- 1Department of Neurosurgery, The Second Affiliated Hospital, School of Medicine, Zhejiang University, Hangzhou, China
- 2Department of Neurosurgery, The Fourth Affiliated Hospital, International Institutes of Medicine, Zhejiang University School of Medicine, Yiwu, China
- 3Brain Research Institute, Zhejiang University, Hangzhou, China
- 4Collaborative Innovation Center for Brain Science, Zhejiang University, Hangzhou, China
- 5Clinical Research Center for Neurological Diseases of Zhejiang Province, Hangzhou, China
Glioma is the most common intracranial malignant tumor in adults and the 5-year survival rate of glioma patients is extremely poor, even in patients who received Stupp treatment after diagnosis and this forces us to explore more efficient clinical strategies. At this time, immunotherapy shows great potential in a variety of tumor clinical treatments, however, its clinical effect in glioma is limited because of tumor immune privilege which was induced by the glioma immunosuppressive microenvironment, so remodeling the immunosuppressive microenvironment is a practical way to eliminate glioma immunotherapy resistance. Recently, increasing studies have confirmed that ferroptosis, a new form of cell death, plays an important role in tumor progression and immune microenvironment and the crosstalk between ferroptosis and tumor immune microenvironment attracts much attention. This work summarizes the progress studies of ferroptosis in the glioma immune microenvironment.
Introduction
Glioma is a threatening primary malignancy tumor in the central nervous system (1, 2), which is divided into grades I-IV according to WHO standard with glioblastoma (WHO grade IV) as the most malignant and common subtype (3). The standard therapy for glioma patients is the Stupp protocol, which consists of maximal safe surgical resection or a diagnostic biopsy, followed by concurrent chemoradiotherapy and then maintenance chemotherapy, where chemotherapy is comprised of temozolomide (4). Although glioblastoma (WHO IV) patients receive the most effective treatment/surgery with radiotherapy and chemotherapy after diagnosis (5, 6), the median survival time is only about 18 months (7), and that is mainly the result of a glioma infiltration boundary and/or the resistance of chemotherapy. Consequently, new therapeutic approaches for glioma are urgently needed (8).
Recently, immunotherapy represented by PD-1/PD-L1 and CTLA-4 has shown excellent clinical effects on numerous tumors such as melanoma and non-small cell lung cancer (9, 10), which have rekindled researchers’ faith in glioma treatment. Unfortunately, its effect is extremely limited in glioma and relevant clinical data show that it works on less than 10% of glioblastoma patients (11) An increasing number of studies have confirmed that it is a result of the glioma immunosuppressive microenvironment (12), therefore, the distruption of the immunosuppressive microenvironment and revision of the glioma from a ‘cold tumor’ to a ‘hot tumor’ is practical to relieve the glioma immunotherapy resistance (8).
The glioma immune microenvironment is composed of glioma cells, immune cells, cytokines and so on (12). Glioma cells can recruit numerous kinds of cell including immune cells that move to the niche by secreting cytokines (like TGF-β, GM-CSF) (13, 14) and then revise these cells into ‘tumor-friendly’ phenotypes (15). In this case, the recruited cells may serve as a physical barrier to prevent later immune cells from approaching and attacking the tumor cells Also, the recruited immune cells can also secrete cytokines (such as IL1-β, TGF-β) that continue to assimilate later recruited immune cells as ‘tumor-friendly’ phenotypes (16, 17). Under this “snowball” interaction, coupled with the unique central nervous system microenvironment, such as the blood-brain barrier (18) and hypoxia (19, 20), acidic tumor microenvironment (2, 21), tumor cells can escape immune surveillance (22) and eventually set the glioma immunosuppressive microenvironment (23, 24).
Ferroptosis is a form of regulated cell death driven by lipid peroxidation, a consequence of imbalance between cell metabolism and redox homeostasis (25). It is different from other cell death such as apoptosis, pyroptosis in morphology, biochemistry and gene (26). Its key process is phospholipids with polyunsaturated fatty acyl tails (PUFAs) are oxidized in an iron- or oxidoreductase- dependant way and ultimately induce cell death (27). Recently, researchers found that activating ferroptosis could improve temozolomide treatment effectiveness in GBM-bearing mice (28), and lonizing radiation could induce cell ferroptosis. The above means that ferroptosis is vital for glioma chemotherapy and radiotherapy (29).
Overview of Ferroptosis and Potential Singling Pathway in Glioma
The main characteristics of ferroptosis include: cell morphology (mitochondria crista, volume reduction, and increase of membrane density); cellular composition [cellular ROS is elevated and lipid peroxidation is significantly increased (27)]. Meanwhile, the intracellular pool of antioxidant executor (GSH or/and glutathione peroxidase 4) was shrunk, and phospholipid peroxide (PLOOH) is the executive driver of ferroptosis (26, 27). With step by step studies, researchers found that ferroptosis could be regulated by a variety of ways including redox homeostasis (30), iron metabolism (31), mitochondrial activity (32), metabolism of amino acids, lipids, and glucose (33). Ferroptosis pathways can be broadly divided into glutathione peroxidase 4 (GPX4) -dependent and -independent pathways (25, 26) (Figure 1).
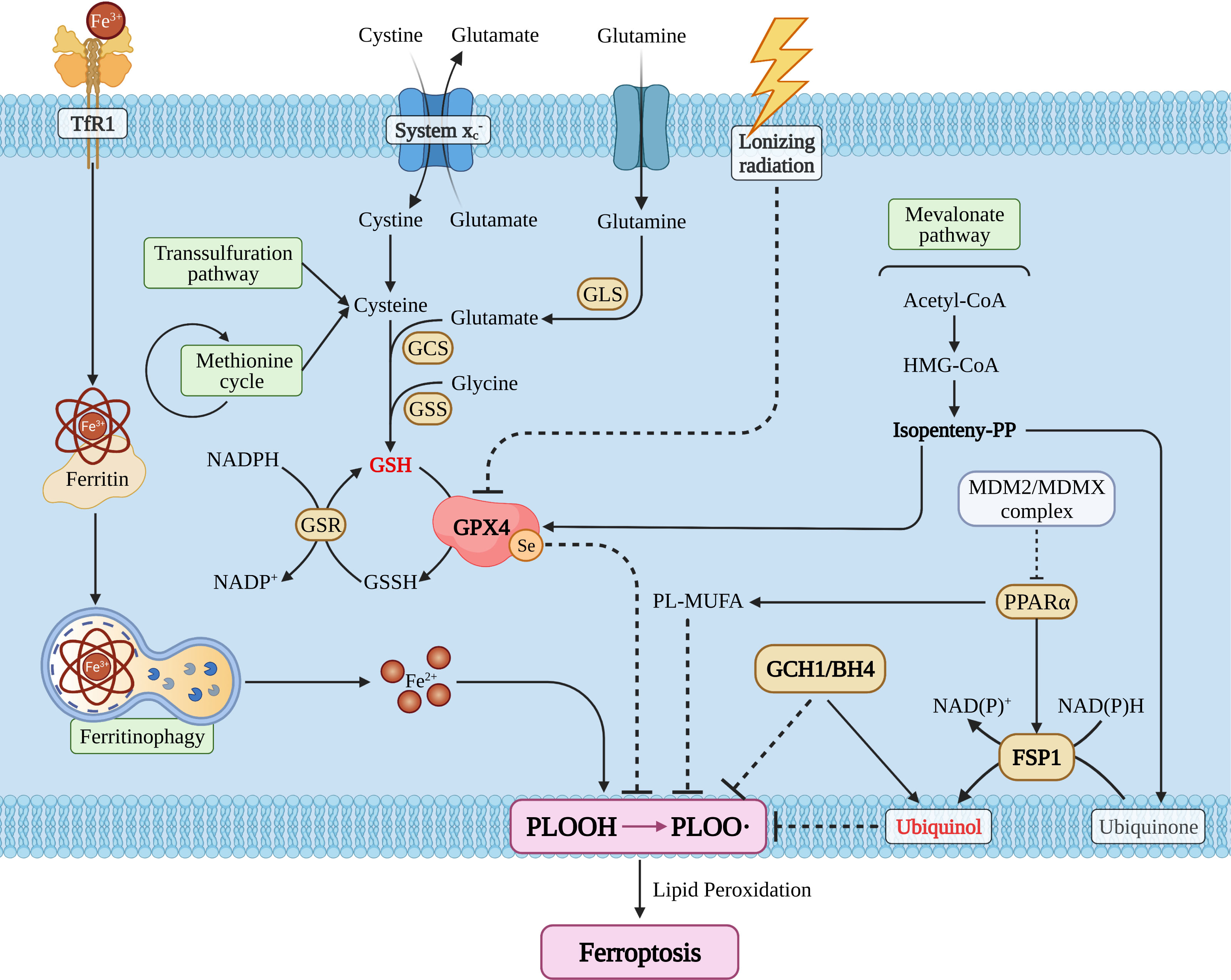
Figure 1 The snapshot of ferroptosis pathways. TfR1, transferrin receptor 1; GLS, glutaminase; GCS, glutamylcysteine synthetase; GSS, glutathione synthetase; GSH/GSSH, glutathione; GSR, glutathione S-reductase; GPX4, glutathione peroxidase 4; MDM2, mouse double minute 2; MDMX, mouse double minute 4; PPARα, peroxisome proliferator activated receptor alpha; FSP1, ferroptosis suppressor protein 1; GCH1, GTP cyclohydrolase 1; BH4, tetrahydrobiopterin; PL, phospholipid; MUFA, monounsaturated fatty acid.
GPX4 Dependent Ferroptosis Pathway
Glutathione peroxidase 4 (GPX4), also known as phospholipid hydrogen peroxide glutathione peroxidase (PHGPx), is a selenoprotein required for peroxidized phospholipids (34). Cystine/glutamic acid reverse transporter (system ) is an upstream regulator (25, 35) and its dysfunction can increase glutamic acid levels and reduce cystine levels (36), which in turn leads to the exhaustion of the intracellular pool of glutathione (GSH), the main reducing substance of human body (37). Subsequently, this causes GPX4 reduction (27), then induces more PUFAs to turn to PLOOH and eventually induces ferroptosis (25). Besides, lonizing radiation also could regulate GPX4 activity directly and then shape ferroptosis (38).
System plays an important role in GPX4 relative pathway, whether the system dysfunction could result in the pool of GSH and GPX4 shrinking (35), and then gives birth to intracellular PLOOH explode and ultimately induces ferroptosis (26, 33). The monitors regulating system are SLC7A11 (39), SLC3A2 (40), NRF2 (41) and so on (42). Stephanie demonstrated that SLC7A11 expression is associated with seizures and predicts poor survival in patients with malignant glioma (43) Ju et al. proved that NRF2 is a potential prognostic biomarker and is correlated with immune infiltration in the brain’s lower grade glioma (44). Long et al. found that dysregulation of system enhances Treg function that promotes VEGF blockade resistance in glioblastoma (45). The above indicates that system should be a key hub between ferroptosis and the glioma immune-microenvironment.
Cystine metabolism is a vital segment in the GPX4-dependent ferroptosis pathway and the main factors affecting cystine metabolism include the transsulfuration pathway and/or the methionine cycle (46). As a vital brick for GSH synthesis, cystine plays a key role in glioma progression, Liu et al. confirmed that methionine and cystine double deprivation stress suppresses glioma proliferation by inducing reactive oxygen species (ROS) and autophagy (47), Wang et al. demonstrated that methionine deprivation can reset numerous immune pathways such as macrophages, T cell activation pathways in glioma (48), as cystine and methionine are all in methionine cycle (49), and there should be cystine/methionine-ferroptosis-immunity related pathways. Simultaneously, glioma cells can selectively uptake methionine, cysteine, and serine (47, 50, 51), so other cells will uptake or store less of these amino acids than glioma cells, which limits the production of cysteine and GSH. It remains to be determined whether it would induce other cells to include immune cells more sensitive to ferroptosis than glioma cells and whether DNA/RNA methylation is vital for glioma escape ferroptosis, as methionine is the major methyl donor (52, 53). Unfortunately, the researchers did not conduct this corresponding work.
In addition, the mevalonate pathway also participated in GPX4 activity regulation and isopentenyl pyrophosphate was the core factor regulating the transcription efficiency of GPX4 (54). E. Cimini et al. has confirmed that zoledronic acid, an aminobisphosphonate drug, can inhibit glioma cell proliferation by interfering with mevalonate pathway of Vγ2 T-cells (55). Deven found that LXRβ knockdown decreased cell cycle progression, cell survival, and decreased feedback repression of the mevalonate pathway in densely-plated glioma cells. LXRβ regulates the expression of immune response gene sets and lipids known to be involved in immune modulation (56) and these works imply that targeting the mevalonate pathway could disturb ferroptosis and immunity in glioma.
Currently, researchers have demonstrated that lonizing radiation could consume GSH, inhibit GPX4 activity, and induce ferroptosis (25, 54), and they denote that ferroptosis should be essential for glioma treatment because radiotherapy is an important part of Stupp strategy (8). Zhang et al. revealed that inhibition of TAZ contributes to radiation-induced senescence and growth arrest in glioma, and immune-related genes are specifically affected as the long-term effect (57). However, we did not know whether ferroptosis cells would act as or release cytokines that induce glioma cells to adapt to radiotherapy resistance.
GPX4 Independent Ferroptosis Pathway
Although GPX4 is the core molecule of ferroptosis, we have now found other pathways that influence PLOOH synthesis and ferroptosis (25, 26).
The first is the ferroptosis inhibition protein 1(FSP1) (58–60), which can reduce the mevalonate pathway produced ubiquinone translate to ubiquinol, suppress production of PLOOH, and eventually inhibit ferroptosis (58). Furthermore, FSP1 could also be activated by the MDM2/MDMX-PPARα axis (25, 61), and in addition to activating FSP1 functions, PPARα also regulates the conversion of PL-MUFA to PLOOH by ACSL3-mediated MUFA way. It has been reported that FSP1 can protect cells from ferroptosis which is induced by GPX4 inhibition/knockout (26). Zou et al. demonstrated that TGF−β1 increases FSP1 expression in human bronchial epithelial cells (62), as TGF−β1 is an important cytokine that can be secreted by glioma or/and immune cell (17, 63, 64), and it means that FSP1 could be a nexus between glioma or/and immune cell ferroptosis.
A critical factor in inducing ferroptosis is the imbalance of intracellular iron metabolism which could cause iron overload. Through the specific receptor TFR1 (transferrin receptor 1), circulating iron (Fe3+) can be imported into the cell and stored mostly within ferritin (Fe3+), changing to cytoplasmic iron (65). A small pool of cytoplasmic free Fe2+ could directly catalyze the formation of free radical formation via the Fenton Reaction where changes of ferritin expression levels affect the homeostasis of iron metabolism by altering the intracellular free and redox active iron pool. Researchers have reported that the overexpression of NCOA4 reinforces the degradation of ferritin, which releases excessive cytoplasmic free Fe2+ and subsequently, promotes ferroptosis (66).
As a “double-edged sword”, autophagy is crucial in glioma progress (67, 68) due to the unbridled proliferation tumor cells that require a large amount of nutrients. Also, an appropriate level of autophagy is conducive to ensure necessary cellular function, as their own bricks, could be reused but excessive levels of autophagy will induce cell ‘self-digestion’ and eventually induce glioma death (69). Recent studies have proven that autophagy can participate in ferroptosis and the main progress is called ferritinophagy (25), and the key interaction hub of these two pathways is NCOA4 and FTH1. Zhang et al. confirmed that COPZ1 is the key molecule that mediates autophagy-dependent ferroptosis in glioma (70). Meanwhile, autophagy is essential for immune cell proliferation and function, and Enyong confirmed that autophagy-dependent ferroptosis drives tumor-associated macrophage polarization via the release and uptake of the oncogenic KRAS protein (71). Sun et al. confirmed that autophagy-dependent ferroptosis-related signature is closely associated with the prognosis and tumor immune escape of patients with glioma (72). Therefore, we recognize autophagy should be one of the hubs between ferroptosis and immunity in glioma.
Moreover, researchers have also confirmed that GTP cyclohydrase 1 (GCH1) inhibits the production of PLOOH through its metabolite BH2/4. Meanwhile, BH4 could also reduce PLOOH pool by regulating the production of Ubiquinol (26). Anh proved that the GCH1 knockdown with short hairpin RNA led to GBM cell growth inhibition and reduced self-renewal in association with decreased CD44 expression (73). Yan et al. showed that blocking CD44 inhibited glioma cell proliferation by regulating autophagy (67) and this means GCH1 could induce glioma cell ferroptosis and influence immunity by autophagy.
Furthermore, AMPK associated energy stress and Hippo pathways are all associated with ferroptosis by regulating the PLOOH pool (25, 26), and these factors are also vital for the glioma immunosuppressive microenvironment (GIME) and glioma proliferation (24).
Ferroptosis and Immune Microenvironment
Inducing tumor cell death is one of the effective methods to treat cancer, so inducing cancer cell ferroptosis is a feasible way for glioma treatment (34). Dead cells can release a series of “find me” and “eat me” signals for immune cells to locate, migrate, and clean dead cells which is confirmed by the phenomenon that ferroptosis tumor cells can be effectively engulfed by macrophages in vitro (74). The calreticulin (CRT), a soluble ER-associated chaperone, is one of the ferroptosis-mediated proteins which regulate the tumor microenvironment. Ferroptosis facilitates the translocation of CRT to expose it on the surface of tumor cells, where CRT could serve as a potent “eat-me” signal and induce a robust antitumor immune response (75). However, the signal communication between ferroptosis glioma cells and surrounding immune cells is not clear (76) (Figure 2).
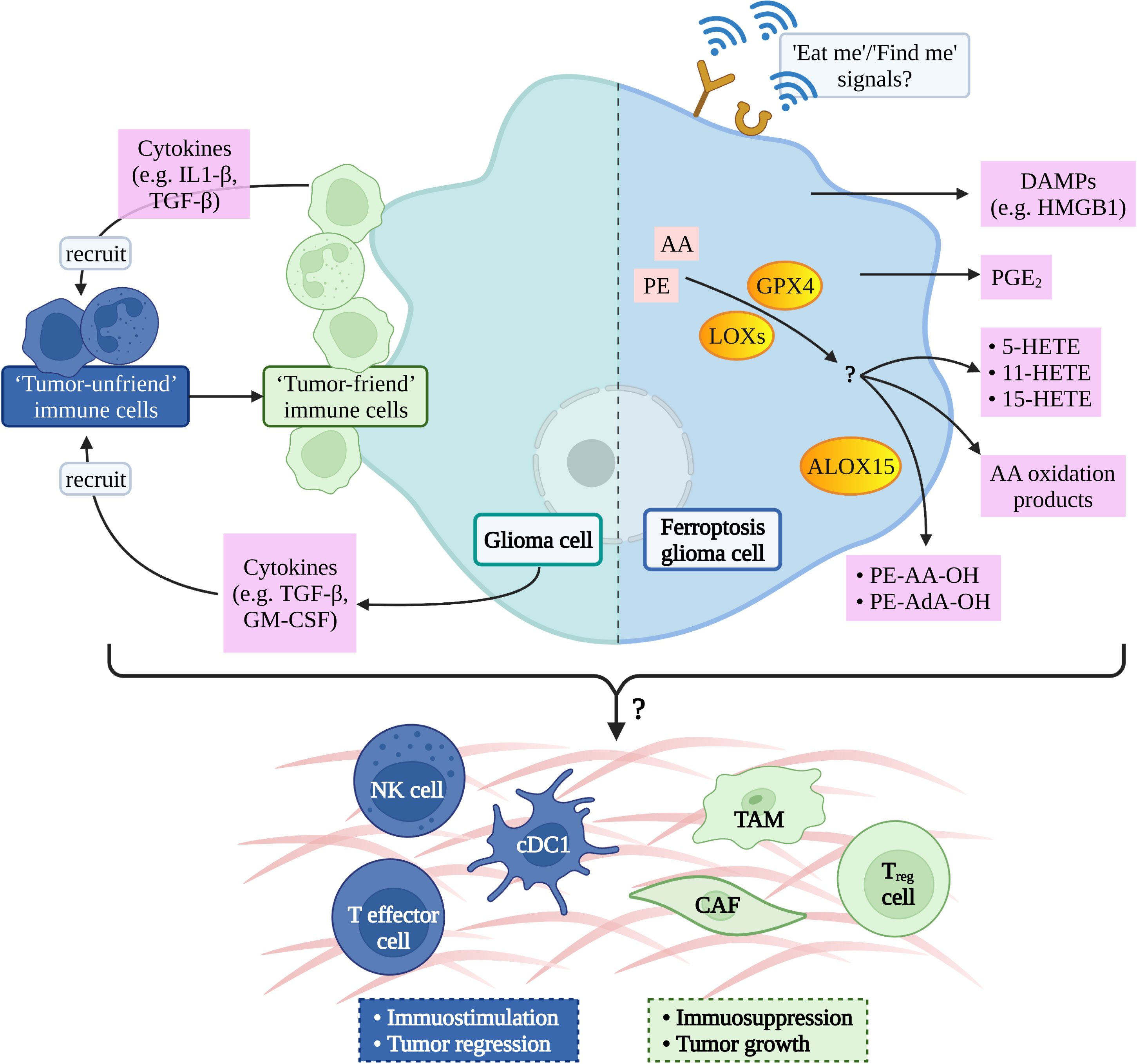
Figure 2 Possible ferroptotic signals in glioma immune-microenvironment. AA, arachidonic acid; PE, phosphatidylethanolamine; GPX4, glutathione peroxidase 4; LOXs, lipoxygenases; ALOX15, arachidonate lipoxygenase 15; DAMPs, damage-associated molecular patterns; HMGB1, high mobility group box-1; PGE2, prostaglandin E2; HETE, hydroxy eicosatetraenoic acid; AdA, adrenic acid; IL1, interleukin-1; TGF, transforming growth factor; GM-CSF, granulocyte-macrophage colony-stimulating factor; NK, natural killer; cDC1, conventional type 1 dendritic cell; TAM, tumor- associated macrophage; CAF, cancer-associated fibroblast; T reg cell, regulatory T cell.
The potential signal is the arachidonic acid (AA) oxidation product released by ferroptosis cells therefore, it has been hypothesized that lipoxygenases (LOXs) can not only induce the PUFAs production but also promote ferroptosis cells to release immune signals and regulate tumor immunity (26). A study has shown that ferroptosis cells can release eicosanoids (5-HETE, 11-HETE, 15-HETE, etc.) when GPX4 was suppressed. Contrarily, ferroptosis cells reduce the production of pro-inflammatory lipids when GPX4 activity was increasing, afterwards inhibiting the production of TNF and IL-1 β by the NF-kB pathway (77). A liposome analysis of ferroptosis cells found that the accumulation of oxygenated AA-containing phosphatidylethanolamine species was associated with ALOX15 (78), which can shape adaptive immune response by inhibiting dendritic cell maturation and T cell helper cell 17 (TH17) differentiation via activating transcription factor NRF2 (79).
Prostaglandin E2 (PGE2) is considered to be one of the important immunosuppressive factors and it can be released after most death cells (26, 80) and then disturb immune cells mainly in the following ways: 1. directly inhibit NK, cytotoxic T cell clean function (81, 82), 2. inhibit the infiltration of Conventional Type 1 dendritic cell (cDC1) into tumor niche via inhibiting the secretion of CCL5 and XCL1 by NK cells (83), and 3. inhibit cDC1-dependent CD8+ T cell-mediated immune response (84). Yoshiteru proved that inhibition of macrophagic PGE2 synthesis is an effective treatment for the induction of anti-glioma immune response (85).
Recent studies have confirmed that GPX4 activity is associated with chronic inflammation (26), and current studies have confirmed that glioma progression is related to chronic inflammation (86). Moreover, Xu et al. demonstrated that GPX4 is crucial for protecting activated Treg cells from lipid peroxidation and ferroptosis and offered a potential therapeutic strategy to improve cancer treatment (87). All of the above has to remind us that GPX4 may be a hub to connect ferroptosis and inflammation/immune in glioma.
In addition to releasing lipid mediators, ferroptosis cells can also release HMGB1 in an autophagy-dependent manner (88). HMGB1 belongs to DAMPs and is one of the key elements for tumor cell immunogenicity, as it will bind to its receptor and activate the immune system once it is released outside of cell (89). Wen et al. confirmed that RAGE is essential for HMGB1 mediated TNF releasing in macrophage when they respond to ferroptosis cells (90). Lowenstein et al. considered that HMGB1-activated dendritic cells, loaded with glioma antigens, migrate to cervical lymph nodes to stimulate a systemic CD8+ T cells cytotoxic immune response against glioma and induce immunological memory (91).
In addition to the above cytokines, there are other cytokines worth exploring (76). Although researchers believe that the cytokines are critical for the “crosstalk” between ferroptosis cells and immune cells, the mechanism remains unclear. Additionally, attention should also be paid to off-target effects of ferroptosis induction (92).
Challenges of Ferroptosis in GIME
The glioma immunosuppressive microenvironment (GIME) is the main reason for poor efficacy of immunotherapy in glioma (8, 22, 23). The rapid proliferation of glioma causes an arduous microenvironment such as acidity, limited of nutrients, and oxygen (47, 93, 94). In this circumstance, immune cells will betray, retreat, or die as they cannot adapt (95, 96) but glioma can adapt to this harsh microenvironment due to their own tremendous plasticity (97, 98). The blood-brain barrier can also hinder immune cells from migrating to the tumor (99, 100). Also, many of the inhibitory cytokines secreted by glioma (101) and inhibitory immune cells suppress the antitumor effect of immune cells (21, 102). In addition, glioma cells can also secrete numerous cytokines to trap immune cells as they present ‘non-tumor cells’ markers (95, 103) and then these “tricked” cells secrete cytokines and continue to later recruit immune cells (104). In this circumstance, glioma cells escape immune surveillance (22, 105)and we should take the above into account when considering glioma immunotherapy (106). Meanwhile, immunotherapy combination regimens (22), administration mode, and timing (107) can also influence the therapeutic efficacy. Currently, accruing studies demonstrate that ferroptosis is crucial for tumor progression and targeting ferroptosis maybe a latent way to remodel the tumor immune microenvironment (26, 27, 34). While we have already done a brief description above, we should also recognize the challenges.
Although ferroptosis does play a crucial role various tumor immune microenvironments, its own mechanism is still unclear (25, 26), which is reflected on the following aspects: 1. The exactly mechanism of PLOOH in ferroptosis is unclear. At present, although it is clear that PLOOH is the ultimate executor of ferroptosis, the exact mechanism of PLOOH inducing ferroptosis is unknown (26); 2. Ferroptosis studies lack a ‘gold standard’. Although we have made great progress in ferroptosis study (26), we have not yet found a relative “gold standard” like LC3, and P62 in autophagy (108) and researchers usually select one or more targets such as GPX4, P53, FTH1 (109–111) in a paper, even worse the targets just like scraped together, which has troubled the following researchers. 3. Ferroptosis shows a ‘double-edged sword’ role in diseases. It is easy to understand that ferroptosis plays different roles in different diseases such as the beneficial outcome of inducing ferroptosis in tumor cells is for disease (112), but inhibiting ferroptosis in stroke is beneficial to the prognosis (113). We hypothesize that ferroptosis may also play different roles in one disease, for example, and there may be tumor cells that choose to sacrifice themselves. Then, the secreted cytokines can make the surrounding tumor cells in a stress state and finally avoid ferroptosis (26). 4. What and how ferroptosis cells release signals after death and what are the functions of these signals (33, 71). 5. The crosstalk between ferroptosis and other forms of death is not clear (26, 27, 33). For cells that may suffer from different kinds of death at the same time (114), it is unknown how can they communicate with each other or whether ferroptosis works more or less in cell death. This is because we found cells suffer ferroptosis but cells still died after we used ferroptosis inhibitors in a proper dose. This means that ferroptosis can induce other kinds of cell death, and/or it plays a minor effect in cell death, or we use inhibitors after the ‘reversible point’ and once this threshold is exceeded, ferroptosis will be irreversible
Recently, we also found that ferroptosis is vital for tumor immunity such as macrophage phagocytosis (71) and T cell killing (115) but the “crosstalk” between ferroptosis and the glioma immunosuppressive microenvironment is not clear. Additional issues to be addressed are: 1. The signal interaction between ferroptosis cells and surrounding immune cells is not clear which is mainly manifested in the specific cytokines of ‘find me’ and ‘eat me’ released by ferroptosis cells (26). 2. Will the cytokines released by ferroptosis cells help other glioma cells escape immune surveillance by seducing or misleading immune cells (34, 88, 103)? 3. Whether GPX4-induced chronic inflammation engaged in glioma progression or outcome (116). 4. What is the role of ferroptosis in glioma immunotherapy tolerance? (95, 103).
Conclusion
The clinicians and researchers are always trying to find new treatments for tumors and it is comforting that treatment methods such as immunotherapy and oncolytic virus have been found. Unfortunately, immunotherapy, which has shed light on numerous tumor treatments, does not always work regarding glioma. Increasing research demonstrates that this is result of the glioma immunosuppressive microenvironment, so researchers are searching for an antidote for remodeling GIME. Ferroptosis, a new form of cell death, plays an important role in glioma cell and immune cell. The exactl mechanism is unclear and multipley works demonstrate that it is deserved to explore its role in GIME and how to regulate ferroptosis for glioma therapy. Although there are still many obstacles in the cognition of crosstalk between ferroptosis and GIME, we believe we will address this with further studies and new technologies, such single cell sequencing and spatial transcriptomics. This will not only improve our understanding of ferroptosis and GIME but also provide a new solution for glioma immunotherapy resistance, a new breakthrough point for glioma treatment.
Author Contributions
KW proposed the research. KW, JW, and JHZ wrote the manuscript and finalized the paper. AZ, YL, and JYZ both reviewed the literature and collected references. XW and JMZ reviewed the literature. All authors contributed to the article and approved the submitted manuscript.
Funding
This work was funded by National Natural Science Foundation of China (No. 82002634, 81171096 and 81371433).
Conflict of Interest
The authors declare that the research was conducted in the absence of any commercial or financial relationships that could be construed as a potential conflict of interest.
Publisher’s Note
All claims expressed in this article are solely those of the authors and do not necessarily represent those of their affiliated organizations, or those of the publisher, the editors and the reviewers. Any product that may be evaluated in this article, or claim that may be made by its manufacturer, is not guaranteed or endorsed by the publisher.
References
1. Ludwig N, Rao A, Sandlesh P, Yerneni SS, Swain AD, Bullock KM, et al. Characterization of Systemic Immunosuppression by Idh Mutant Glioma Small Extracellular Vesicles. Neuro Oncol (2022) 24(2):197–209. doi: 10.1093/neuonc/noab153
2. Singh D, Chan JM, Zoppoli P, Niola F, Sullivan R, Castano A, et al. Transforming Fusions of Fgfr and Tacc Genes in Human Glioblastoma. Science (2012) 337(6099):1231–5. doi: 10.1126/science.1220834
3. Weller M, Wick W, Aldape K, Brada M, Berger M, Pfister SM, et al. Glioma. Nat Rev Dis Primers (2015) 1:15017. doi: 10.1038/nrdp.2015.17
4. Jin L, Guo S, Zhang X, Mo Y, Ke S, Duan C. Optimal Treatment Strategy for Adult Patients With Newly Diagnosed Glioblastoma: A Systematic Review and Network Meta-Analysis. Neurosurg Rev (2021) 44(4):1943–55. doi: 10.1007/s10143-020-01403-2
5. Stupp R, Mason WP, van den Bent MJ, Weller M, Fisher B, Taphoorn MJ, et al. Radiotherapy Plus Concomitant and Adjuvant Temozolomide for Glioblastoma. N Engl J Med (2005) 352(10):987–96. doi: 10.1056/NEJMoa043330
6. Perry JR, Laperriere N, O'Callaghan CJ, Brandes AA, Menten J, Phillips C, et al. Short-Course Radiation Plus Temozolomide in Elderly Patients With Glioblastoma. N Engl J Med (2017) 376(11):1027–37. doi: 10.1056/NEJMoa1611977
7. McCutcheon IE, Preul MC. Historical Perspective on Surgery and Survival With Glioblastoma: How Far Have We Come? World Neurosurg (2021) 149:148–68. doi: 10.1016/j.wneu.2021.02.047
8. Keskin DB, Anandappa AJ, Sun J, Tirosh I, Mathewson ND, Li S, et al. Neoantigen Vaccine Generates Intratumoral T Cell Responses in Phase Ib Glioblastoma Trial. Nature (2019) 565(7738):234–9. doi: 10.1038/s41586-018-0792-9
9. Snyder A, Makarov V, Merghoub T, Yuan J, Zaretsky JM, Desrichard A, et al. Genetic Basis for Clinical Response to Ctla-4 Blockade in Melanoma. New Engl J Med (2014) 371(23):2189–99. doi: 10.1056/NEJMoa1406498
10. Garon EB, Rizvi NA, Hui R, Leighl N, Balmanoukian AS, Eder JP, et al. Pembrolizumab for the Treatment of Non-Small-Cell Lung Cancer. N Engl J Med (2015) 372(21):2018–28. doi: 10.1056/NEJMoa1501824
11. Jackson CM, Choi J, Lim M. Mechanisms of Immunotherapy Resistance: Lessons From Glioblastoma. Nat Immunol (2019) 20(9):1100–9. doi: 10.1038/s41590-019-0433-y
12. Hilf N, Kuttruff-Coqui S, Frenzel K, Bukur V, Stevanovic S, Gouttefangeas C, et al. Actively Personalized Vaccination Trial for Newly Diagnosed Glioblastoma. Nature (2019) 565(7738):240–5. doi: 10.1038/s41586-018-0810-y
13. Dougan M, Dranoff G, Dougan SK. Gm-Csf, Il-3, and Il-5 Family of Cytokines: Regulators of Inflammation. Immunity (2019) 50(4):796–811. doi: 10.1016/j.immuni.2019.03.022
14. Pyonteck SM, Akkari L, Schuhmacher AJ, Bowman RL, Sevenich L, Quail DF, et al. Csf-1r Inhibition Alters Macrophage Polarization and Blocks Glioma Progression. Nat Med (2013) 19(10):1264–72. doi: 10.1038/nm.3337
15. Abdelfattah N, Kumar P, Wang C, Leu JS, Flynn WF, Gao R, et al. Single-Cell Analysis of Human Glioma and Immune Cells Identifies S100a4 as an Immunotherapy Target. Nat Commun (2022) 13(1):767. doi: 10.1038/s41467-022-28372-y
16. Mantovani A, Dinarello CA, Molgora M, Garlanda C. Interleukin-1 and Related Cytokines in the Regulation of Inflammation and Immunity. Immunity (2019) 50(4):778–95. doi: 10.1016/j.immuni.2019.03.012
17. Eichhorn PJ, Rodon L, Gonzalez-Junca A, Dirac A, Gili M, Martinez-Saez E, et al. Usp15 Stabilizes Tgf-Beta Receptor I and Promotes Oncogenesis Through the Activation of Tgf-Beta Signaling in Glioblastoma. Nat Med (2012) 18(3):429–35. doi: 10.1038/nm.2619
18. Martin M, Vermeiren S, Bostaille N, Eubelen M, Spitzer D, Vermeersch M, et al. Engineered Wnt Ligands Enable Blood-Brain Barrier Repair in Neurological Disorders. Science (2022) 375(6582):eabm4459. doi: 10.1126/science.abm4459
19. Patel AP, Tirosh I, Trombetta JJ, Shalek AK, Gillespie SM, Wakimoto H, et al. Single-Cell Rna-Seq Highlights Intratumoral Heterogeneity in Primary Glioblastoma. Science (2014) 344(6190):1396–401. doi: 10.1126/science.1254257
20. Lee SB, Frattini V, Bansal M, Castano AM, Sherman D, Hutchinson K, et al. An Id2-Dependent Mechanism for Vhl Inactivation in Cancer. Nature (2016) 529(7585):172–7. doi: 10.1038/nature16475
21. Gieryng A, Pszczolkowska D, Walentynowicz KA, Rajan WD, Kaminska B. Immune Microenvironment of Gliomas. Lab Invest (2017) 97(5):498–518. doi: 10.1038/labinvest.2017.19
22. Xu S, Tang L, Li X, Fan F, Liu Z. Immunotherapy for Glioma: Current Management and Future Application. Cancer Lett (2020) 476:1–12. doi: 10.1016/j.canlet.2020.02.002
23. Zhao J, Chen AX, Gartrell RD, Silverman AM, Aparicio L, Chu T, et al. Immune and Genomic Correlates of Response to Anti-Pd-1 Immunotherapy in Glioblastoma. Nat Med (2019) 25(3):462–9. doi: 10.1038/s41591-019-0349-y
24. Ott M, Prins RM, Heimberger AB. The Immune Landscape of Common Cns Malignancies: Implications for Immunotherapy. Nat Rev Clin Oncol (2021) 18(11):729–44. doi: 10.1038/s41571-021-00518-9
25. Hadian K, Stockwell BR. Snapshot: Ferroptosis. Cell (2020) 181(5):1188– e1. doi: 10.1016/j.cell.2020.04.039
26. Jiang X, Stockwell BR, Conrad M. Ferroptosis: Mechanisms, Biology and Role in Disease. Nat Rev Mol Cell Biol (2021) 22(4):266–82. doi: 10.1038/s41580-020-00324-8
27. Dixon SJ, Lemberg KM, Lamprecht MR, Skouta R, Zaitsev EM, Gleason CE, et al. Ferroptosis: An Iron-Dependent Form of Nonapoptotic Cell Death. Cell (2012) 149(5):1060–72. doi: 10.1016/j.cell.2012.03.042
28. Chen Q, Wang W, Wu Z, Chen S, Chen X, Zhuang S, et al. Over-Expression of Lncrna Tmem161b-As1 Promotes the Malignant Biological Behavior of Glioma Cells and the Resistance to Temozolomide Via Up-Regulating the Expression of Multiple Ferroptosis-Related Genes by Sponging Hsa-Mir-27a-3p. Cell Death Discovery (2021) 7(1):311. doi: 10.1038/s41420-021-00709-4
29. Ye LF, Chaudhary KR, Zandkarimi F, Harken AD, Kinslow CJ, Upadhyayula PS, et al. Radiation-Induced Lipid Peroxidation Triggers Ferroptosis and Synergizes With Ferroptosis Inducers. ACS Chem Biol (2020) 15(2):469–84. doi: 10.1021/acschembio.9b00939
30. Yao X, Li W, Fang, Xiao C, Wu X, Li M, et al. Emerging Roles of Energy Metabolism in Ferroptosis Regulation of Tumor Cells. Adv Sci (Weinh) (2021) 8(22):e2100997. doi: 10.1002/advs.202100997
31. Yu Y, Jiang L, Wang H, Shen Z, Cheng Q, Zhang P, et al. Hepatic Transferrin Plays a Role in Systemic Iron Homeostasis and Liver Ferroptosis. Blood (2020) 136(6):726–39. doi: 10.1182/blood.2019002907
32. Gao M, Yi J, Zhu J, Minikes AM, Monian P, Thompson CB, et al. Role of Mitochondria in Ferroptosis. Mol Cell (2019) 73(2):354–63-e3. doi: 10.1016/j.molcel.2018.10.042
33. Xie Y, Hou W, Song X, Yu Y, Huang J, Sun X, et al. Ferroptosis: Process and Function. Cell Death Differ (2016) 23(3):369–79. doi: 10.1038/cdd.2015.158
34. Su Y, Zhao B, Zhou L, Zhang Z, Shen Y, Lv H, et al. Ferroptosis, a Novel Pharmacological Mechanism of Anti-Cancer Drugs. Cancer Lett (2020) 483:127–36. doi: 10.1016/j.canlet.2020.02.015
35. Tsuchihashi K, Okazaki S, Ohmura M, Ishikawa M, Sampetrean O, Onishi N, et al. The Egf Receptor Promotes the Malignant Potential of Glioma by Regulating Amino Acid Transport System Xc(-). Cancer Res (2016) 76(10):2954–63. doi: 10.1158/0008-5472.CAN-15-2121
36. de Groot J, Sontheimer H. Glutamate and the Biology of Gliomas. Glia (2011) 59(8):1181–9. doi: 10.1002/glia.21113
37. McBrayer SK, Mayers JR, DiNatale GJ, Shi DD, Khanal J, Chakraborty AA, et al. Transaminase Inhibition by 2-Hydroxyglutarate Impairs Glutamate Biosynthesis and Redox Homeostasis in Glioma. Cell (2018) 175(1):101–16.e25. doi: 10.1016/j.cell.2018.08.038
38. Lei G, Zhang Y, Koppula P, Liu X, Zhang J, Lin SH, et al. The Role of Ferroptosis in Ionizing Radiation-Induced Cell Death and Tumor Suppression. Cell Res (2020) 30(2):146–62. doi: 10.1038/s41422-019-0263-3
39. Yadav P, Sharma P, Sundaram S, Venkatraman G, Bera AK, Karunagaran D. Slc7a11/ Xct Is a Target of Mir-5096 and Its Restoration Partially Rescues Mir-5096-Mediated Ferroptosis and Anti-Tumor Effects in Human Breast Cancer Cells. Cancer Lett (2021) 522:211–24. doi: 10.1016/j.canlet.2021.09.033
40. Liu J, Xia X, Huang P. Xct: A Critical Molecule That Links Cancer Metabolism to Redox Signaling. Mol Ther (2020) 28(11):2358–66. doi: 10.1016/j.ymthe.2020.08.021
41. Dodson M, Castro-Portuguez R, Zhang DD. Nrf2 Plays a Critical Role in Mitigating Lipid Peroxidation and Ferroptosis. Redox Biol (2019) 23:101107. doi: 10.1016/j.redox.2019.101107
42. Liu Y, Chen Q, Zhu Y, Wang T, Ye L, Han L, et al. Non-Coding Rnas in Necroptosis, Pyroptosis and Ferroptosis in Cancer Metastasis. Cell Death Discovery (2021) 7(1):210. doi: 10.1038/s41420-021-00596-9
43. Robert SM, Buckingham SC, Campbell SL, Robel S, Holt KT, Ogunrinu-Babarinde T, et al. Slc7a11 Expression Is Associated With Seizures and Predicts Poor Survival in Patients With Malignant Glioma. Sci Transl Med (2015) 7(289):289ra86. doi: 10.1126/scitranslmed.aaa8103
44. Ju Q, Li X, Zhang H, Yan S, Li Y, Zhao Y. Nfe2l2 Is a Potential Prognostic Biomarker and Is Correlated With Immune Infiltration in Brain Lower Grade Glioma: A Pan-Cancer Analysis. Oxid Med Cell Longev (2020) 2020:3580719. doi: 10.1155/2020/3580719
45. Long Y, Tao H, Karachi A, Grippin AJ, Jin L, Chang YE, et al. Dysregulation of Glutamate Transport Enhances Treg Function That Promotes Vegf Blockade Resistance in Glioblastoma. Cancer Res (2020) 80(3):499–509. doi: 10.1158/0008-5472.CAN-19-1577
46. Ruiz-Rodado V, Dowdy T, Lita A, Kramp T, Zhang M, Jung J, et al. Cysteine Is a Limiting Factor for Glioma Proliferation and Survival. Mol Oncol (2021) 16(9):1777–94. doi: 10.1002/1878-0261.13148
47. Liu H, Zhang W, Wang K, Wang X, Yin F, Li C, et al. Methionine and Cystine Double Deprivation Stress Suppresses Glioma Proliferation Via Inducing Ros/Autophagy. Toxicol Lett (2015) 232(2):349–55. doi: 10.1016/j.toxlet.2014.11.011
48. Wang K, Liu H, Liu J, Wang X, Teng L, Zhang J, et al. Il1rn Mediates the Suppressive Effect of Methionine Deprivation on Glioma Proliferation. Cancer Lett (2019) 454:146–57. doi: 10.1016/j.canlet.2019.04.004
49. May JL, Kouri FM, Hurley LA, Liu J, Tommasini-Ghelfi S, Ji Y, et al. Idh3alpha Regulates One-Carbon Metabolism in Glioblastoma. Sci Adv (2019) 5(1):eaat0456. doi: 10.1126/sciadv.aat0456
50. Geisler S, Stegmayr C, Niemitz N, Lohmann P, Rapp M, Stoffels G, et al. Treatment-Related Uptake of O-(2-(18)F-Fluoroethyl)-L-Tyrosine and L-[Methyl-(3)H]-Methionine After Tumor Resection in Rat Glioma Models. J Nucl Med (2019) 60(10):1373–9. doi: 10.2967/jnumed.119.225680
51. Cornford EM, Young D, Paxton JW, Finlay GJ, Wilson WR, Pardridge WM. Melphalan Penetration of the Blood-Brain Barrier Via the Neutral Amino Acid Transporter in Tumor-Bearing Brain. Cancer Res (1992) 52(1):138–43.
52. Hervouet E, Debien E, Campion L, Charbord J, Menanteau J, Vallette FM, et al. Folate Supplementation Limits the Aggressiveness of Glioma Via the Remethylation of DNA Repeats Element and Genes Governing Apoptosis and Proliferation. Clin Cancer Res (2009) 15(10):3519–29. doi: 10.1158/1078-0432.CCR-08-2062
53. Maddocks OD, Labuschagne CF, Adams PD, Vousden KH. Serine Metabolism Supports the Methionine Cycle and DNA/Rna Methylation Through De Novo Atp Synthesis in Cancer Cells. Mol Cell (2016) 61(2):210–21. doi: 10.1016/j.molcel.2015.12.014
54. Zheng J, Conrad M. The Metabolic Underpinnings of Ferroptosis. Cell Metab (2020) 32(6):920–37. doi: 10.1016/j.cmet.2020.10.011
55. Cimini E, Piacentini P, Sacchi A, Gioia C, Leone S, Lauro GM, et al. Zoledronic Acid Enhances Vdelta2 T-Lymphocyte Antitumor Response to Human Glioma Cell Lines. Int J Immunopathol Pharmacol (2011) 24(1):139–48. doi: 10.1177/039463201102400116
56. Patel D, Ahmad F, Kambach DM, Sun Q, Halim AS, Kramp T, et al. Lxrbeta Controls Glioblastoma Cell Growth, Lipid Balance, and Immune Modulation Independently of Abca1. Sci Rep (2019) 9(1):15458. doi: 10.1038/s41598-019-51865-8
57. Zhang L, Cheng F, Wei Y, Zhang L, Guo D, Wang B, et al. Inhibition of Taz Contributes Radiation-Induced Senescence and Growth Arrest in Glioma Cells. Oncogene (2019) 38(15):2788–99. doi: 10.1038/s41388-018-0626-0
58. Doll S, Freitas FP, Shah R, Aldrovandi M, da Silva MC, Ingold I, et al. Fsp1 Is a Glutathione-Independent Ferroptosis Suppressor. Nature (2019) 575(7784):693–8. doi: 10.1038/s41586-019-1707-0
59. Bersuker K, Hendricks JM, Li Z, Magtanong L, Ford B, Tang PH, et al. The Coq Oxidoreductase Fsp1 Acts Parallel to Gpx4 to Inhibit Ferroptosis. Nature (2019) 575(7784):688–92. doi: 10.1038/s41586-019-1705-2
60. Mao C, Liu X, Zhang Y, Lei G, Yan Y, Lee H, et al. Dhodh-Mediated Ferroptosis Defence Is a Targetable Vulnerability in Cancer. Nature (2021) 593(7860):586–90. doi: 10.1038/s41586-021-03539-7
61. Venkatesh D, O'Brien NA, Zandkarimi F, Tong DR, Stokes ME, Dunn DE, et al. Mdm2 and Mdmx Promote Ferroptosis by Pparalpha-Mediated Lipid Remodeling. Genes Dev (2020) 34(7-8):526–43. doi: 10.1101/gad.334219.119
62. Zou Y, Song W, Zhou L, Mao Y, Hong W. House Dust Mite Induces Sonic Hedgehog Signaling That Mediates Epithelialmesenchymal Transition in Human Bronchial Epithelial Cells. Mol Med Rep (2019) 20(5):4674–82. doi: 10.3892/mmr.2019.10707
63. Nie E, Jin X, Miao F, Yu T, Zhi T, Shi Z, et al. Tgf-Beta1 Modulates Temozolomide Resistance in Glioblastoma Via Altered Microrna Processing and Elevated Mgmt. Neuro Oncol (2021) 23(3):435–46. doi: 10.1093/neuonc/noaa198
64. Quail DF, Bowman RL, Akkari L, Quick ML, Schuhmacher AJ, Huse JT, et al. The Tumor Microenvironment Underlies Acquired Resistance to Csf-1r Inhibition in Gliomas. Science (2016) 352(6288):aad3018. doi: 10.1126/science.aad3018
65. Battaglia AM, Chirillo R, Aversa I, Sacco A, Costanzo F, Biamonte F. Ferroptosis and Cancer: Mitochondria Meet the "Iron Maiden" Cell Death. Cells (2020) 9(6):1505. doi: 10.3390/cells9061505
66. Torii S, Shintoku R, Kubota C, Yaegashi M, Torii R, Sasaki M, et al. An Essential Role for Functional Lysosomes in Ferroptosis of Cancer Cells. Biochem J (2016) 473(6):769–77. doi: 10.1042/BJ20150658
67. Yan T, Chen X, Zhan H, Yao P, Wang N, Yang H, et al. Interfering With Hyaluronic Acid Metabolism Suppresses Glioma Cell Proliferation by Regulating Autophagy. Cell Death Dis (2021) 12(5):486. doi: 10.1038/s41419-021-03747-z
68. Feng X, Zhang H, Meng L, Song H, Zhou Q, Qu C, et al. Hypoxia-Induced Acetylation of Pak1 Enhances Autophagy and Promotes Brain Tumorigenesis Via Phosphorylating Atg5. Autophagy (2021) 17(3):723–42. doi: 10.1080/15548627.2020.1731266
69. Meyer N, Henkel L, Linder B, Zielke S, Tascher G, Trautmann S, et al. Autophagy Activation, Lipotoxicity and Lysosomal Membrane Permeabilization Synergize to Promote Pimozide- and Loperamide-Induced Glioma Cell Death. Autophagy (2021) 17(11):3424–43. doi: 10.1080/15548627.2021.1874208
70. Zhang Y, Kong Y, Ma Y, Ni S, Wikerholmen T, Xi K, et al. Loss of Copz1 Induces Ncoa4 Mediated Autophagy and Ferroptosis in Glioblastoma Cell Lines. Oncogene (2021) 40(8):1425–39. doi: 10.1038/s41388-020-01622-3
71. Dai E, Han L, Liu J, Xie Y, Kroemer G, Klionsky DJ, et al. Autophagy-Dependent Ferroptosis Drives Tumor-Associated Macrophage Polarization Via Release and Uptake of Oncogenic Kras Protein. Autophagy (2020) 16(11):2069–83. doi: 10.1080/15548627.2020.1714209
72. Sun W, Yan J, Ma H, Wu J, Zhang Y. Autophagy-Dependent Ferroptosis-Related Signature Is Closely Associated With the Prognosis and Tumor Immune Escape of Patients With Glioma. Int J Gen Med (2022) 15:253–70. doi: 10.2147/IJGM.S343046
73. Tran AN, Walker K, Harrison DG, Chen W, Mobley J, Hocevar L, et al. Reactive Species Balance Via Gtp Cyclohydrolase I Regulates Glioblastoma Growth and Tumor Initiating Cell Maintenance. Neuro Oncol (2018) 20(8):1055–67. doi: 10.1093/neuonc/noy012
74. Kloditz K, Fadeel B. Three Cell Deaths and a Funeral: Macrophage Clearance of Cells Undergoing Distinct Modes of Cell Death. Cell Death Discovery (2019) 5:65. doi: 10.1038/s41420-019-0146-x
75. Sacco A, Battaglia AM, Botta C, Aversa I, Mancuso S, Costanzo F, et al. Iron Metabolism in the Tumor Microenvironment-Implications for Anti-Cancer Immune Response. Cells (2021) 10(2):303. doi: 10.3390/cells10020303
76. Friedmann Angeli JP, Krysko DV, Conrad M. Ferroptosis at the Crossroads of Cancer-Acquired Drug Resistance and Immune Evasion. Nat Rev Cancer (2019) 19(7):405–14. doi: 10.1038/s41568-019-0149-1
77. Friedmann Angeli JP, Schneider M, Proneth B, Tyurina YY, Tyurin VA, Hammond VJ, et al. Inactivation of the Ferroptosis Regulator Gpx4 Triggers Acute Renal Failure in Mice. Nat Cell Biol (2014) 16(12):1180–91. doi: 10.1038/ncb3064
78. Kagan VE, Mao G, Qu F, Angeli JP, Doll S, Croix CS, et al. Oxidized Arachidonic and Adrenic Pes Navigate Cells to Ferroptosis. Nat Chem Biol (2017) 13(1):81–90. doi: 10.1038/nchembio.2238
79. Rothe T, Gruber F, Uderhardt S, Ipseiz N, Rossner S, Oskolkova O, et al. 12/15-Lipoxygenase-Mediated Enzymatic Lipid Oxidation Regulates Dc Maturation and Function. J Clin Invest (2015) 125(5):1944–54. doi: 10.1172/JCI78490
80. Yagami T, Koma H, Yamamoto Y. Pathophysiological Roles of Cyclooxygenases and Prostaglandins in the Central Nervous System. Mol Neurobiol (2016) 53(7):4754–71. doi: 10.1007/s12035-015-9355-3
81. Kalinski P. Regulation of Immune Responses by Prostaglandin E2. J Immunol (2012) 188(1):21–8. doi: 10.4049/jimmunol.1101029
82. Wang D, DuBois RN. Immunosuppression Associated With Chronic Inflammation in the Tumor Microenvironment. Carcinogenesis (2015) 36(10):1085–93. doi: 10.1093/carcin/bgv123
83. Bottcher JP, Bonavita E, Chakravarty P, Blees H, Cabeza-Cabrerizo M, Sammicheli S, et al. Nk Cells Stimulate Recruitment of Cdc1 Into the Tumor Microenvironment Promoting Cancer Immune Control. Cell (2018) 172(5):1022–37.e14. doi: 10.1016/j.cell.2018.01.004
84. Zelenay S, van der Veen AG, Bottcher JP, Snelgrove KJ, Rogers N, Acton SE, et al. Cyclooxygenase-Dependent Tumor Growth Through Evasion of Immunity. Cell (2015) 162(6):1257–70. doi: 10.1016/j.cell.2015.08.015
85. Nakano Y, Kuroda E, Kito T, Yokota A, Yamashita U. Induction of Macrophagic Prostaglandin E2 Synthesis by Glioma Cells. J Neurosurg (2006) 104(4):574–82. doi: 10.3171/jns.2006.104.4.574
86. Chobrutskiy BI, Yeagley M, Tipping P, Zaman S, Diviney A, Patel DN, et al. Chemical Complementarity Between Immune Receptor Cdr3s and Idh1 Mutants Correlates With Increased Survival for Lower Grade Glioma. Oncogene (2020) 39(8):1773–83. doi: 10.1038/s41388-019-1101-2
87. Xu C, Sun S, Johnson T, Qi R, Zhang S, Zhang J, et al. The Glutathione Peroxidase Gpx4 Prevents Lipid Peroxidation and Ferroptosis to Sustain Treg Cell Activation and Suppression of Antitumor Immunity. Cell Rep (2021) 35(11):109235. doi: 10.1016/j.celrep.2021.109235
88. Chen R, Kang R, Tang D. The Mechanism of Hmgb1 Secretion and Release. Exp Mol Med (2022) 54(2):91–102. doi: 10.1038/s12276-022-00736-w
89. Yamazaki T, Hannani D, Poirier-Colame V, Ladoire S, Locher C, Sistigu A, et al. Defective Immunogenic Cell Death of Hmgb1-Deficient Tumors: Compensatory Therapy With TLR4 Agonists. Cell Death Differ (2014) 21(1):69–78. doi: 10.1038/cdd.2013.72
90. Wen Q, Liu J, Kang R, Zhou B, Tang D. The Release and Activity of Hmgb1 in Ferroptosis. Biochem Biophys Res Commun (2019) 510(2):278–83. doi: 10.1016/j.bbrc.2019.01.090
91. Lowenstein PR, Castro MG. The Long and Winding Road: From the High-Affinity Choline Uptake Site to Clinical Trials for Malignant Brain Tumors. Adv Pharmacol (2016) 76:147–73. doi: 10.1016/bs.apha.2016.03.002
92. Dahlmanns M, Yakubov E, Dahlmanns JK. Genetic Profiles of Ferroptosis in Malignant Brain Tumors and Off-Target Effects of Ferroptosis Induction. Front Oncol (2021) 11:783067. doi: 10.3389/fonc.2021.783067
93. Li J, Liao T, Liu H, Yuan H, Ouyang T, Wang J, et al. Hypoxic Glioma Stem Cell-Derived Exosomes Containing Linc01060 Promote Progression of Glioma by Regulating the Mzf1/C-Myc/Hif1alpha Axis. Cancer Res (2021) 81(1):114–28. doi: 10.1158/0008-5472.CAN-20-2270
94. Colwell N, Larion M, Giles AJ, Seldomridge AN, Sizdahkhani S, Gilbert MR, et al. Hypoxia in the Glioblastoma Microenvironment: Shaping the Phenotype of Cancer Stem-Like Cells. Neuro Oncol (2017) 19(7):887–96. doi: 10.1093/neuonc/now258
95. Lim M, Xia Y, Bettegowda C, Weller M. Current State of Immunotherapy for Glioblastoma. Nat Rev Clin Oncol (2018) 15(7):422–42. doi: 10.1038/s41571-018-0003-5
96. Hambardzumyan D, Gutmann DH, Kettenmann H. The Role of Microglia and Macrophages in Glioma Maintenance and Progression. Nat Neurosci (2016) 19(1):20–7. doi: 10.1038/nn.4185
97. Chaligne R, Gaiti F, Silverbush D, Schiffman JS, Weisman HR, Kluegel L, et al. Epigenetic Encoding, Heritability and Plasticity of Glioma Transcriptional Cell States. Nat Genet (2021) 53(10):1469–79. doi: 10.1038/s41588-021-00927-7
98. Nicholson JG, Fine HA. Diffuse Glioma Heterogeneity and Its Therapeutic Implications. Cancer Discov (2021) 11(3):575–90. doi: 10.1158/2159-8290.CD-20-1474
99. van Tellingen O, Yetkin-Arik B, de Gooijer MC, Wesseling P, Wurdinger T, de Vries HE. Overcoming the Blood-Brain Tumor Barrier for Effective Glioblastoma Treatment. Drug Resist Update (2015) 19:1–12. doi: 10.1016/j.drup.2015.02.002
100. Zhou W, Chen C, Shi Y, Wu Q, Gimple RC, Fang X, et al. Targeting Glioma Stem Cell-Derived Pericytes Disrupts the Blood-Tumor Barrier and Improves Chemotherapeutic Efficacy. Cell Stem Cell (2017) 21(5):591–603.e4. doi: 10.1016/j.stem.2017.10.002
101. De Boeck A, Ahn BY, D'Mello C, Lun X, Menon SV, Alshehri MM, et al. Glioma-Derived IL-33 Orchestrates an Inflammatory Brain Tumor Microenvironment That Accelerates Glioma Progression. Nat Commun (2020) 11(1):4997. doi: 10.1038/s41467-020-18569-4
102. Wang Q, Hu B, Hu X, Kim H, Squatrito M, Scarpace L, et al. Tumor Evolution of Glioma-Intrinsic Gene Expression Subtypes Associates With Immunological Changes in the Microenvironment. Cancer Cell (2017) 32(1):42–56.e6. doi: 10.1016/j.ccell.2017.06.003
103. Wang H, Xu T, Huang Q, Jin W, Chen J. Immunotherapy for Malignant Glioma: Current Status and Future Directions. Trends Pharmacol Sci (2020) 41(2):123–38. doi: 10.1016/j.tips.2019.12.003
104. Iwami K, Natsume A, Wakabayashi T. Cytokine Networks in Glioma. Neurosurg Rev (2011) 34(3):253–63. doi: 10.1007/s10143-011-0320-y
105. Tran TT, Uhl M, Ma JY, Janssen L, Sriram V, Aulwurm S, et al. Inhibiting Tgf-Beta Signaling Restores Immune Surveillance in the Sma-560 Glioma Model. Neuro Oncol (2007) 9(3):259–70. doi: 10.1215/15228517-2007-010
106. Prionisti I, Buhler LH, Walker PR, Jolivet RB. Harnessing Microglia and Macrophages for the Treatment of Glioblastoma. Front Pharmacol (2019) 10:506. doi: 10.3389/fphar.2019.00506
107. Mathios D, Kim JE, Mangraviti A, Phallen J, Park CK, Jackson CM, et al. Anti-Pd-1 Antitumor Immunity Is Enhanced by Local and Abrogated by Systemic Chemotherapy in Gbm. Sci Transl Med (2016) 8(370):370ra180. doi: 10.1126/scitranslmed.aag2942
108. Klionsky DJ, Abdel-Aziz AK, Abdelfatah S, Abdellatif M, Abdoli A, Abel S, et al. Guidelines for the Use and Interpretation of Assays for Monitoring Autophagy (4th Edition)(1). Autophagy (2021) 17(1):1–382. doi: 10.1080/15548627.2020.1797280
109. Yang WS, SriRamaratnam R, Welsch ME, Shimada K, Skouta R, Viswanathan VS, et al. Regulation of Ferroptotic Cancer Cell Death by Gpx4. Cell (2014) 156(1-2):317–31. doi: 10.1016/j.cell.2013.12.010
110. Li Y, Cao Y, Xiao J, Shang J, Tan Q, Ping F, et al. Inhibitor of Apoptosis-Stimulating Protein of P53 Inhibits Ferroptosis and Alleviates Intestinal Ischemia/Reperfusion-Induced Acute Lung Injury. Cell Death Differ (2020) 27(9):2635–50. doi: 10.1038/s41418-020-0528-x
111. Fang Y, Chen X, Tan Q, Zhou H, Xu J, Gu Q. Inhibiting Ferroptosis Through Disrupting the Ncoa4-Fth1 Interaction: A New Mechanism of Action. ACS Cent Sci (2021) 7(6):980–9. doi: 10.1021/acscentsci.0c01592
112. Liu T, Zhu C, Chen X, Guan G, Zou C, Shen S, et al. Ferroptosis, as the Most Enriched Programmed Cell Death Process in Glioma, Induces Immunosuppression and Immunotherapy Resistance. Neuro Oncol (2022) 11:noac033. doi: 10.1093/neuonc/noac033
113. Liu Y, Fang Y, Zhang Z, Luo Y, Zhang A, Lenahan C, et al. Ferroptosis: An Emerging Therapeutic Target in Stroke. J Neurochem (2022) 160(1):64–73. doi: 10.1111/jnc.15351
114. Zhao H, Ji B, Chen J, Huang Q, Lu X. Gpx 4 Is Involved in the Proliferation, Migration and Apoptosis of Glioma Cells. Pathol Res Pract (2017) 213(6):626–33. doi: 10.1016/j.prp.2017.04.025
115. Wang W, Green M, Choi JE, Gijon M, Kennedy PD, Johnson JK, et al. Cd8(+) T Cells Regulate Tumour Ferroptosis During Cancer Immunotherapy. Nature (2019) 569(7755):270–4. doi: 10.1038/s41586-019-1170-y
Keywords: glioma, ferroptosis, immune microenvironment, immunotherapy, GPX4
Citation: Wang K, Wang J, Zhang J, Zhang A, Liu Y, Zhou J, Wang X and Zhang J (2022) Ferroptosis in Glioma Immune Microenvironment: Opportunity and Challenge. Front. Oncol. 12:917634. doi: 10.3389/fonc.2022.917634
Received: 11 April 2022; Accepted: 13 May 2022;
Published: 27 June 2022.
Edited by:
Eduard Yakubov, Paracelsus Medical Private University, GermanyReviewed by:
Aayushi Mahajan, Columbia University, United StatesFlavia Biamonte, Magna Græcia University of Catanzaro, Italy
Copyright © 2022 Wang, Wang, Zhang, Zhang, Liu, Zhou, Wang and Zhang. This is an open-access article distributed under the terms of the Creative Commons Attribution License (CC BY). The use, distribution or reproduction in other forums is permitted, provided the original author(s) and the copyright owner(s) are credited and that the original publication in this journal is cited, in accordance with accepted academic practice. No use, distribution or reproduction is permitted which does not comply with these terms.
*Correspondence: Xiaoyu Wang, wxy95@zju.edu.cn; Jianmin Zhang, zjm135@zju.edu.cn
†These authors have contributed equally to this work