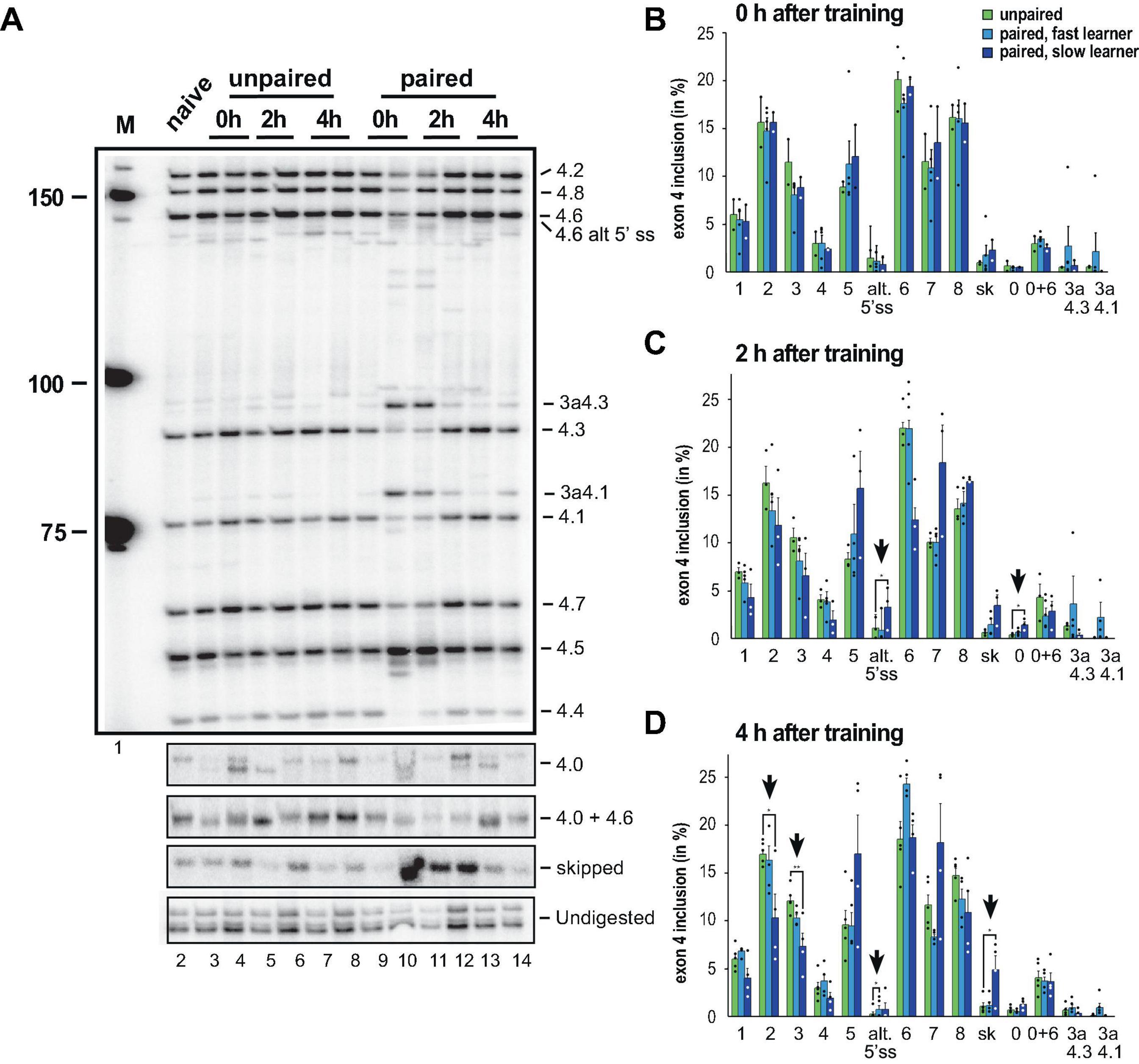
Figure 3. Down syndrome cell adhesion molecule (Dscam) variable exon 4 alternative splicing analysis during memory consolidation. (A) Representative 5% denaturing polyacrylamide gel separating 32P-labeled alternative splice products from worker brains conditioned with unpaired (left) and paired odors and reward. Identity of splice variants are indicated at the right. M, Marker. (B–D) Analysis of alternative splicing unpaired control (green), fast (light blue) and slow learners (dark blue) indicating exon inclusion frequency (in%) for the different variables immediately after training (0 h), 2 or 4 h after training. Significant changes are indicated by vertical arrows (*p < 0.05; **p < 0.01). The source date underlying this figure are available in Supplementary Data 1.
Alternative splicing in Dscam exon 10 variables changes most prominently in slow learners 2 h after training
To analyse alternative splicing of Dscam in the variable cluster 10, we also used our previously established gel-based assay, that can distinguish 13 from 18 variables (72%) and exon skipping with significant changes during development and in adult morphs (Figure 4A and Supplementary Figure 2).
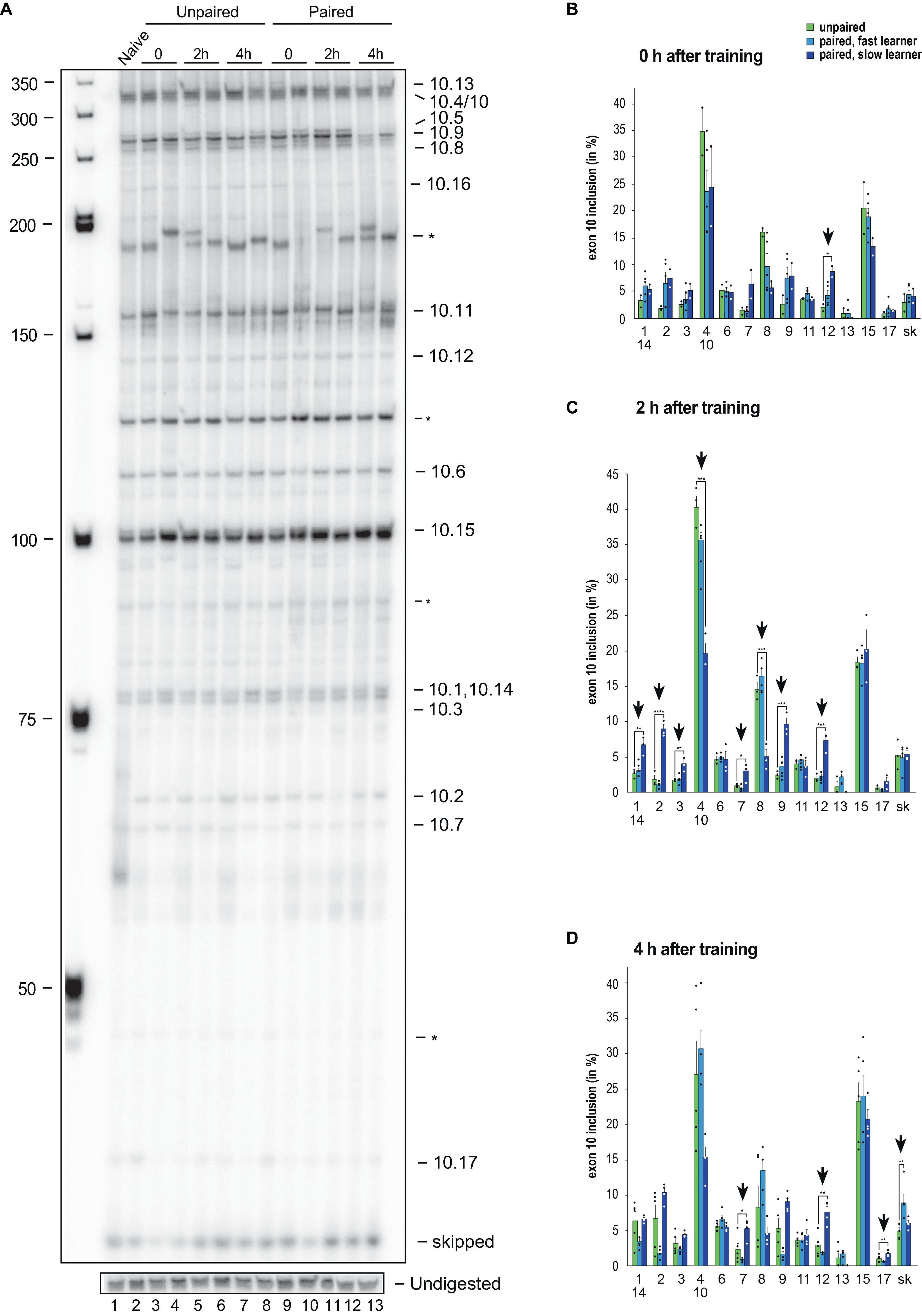
Figure 4. Down syndrome cell adhesion molecule (Dscam) variable exon 10 alternative splicing analysis during memory consolidation. (A) Representative 5% denaturing polyacrylamide gel separating 32P-labeled alternative splice products from worker brains conditioned with unpaired (left) and paired odors and reward. Identity of splice variants are indicated at the right. M, Marker. (B–D) Analysis of alternative splicing unpaired control (green), fast (light blue) and slow learners (dark blue) indicating exon inclusion frequency (in%) for the different variables immediately after training (0 h), 2 or 4 h after training. Significant changes are indicated by vertical arrows (*p < 0.05; **p < 0.01; ***p < 0.001; ****p < 0.0001). The source date underlying this figure are available in Supplementary Data 1.
Upon olfactory conditioning of honey bees, significant changes were detected immediately after training in slow learners for exon 10.12 (Figure 4B). Intriguingly, 2 h after training significant changes were detected in 62% (8 from 13) of variable exons in slow learners (Figure 4C). A total of 4 h after training, significant changes were found in exons 10.7, 10.12, and 10.17 of slow learners, while fast learners show a significant increase in exon skipping (Figure 4D).
Alternative splicing in Dscam of more than half of exon 6 variables changes in fast learners 2 h after training
For the analysis of Dscam alternative splicing in the exon 6 cluster, we switched to amplicon sequencing as the gel-based assay could not sufficiently resolve the variables. For this analysis we did paired-end sequencing 2 h after training and summed up the reads for control honey bees where no paired stimulus was given, and for honey bees that either learned fast or slow.
Strikingly, from the 48 different exon 6 variables, 63% (30 out of 48), showed significant changes in fast learning honey bees compared to controls, while no significant differences were detected between controls and slow learners (Figures 5A, B).
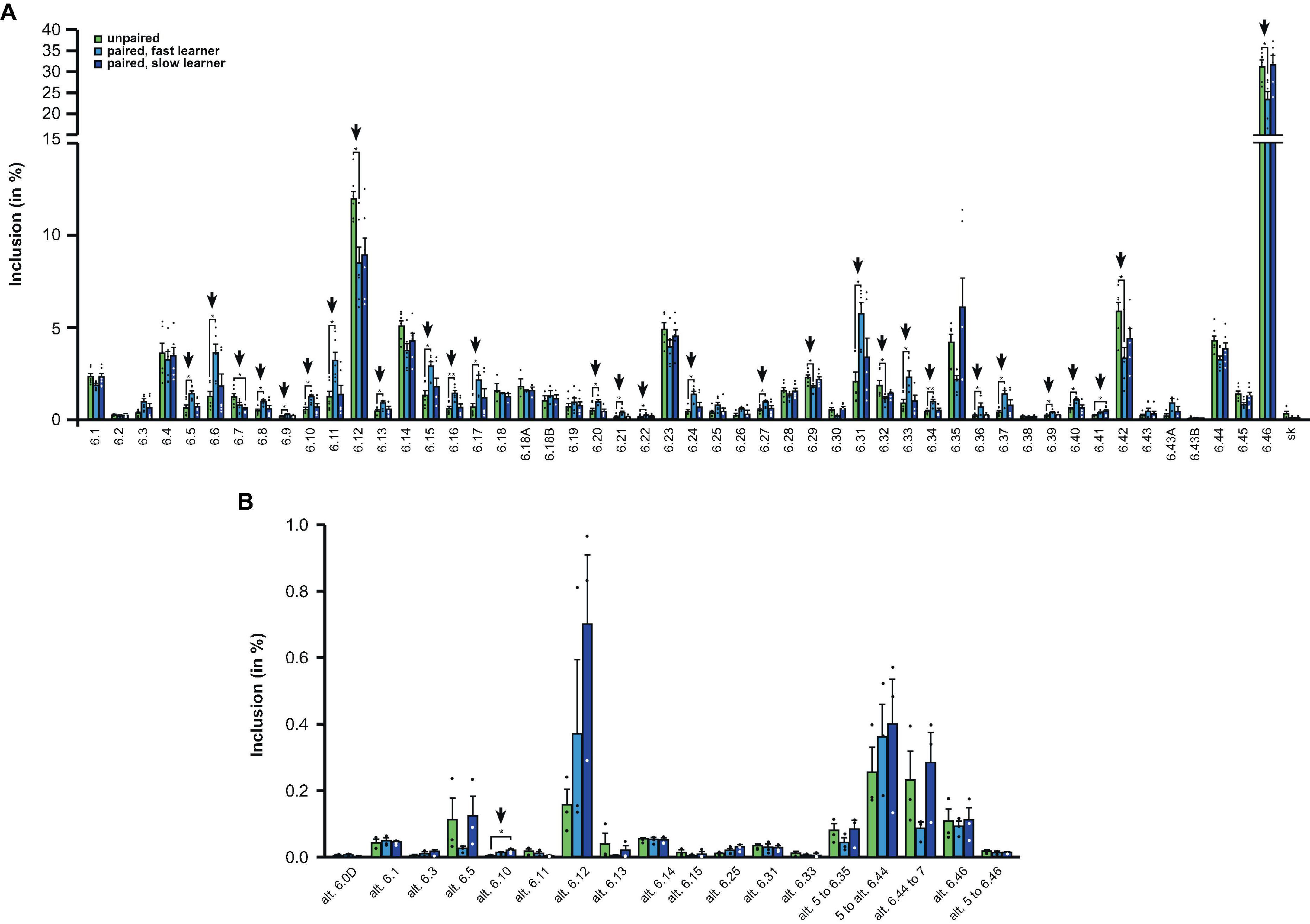
Figure 5. Down syndrome cell adhesion molecule (Dscam) variable exon 6 alternative splicing analysis during memory consolidation. (A,B) Analysis of alternative splicing unpaired control (green), fast (light blue) and slow learners (dark blue) indicating exon inclusion frequency (in%) from amplicon sequencing for the different variables 2 h after training for main variable (A) and alternative splice sites (B). Significant changes are indicated by vertical arrows (*p < 0.05; **p < 0.01). The source date underlying this figure are available in Supplementary Data 1.
Discussion
Alternative splicing massively expands proteomic diversity and is particularly abundant in the brain. It is most prominently elaborated in the arthropod Dscam gene making about three times as many proteins than genes present in the genome (Soller, 2006; Hemani and Soller, 2012). Here, we characterize in depth the alternative splicing landscape of the honey bee Dscam gene and identify novel isoforms including an increased use of alternative 5’ and 3’ splice sites and intra-cluster splicing further expanding the diversity and shedding light on its unusual splicing regulation. Dscam mis-regulation has been associated with intellectual disabilities in humans associated with an additional copy of chromosome 21 (Vacca et al., 2019). In particular, an extra copy of Dscam in Drosophila causes strengthening of synapses and in a mouse model leads to excessive GABAergic synapses in the neocortex (Lowe et al., 2018; Liu et al., 2023). When we analysed learning and memory in honey bees upon reducing Dscam levels by RNAi we find enhanced memory storage. In addition, we find that alternative splicing of Dscam changes in the variable clusters during memory consolidation. Since Dscam homophilic interaction could stabilize existing connections (Chen et al., 2006), down-regulation of specific isoforms could loosen existing neuronal connections to stimulate formation of new ones, essentially recapitulating down-regulation of Dscam as obtained by RNA interference. Hence, an evolutionary conserved function of Dscam in learning and memory likely lies in changing expression, that in Drosophila can also be achieved by alterations in alternative splicing.
To store individual memory traces in the mushroom bodies, likely only few neurons are involved. It is thus surprising that Dscam alternative splicing changed substantially, such that significant changes could be detected in central brains. It will be of interest to follow up which neurons exactly change splicing upon learning, which might be possible with the development of long-read sequencing technology for single cell analysis to overcome current limitations in alternative splicing analysis (Zaharieva et al., 2012; Decio et al., 2019; Decio et al., 2021).
Memory consolidation requires transcription and implicates that alternative splicing can impact on the process of memory storage (Kandel et al., 2014; Ustaoglu et al., 2021). In honey bees, a first transcriptional wave occurs at the beginning of memory consolidation (Lefer et al., 2012). Of note, it is during this phase that also Dscam alternative splicing changes. And importantly, honey bees have displayed strikingly different splicing patterns depending on whether they actually learn or not during training. The most prominent changes occur in the exon 6 cluster, which compared to Drosophila has expanded by additional isoforms but also more frequent use alternative 5’ and 3’ splice sites, which is constituent with the more sophisticated neuroanatomy and behavioral performance of honey bees compared to Drosophila. In contrast, exon 4 and 10 clusters in honey bees have less isoforms. Lower diversity in these two clusters has been suggested to be associated with Dscam’s role as a pathogen receptor in the immune system as flies are extensively exposed to pathogens due to their life in a decomposing environment (Graveley et al., 2004).
Dscam diversity has been shown to change in mosquitos toward isoforms that recognize pathogens with higher affinity (Dong et al., 2006). Our finding that Dscam splicing changes upon learning further supports that the Dscam splicing pattern is not fixed and can change on demand. In fact, levels of Dscam have been found critical to neuronal function in Drosophila affecting nerve growth, synaptic targeting and neuronal physiology (Cvetkovska et al., 2013; Lowe et al., 2018; Hernández et al., 2023). Moreover, Dscam has also been identified as a target of Fragile X messenger ribonucleoprotein 1 (Fmr1) that together with mRNA modification pathways impacts on local translation important for neuronal functions such as learning and memory (Cvetkovska et al., 2013; Haussmann et al., 2022; Anreiter et al., 2023).
Consistent with subtle changes in Dscam expression being effective in altering nerve growth, synaptic targeting and neuronal physiology, we find changes in alternative splicing during memory consolidation. In particular, skipping of the entire variable cluster occurs for exon 4 and 10 shortly after training resulting in removal of part of the homophilic interaction domain concomitantly reducing cell adhesion (Wojtowicz et al., 2004).
Dynamic regulation of Dscam expression in the nervous system is somewhat unexpected based on Dscam’s role in mediating axonal bifurcation for exit from axonal tracts or spreading in dendritic fields based on Dscam’s homophilic repulsive properties. Potentially, Dscam’s repulsive homophillic properties could only apply during development of the nervous system and convert to attraction to expand connectivity in mushroom bodies to consolidate memories. In fact, attractive properties of Dscam have been found with lower Dscam concentration (Wojtowicz et al., 2004). Moreover, Dscam has been shown roles in directed wiring of peripheral nervous system (PNS) neurons to innervate the ventral nerve cord (Chen et al., 2006). Likewise, whether homophilic binding of Dscam turns into repulsive or attractive cues is also dependent on interacting partners such as Netrins, Robo and Slit (Andrews et al., 2008; Liu et al., 2009; Alavi et al., 2016).
How Dscam mutually exclusive alternative splicing is regulated is still poorly understood. Initially, it has been proposed that base-pairing of a constant region at the beginning or end of the cluster with sequences in front of variable exons would direct inclusion of variable exons, but compelling sequence conservation is only present in the exon 6 cluster (Graveley, 2005; Haussmann et al., 2019; Ustaoglu et al., 2019). Our finding that inclusion of alternative exons from the variable clusters changes upon memory consolidation further suggests that there is a mechanism to alter inclusion of specific variables possibly to change neuronal connections for enhancing memory. Given an increasingly aging human population, deciphering the molecular mechanism underlying memory enhancement is of great interest in light of cognitive disfunctions associated with Alzheimer’s, Parkinson and other age-related diseases (Stern and Alberini, 2013; Noyes et al., 2021).
Materials and methods
Honey bees, treatment, and behavioral assays
For initial molecular analysis of honey bee Dscam, honey bees (Apis mellifera) were collected from local honey bee hives in the UK (worker honey bees were used unless otherwise specified). For developmental gene expression analysis and behavioral experiments, honey bees were taken from the experimental apiary on the university campus in Toulouse (France), on the morning of each experiment. For behavioral experiments, workers were harnessed in metal tubes following cold-anesthesia leaving access to the head, fed with 5 μl of sucrose solution (50% weight/weight in water) and until needed kept in the dark at room temperature. They were fed in the same way on every morning and evening during the time of each experiment. Learning and memory experiments were done as described (Ustaoglu et al., 2021).
RNAi, Western analysis recombinant protein expression antibody stainings and imaging
For RNAi knockdown in honey bees, a Dscam DNA template of 820 bp was amplified spanning a cDNA of the most conserved part of Dscam (exons 11−14) with primers AM Dscam T7 RNAi 11F and AM Dscam T7 RNAi 14R containing a T7 promoter on each side and cloned into into pB SK + tango with Xho and EcoRV according to standard procedures (Soller and White, 2005). A 700 bp fragment for GFP was amplified as described (Ustaoglu et al., 2021). dsRNA was generated by in vitro transcription with T7 polymerase with the MegaScript kit (Ambion) for 3 h according to the manufacturer’s instructions. After digestion of the template with TurboDNAse (Ambion), dsRNA was phenol/chloroform extracted, ethanol precipitated and taken up in RNAse free water at a concentration of 5 μg/μl. The dsRNA (250 nl) was then injected into the brain through the median ocellus with a Nanoject II microinjector (Drummond).
RNAi efficiency testing for Dscam was done from dissected central brains by Western blotting according to standard protocols as described (Soller and White, 2005) using a polyclonal rat anti-Dscam antibody (1:1000, 358 against a constant part from amino acid 1650 to 2016) (Watson et al., 2005) and infrared dye coupled secondary antibodies (IRDye800CW, LI-COR) were used and detected with an Odyssey infrared imaging system (LI-COR). Tubulin was detected with a mouse anti-alpha tubulin antibody (1:10,000, clone DM1A, SIGMA). Quantification of Western blots was done with Quantity ONE 4.6.8 (BioRad) according to the manufacturer’s instructions.
RT-PCR and analysis of alternative splicing
The sequence of oligonucleotides used in this study are listed in Supplementary Table 1. RNA extraction from eggs or dissected central brains and RT-PCR was done as described (Koushika et al., 1999). For RT, AM GSP exon 13 RT1 was used at concentration of 10 nM spiked into oligo dT (10 μM) (Haussmann et al., 2011).
For high resolution analysis of Dscam alternative splicing primers AM Dscam 3F1MH and AM Dscam 5R1 were used to amplify exon 4 variables, and AM Dscam 9F2 and AM Dscam 11R2 for exon 10 variables from cDNA by PCR with Taq according to the manufacturer’s instructions for 40 cycles as described (Soller and White, 2005). One of the primers was labeled using gamma32P-ATP (NEN). PCR products for exon 4 variables were digested with a combination of restriction enzymes Sau3AI, HaeIII, and MboI, and PCR products for exon 10 variables with a combination of restriction enzymes RsaI, PvuII, and BsaJI. Then, PCR products were separated on 5% sequencing type denaturing polyacrylamide gels. Polyacrylamide gels were dried, exposed to phosphoimager screens (BioRad) and quantified with QuantityOne (BioRad).
Amplicon sequencing and sequence analysis
For amplicon sequencing, primers in constant exons flanking the variable parts were used for amplification from cDNA generated from dissected central brains as described above. For amplification of variable exon 6 from three groups of three individual honey bees AM Dscam 5F1A, B and C were used for the different groups and AM Dscam 7R1A, B and C were used for the three individual honey bees. Amplicons were then quantified on ethidium bromide stained agarose gels against a DNA marker (100 bp ladder, NEB), pooled and then Illumina sequenced (paired end, read-length 126) by GATC (Eurofins, Germany). Sequences were demultiplexed according to barcodes for exon 6 variables or by sequence cluster identity for exon 4 and 10 variables and barcodes removed using an in-house script. The demultiplexed data was mapped to the Honey Bee reference genome (version Amel_HAv3.1) using STAR (STAR version 272B, parameters: –alignIntronMin 20 –alignIntronMax 200000 –alignMatesGapMax 200000 –twopassMode Basic). Junction read coverage matching Dscam (AY686596) (Graveley et al., 2004) have been used to compute inclusion levels of splicing events, following which junction reads were manually checked.
Statistics and reproducibility
Multiple planned pairwise comparisons of expression levels were done by ANOVA followed by Fisher’s protected least significance difference post-hoc test using Prism. To compare proportions of conditioned responses between groups, a repeated-measure analysis of variance (ANOVA) was run for the acquisition data (one factor, treatment, with trial as the repeated measure) (Mota and Giurfa, 2010). Comparisons of responses at each memory test were run using McNemar and Fischer’s exact tests, for intra- and inter-group comparisons, respectively.
Data availability statement
All data are available in the main text or the Supplementary Data 1. Reagents are available upon reasonable request from the corresponding author. Amplicon-Seq data have been deposited at GEO (GSE244051).
Ethics statement
Ethical review and approval was not required for this study because this study was conducted with an invertebrate model – honey bee (Apis mellifera). Experiments with invertebrates are not regulated by law.
Author contributions
PU: Formal analysis, Investigation, Writing – review & editing. DM: Data curation, Formal analysis, Investigation, Writing – review & editing. AR: Formal analysis, Investigation, Writing – review & editing. TD: Formal analysis, Investigation, Writing – review & editing. IH: Conceptualization, Formal analysis, Investigation, Writing – review & editing. RA: Data curation, Formal analysis, Supervision, Writing – review & editing. J-MD: Conceptualization, Formal analysis, Investigation, Validation, Writing – review & editing. MS: Conceptualization, Funding acquisition, Investigation, Supervision, Validation, Writing – original draft, Writing – review & editing.
Funding
The author(s) declare financial support was received for the research, authorship, and/or publication of this article. For this work we acknowledge funding from the Sukran Sinan Fund, the Genetics Society, the Biochemical Society, MRC DTP, and BBSRC (BB/T003936/1). J-MD acknowledges funding from the CNRS and Université Paul Sabatier.
Acknowledgments
We thank the Winterbourne garden (Birmingham), Jane and Lucie Hotier (Toulouse) for providing honey bees, Dietmar Schmucker for a rabbit anti-Dscam antibodies, Anna Lassota for help with stainings and imaging, Reinhard Stöger for discussions and comments on the manuscript.
Conflict of interest
The authors declare that the research was conducted in the absence of any commercial or financial relationships that could be construed as a potential conflict of interest.
Publisher’s note
All claims expressed in this article are solely those of the authors and do not necessarily represent those of their affiliated organizations, or those of the publisher, the editors and the reviewers. Any product that may be evaluated in this article, or claim that may be made by its manufacturer, is not guaranteed or endorsed by the publisher.
Supplementary material
The Supplementary Material for this article can be found online at: https://www.frontiersin.org/articles/10.3389/fnmol.2023.1322808/full#supplementary-material
References
Alavi, M., Song, M., King, G. L., Gillis, T., Propst, R., Lamanuzzi, M., et al. (2016). Dscam1 forms a complex with robo1 and the N-terminal fragment of slit to promote the growth of longitudinal axons. PLoS Biol. 14:e1002560. doi: 10.1371/journal.pbio.1002560
Alberini, C. M. (2009). Transcription factors in long-term memory and synaptic plasticity. Physiol. Rev. 89, 121–145.
Alberini, C. M., Ghirardi, M., Metz, R., and Kandel, E. R. (1994). C/EBP is an immediate-early gene required for the consolidation of long-term facilitation in Aplysia. Cell 76, 1099–1114.
Andrews, G. L., Tanglao, S., Farmer, W. T., Morin, S., Brotman, S., Berberoglu, M. A., et al. (2008). Dscam guides embryonic axons by Netrin-dependent and -independent functions. Development 135, 3839–3848. doi: 10.1242/dev.023739
Anreiter, I., Tian, Y. W., and Soller, M. (2023). The cap epitranscriptome: Early directions to a complex life as mRNA. Bioessays 45:e2200198. doi: 10.1002/bies.202200198
Beffert, U., Weeber, E. J., Durudas, A., Qiu, S., Masiulis, I., Sweatt, J. D., et al. (2005). Modulation of synaptic plasticity and memory by Reelin involves differential splicing of the lipoprotein receptor Apoer2. Neuron 47, 567–579. doi: 10.1016/j.neuron.2005.07.007
Chen, B. E., Kondo, M., Garnier, A., Watson, F. L., Puettmann-Holgado, R., Lamar, D. R., et al. (2006). The molecular diversity of Dscam is functionally required for neuronal wiring specificity in Drosophila. Cell 125, 607–620.
Crayton, M. E. III, Powell, B. C., Vision, T. J., and Giddings, M. C. (2006). Tracking the evolution of alternatively spliced exons within the Dscam family. BMC Evol. Biol. 6:16. doi: 10.1186/1471-2148-6-16
Cvetkovska, V., Hibbert, A. D., Emran, F., and Chen, B. E. (2013). Overexpression of Down syndrome cell adhesion molecule impairs precise synaptic targeting. Nat. Neurosci. 16, 677–682. doi: 10.1038/nn.3396
Decio, P., Ustaoglu, P., Derecka, K., Hardy, I. C. W., Roat, T. C., Malaspina, O., et al. (2021). Thiamethoxam exposure deregulates short ORF gene expression in the honey bee and compromises immune response to bacteria. Sci. Rep. 11:1489. doi: 10.1038/s41598-020-80620-7
Decio, P., Ustaoglu, P., Roat, T. C., Malaspina, O., Devaud, J. M., Stoger, R., et al. (2019). Acute thiamethoxam toxicity in honeybees is not enhanced by common fungicide and herbicide and lacks stress-induced changes in mRNA splicing. Sci. Rep. 9:19196.
Demares, F., Drouard, F., Massou, I., Crattelet, C., Loeuillet, A., Bettiol, C., et al. (2014). Differential involvement of glutamate-gated chloride channel splice variants in the olfactory memory processes of the honeybee Apis mellifera. Pharmacol. Biochem. Behav. 124, 137–144. doi: 10.1016/j.pbb.2014.05.025
Demares, F., Raymond, V., and Armengaud, C. (2013). Expression and localization of glutamate-gated chloride channel variants in honeybee brain (Apis mellifera). Insect Biochem. Mol. Biol. 43, 115–124.
Dong, Y., Taylor, H. E., and Dimopoulos, G. (2006). AgDscam, a hypervariable immunoglobulin domain-containing receptor of the Anopheles gambiae innate immune system. PLoS Biol. 4:e229. doi: 10.1371/journal.pbio.0040229
Graveley, B. R. (2005). Mutually exclusive splicing of the insect Dscam pre-mRNA directed by competing intronic RNA secondary structures. Cell 123, 65–73. doi: 10.1016/j.cell.2005.07.028
Graveley, B. R., Kaur, A., Gunning, D., Zipursky, S. L., Rowen, L., and Clemens, J. C. (2004). The organization and evolution of the dipteran and hymenopteran Down syndrome cell adhesion molecule (Dscam) genes. RNA 10, 1499–1506. doi: 10.1261/rna.7105504
Haussmann, I. U., Li, M., and Soller, M. (2011). ELAV-mediated 3’-end processing of ewg transcripts is evolutionarily conserved despite sequence degeneration of the ELAV-binding site. Genetics 189, 97–107. doi: 10.1534/genetics.111.131383
Haussmann, I. U., Ustaoglu, P., Brauer, U., Hemani, Y., Dix, T. C., and Soller, M. (2019). Plasmid-based gap-repair recombineered transgenes reveal a central role for introns in mutually exclusive alternative splicing in Down Syndrome Cell Adhesion Molecule exon 4. Nucleic Acids Res. 47, 1389–1403. doi: 10.1093/nar/gky1254
Haussmann, I. U., Wu, Y., Nallasivan, M. P., Archer, N., Bodi, Z., Hebenstreit, D., et al. (2022). CMTr cap-adjacent 2‘-O-ribose methyltransferases are required for reward learning and mRNA localization to synapses. Nat. Commun. 31:1209. doi: 10.1038/s41467-022-28549-5
Hemani, Y., and Soller, M. (2012). Mechanisms of Drosophila Dscam mutually exclusive splicing regulation. Biochem. Soc. Trans. 40, 804–809.
Hernández, K., Godoy, L., Newquist, G., Kellermeyer, R., Alavi, M., Mathew, D., et al. (2023). Dscam1 overexpression impairs the function of the gut nervous system in Drosophila. Dev. Dyn. 252, 156–171. doi: 10.1002/dvdy.554
Hourcade, B., Muenz, T. S., Sandoz, J. C., Rossler, W., and Devaud, J. M. (2010). Long-term memory leads to synaptic reorganization in the mushroom bodies: A memory trace in the insect brain? J. Neurosci. 30, 6461–6465. doi: 10.1523/JNEUROSCI.0841-10.2010
Hu, Y., Flockhart, I., Vinayagam, A., Bergwitz, C., Berger, B., Perrimon, N., et al. (2011). An integrative approach to ortholog prediction for disease-focused and other functional studies. BMC Bioinformatics 12:357. doi: 10.1186/1471-2105-12-357
Hughes, M. E., Bortnick, R., Tsubouchi, A., Baumer, P., Kondo, M., Uemura, T., et al. (2007). Homophilic Dscam interactions control complex dendrite morphogenesis. Neuron 54, 417–427. doi: 10.1016/j.neuron.2007.04.013
Kandel, E. R., Dudai, Y., and Mayford, M. R. (2014). The molecular and systems biology of memory. Cell 157, 163–186.
Koushika, S. P., Soller, M., Desimone, S. M., Daub, D. M., and White, K. (1999). Differential and inefficient splicing of a broadly expressed Drosophila erect wing transcript results in tissue-specific enrichment of the vital EWG protein isoform. Mol. Cell Biol. 19, 3998–4007. doi: 10.1128/MCB.19.6.3998
Lefer, D., Perisse, E., Hourcade, B., Sandoz, J., and Devaud, J. M. (2012). Two waves of transcription are required for long-term memory in the honeybee. Learn. Mem. 20, 29–33.
Liu, G., Li, W., Wang, L., Kar, A., Guan, K. L., Rao, Y., et al. (2009). DSCAM functions as a netrin receptor in commissural axon pathfinding. Proc. Natl. Acad. Sci. U. S. A. 106, 2951–2956.
Liu, H., Caballero-Florán, R. N., Hergenreder, T., Yang, T., Hull, J. M., Pan, G., et al. (2023). DSCAM gene triplication causes excessive GABAergic synapses in the neocortex in Down syndrome mouse models. PLoS Biol. 21:e3002078. doi: 10.1371/journal.pbio.3002078
Lowe, S. A., Hodge, J. J. L., and Usowicz, M. M. (2018). A third copy of the Down syndrome cell adhesion molecule (Dscam) causes synaptic and locomotor dysfunction in Drosophila. Neurobiol. Dis. 110, 93–101. doi: 10.1016/j.nbd.2017.11.013
Matthews, B. J., Kim, M. E., Flanagan, J. J., Hattori, D., Clemens, J. C., Zipursky, S. L., et al. (2007). Dendrite self-avoidance is controlled by Dscam. Cell 129, 593–604. doi: 10.1016/j.cell.2007.04.013
Miura, S. K., Martins, A., Zhang, K. X., Graveley, B. R., and Zipursky, S. L. (2013). Probabilistic splicing of Dscam1 establishes identity at the level of single neurons. Cell 155, 1166–1177. doi: 10.1016/j.cell.2013.10.018
Mota, T., and Giurfa, M. (2010). Multiple reversal olfactory learning in honeybees. Front. Behav. Neurosci. 4:48. doi: 10.3389/fnbeh.2010.00048
Neves, G., Zucker, J., Daly, M., and Chess, A. (2004). Stochastic yet biased expression of multiple Dscam splice variants by individual cells. Nat. Genet. 36, 240–246. doi: 10.1038/ng1299
Noyes, N. C., Phan, A., and Davis, R. L. (2021). Memory suppressor genes: Modulating acquisition, consolidation, and forgetting. Neuron 109, 3211–3227. doi: 10.1016/j.neuron.2021.08.001
Palavalli, A., Tizón-Escamilla, N., Rupprecht, J. F., and Lecuit, T. (2021). Deterministic and stochastic rules of branching govern dendrite morphogenesis of sensory neurons. Curr. Biol. 31, 459–472.e4. doi: 10.1016/j.cub.2020.10.054
Poplawski, S. G., Peixoto, L., Porcari, G. S., Wimmer, M. E., Mcnally, A. G., Mizuno, K., et al. (2016). Contextual fear conditioning induces differential alternative splicing. Neurobiol. Learn. Mem. 134(Pt B), 221–235. doi: 10.1016/j.nlm.2016.07.018
Schmucker, D., and Chen, B. (2009). Dscam and DSCAM: Complex genes in simple animals, complex animals yet simple genes. Genes Dev. 23, 147–156.
Schmucker, D., Clemens, J. C., Shu, H., Worby, C. A., Xiao, J., Muda, M., et al. (2000). Drosophila Dscam is an axon guidance receptor exhibiting extraordinary molecular diversity. Cell 101, 671–684. doi: 10.1016/s0092-8674(00)80878-8
Sengar, A. S., Li, H., Zhang, W., Leung, C., Ramani, A. K., Saw, N. M., et al. (2019). Control of long-term synaptic potentiation and learning by alternative splicing of the NMDA receptor subunit GluN1. Cell Rep. 29:e5. doi: 10.1016/j.celrep.2019.11.087
Soba, P., Zhu, S., Emoto, K., Younger, S., Yang, S. J., Yu, H. H., et al. (2007). Drosophila sensory neurons require Dscam for dendritic self-avoidance and proper dendritic field organization. Neuron 54, 403–416. doi: 10.1016/j.neuron.2007.03.029
Soller, M. (2006). Pre-messenger RNA processing and its regulation: A genomic perspective. Cell Mol Life Sci 63, 796–819.
Soller, M., and White, K. (2005). ELAV multimerizes on conserved AU4-6 motifs important for ewg splicing regulation. Mol. Cell Biol. 25, 7580–7591. doi: 10.1128/MCB.25.17.7580-7591.2005
Stern, S. A., and Alberini, C. M. (2013). Mechanisms of memory enhancement. Wiley Interdiscip. Rev. Syst. Biol. Med. 5, 37–53.
Torres-Mendez, A., Bonnal, S., Marquez, Y., Roth, J., Iglesias, M., Permanyer, J., et al. (2019). A novel protein domain in an ancestral splicing factor drove the evolution of neural microexons. Nat. Ecol. Evol. 3, 691–701. doi: 10.1038/s41559-019-0813-6
Torres-Méndez, A., Pop, S., Bonnal, S., Almudi, I., Avola, A., Roberts, R. J. V., et al. (2022). Parallel evolution of a splicing program controlling neuronal excitability in flies and mammals. Sci. Adv. 8:eabk0445. doi: 10.1126/sciadv.abk0445
Ule, J., and Blencowe, B. J. (2019). Alternative splicing regulatory networks: Functions, mechanisms, and evolution. Mol. Cell 76, 329–345.
Ustaoglu, P., Gill, J. K., Doubovetzky, N., Haussmann, I. U., Dix, T. C., Arnold, R., et al. (2021). Dynamically expressed single ELAV/Hu orthologue elavl2 of bees is required for learning and memory. Commun. Biol. 4:1234. doi: 10.1038/s42003-021-02763-1
Ustaoglu, P., Haussmann, I. U., Liao, H., Torres-Mendez, A., Arnold, R., Irimia, M., et al. (2019). Srrm234, but not canonical SR and hnRNP proteins, drive inclusion of Dscam exon 9 variable exons. RNA 25, 1353–1365. doi: 10.1261/rna.071316.119
Vacca, R. A., Bawari, S., Valenti, D., Tewari, D., Nabavi, S. F., Shirooie, S., et al. (2019). Down syndrome: Neurobiological alterations and therapeutic targets. Neurosci. Biobehav. Rev. 98, 234–255.
Villar, M. E., Marchal, P., Viola, H., and Giurfa, M. (2020). Redefining single-trial memories in the honeybee. Cell Rep. 30:e3. doi: 10.1016/j.celrep.2020.01.086
Vuong, C. K., Black, D. L., and Zheng, S. (2016). The neurogenetics of alternative splicing. Nat. Rev. Neurosci. 17, 265–281.
Wang, J., Zugates, C. T., Liang, I. H., Lee, C. H., and Lee, T. (2002). Drosophila Dscam is required for divergent segregation of sister branches and suppresses ectopic bifurcation of axons. Neuron 33, 559–571. doi: 10.1016/s0896-6273(02)00570-6
Watson, F. L., Puttmann-Holgado, R., Thomas, F., Lamar, D. L., Hughes, M., Kondo, M., et al. (2005). Extensive diversity of Ig-superfamily proteins in the immune system of insects. Science 309, 1874–1878. doi: 10.1126/science.1116887
Wojtowicz, W. M., Flanagan, J. J., Millard, S. S., Zipursky, S. L., and Clemens, J. C. (2004). Alternative splicing of Drosophila Dscam generates axon guidance receptors that exhibit isoform-specific homophilic binding. Cell 118, 619–633. doi: 10.1016/j.cell.2004.08.021
Wojtowicz, W. M., Wu, W., Andre, I., Qian, B., Baker, D., and Zipursky, S. L. (2007). A vast repertoire of Dscam binding specificities arises from modular interactions of variable Ig domains. Cell 130, 1134–1145. doi: 10.1016/j.cell.2007.08.026
Zaharieva, E., Chipman, J. K., and Soller, M. (2012). Alternative splicing interference by xenobiotics. Toxicology 296, 1–12.
Keywords: Dscam, alternative splicing, learning and memory, honey bee, RNA interference (gene silencing)
Citation: Ustaoglu P, McQuarrie DWJ, Rochet A, Dix TC, Haussmann IU, Arnold R, Devaud J-M and Soller M (2024) Memory consolidation in honey bees is enhanced by down-regulation of Down syndrome cell adhesion molecule and changes its alternative splicing. Front. Mol. Neurosci. 16:1322808. doi: 10.3389/fnmol.2023.1322808
Received: 16 October 2023; Accepted: 13 December 2023;
Published: 09 January 2024.
Edited by:
Fabio Mammano, University of Padua, ItalyReviewed by:
Luis R. Hernandez-Miranda, Charité University Medicine Berlin, GermanySourav Banerjee, National Brain Research Centre (NBRC), India
Copyright © 2024 Ustaoglu, McQuarrie, Rochet, Dix, Haussmann, Arnold, Devaud and Soller. This is an open-access article distributed under the terms of the Creative Commons Attribution License (CC BY). The use, distribution or reproduction in other forums is permitted, provided the original author(s) and the copyright owner(s) are credited and that the original publication in this journal is cited, in accordance with accepted academic practice. No use, distribution or reproduction is permitted which does not comply with these terms.
*Correspondence: Matthias Soller, m.soller@bham.ac.uk