- Institute for Theoretical Biology, Humboldt-University Berlin, Berlin, Germany
Caffeine is by far the most ubiquitous psychostimulant worldwide found in tea, coffee, cocoa, energy drinks, and many other beverages and food. Caffeine is almost exclusively metabolized in the liver by the cytochrome P-450 enzyme system to the main product paraxanthine and the additional products theobromine and theophylline. Besides its stimulating properties, two important applications of caffeine are metabolic phenotyping of cytochrome P450 1A2 (CYP1A2) and liver function testing. An open challenge in this context is to identify underlying causes of the large inter-individual variability in caffeine pharmacokinetics. Data is urgently needed to understand and quantify confounding factors such as lifestyle (e.g., smoking), the effects of drug-caffeine interactions (e.g., medication metabolized via CYP1A2), and the effect of disease. Here we report the first integrative and systematic analysis of data on caffeine pharmacokinetics from 141 publications and provide a comprehensive high-quality data set on the pharmacokinetics of caffeine, caffeine metabolites, and their metabolic ratios in human adults. The data set is enriched by meta-data on the characteristics of studied patient cohorts and subjects (e.g., age, body weight, smoking status, health status), the applied interventions (e.g., dosing, substance, route of application), measured pharmacokinetic time-courses, and pharmacokinetic parameters (e.g., clearance, half-life, area under the curve). We demonstrate via multiple applications how the data set can be used to solidify existing knowledge and gain new insights relevant for metabolic phenotyping and liver function testing based on caffeine. Specifically, we analyzed 1) the alteration of caffeine pharmacokinetics with smoking and use of oral contraceptives; 2) drug-drug interactions with caffeine as possible confounding factors of caffeine pharmacokinetics or source of adverse effects; 3) alteration of caffeine pharmacokinetics in disease; and 4) the applicability of caffeine as a salivary test substance by comparison of plasma and saliva data. In conclusion, our data set and analyses provide important resources which could enable more accurate caffeine-based metabolic phenotyping and liver function testing.
1 Introduction
Caffeine is commonly found in tea, coffee, cocoa, energy drinks, and many other beverages. It is by far the most ubiquitous psychostimulant worldwide (Gilbert, 1984), with 85% of the United States population consuming caffeine daily (Mitchell et al., 2014). Among caffeine consumers, the average consumption is more than 200 mg of caffeine per day (Frary et al., 2005). Caffeine is mainly known for its stimulating properties but is also consumed for improved exercise performance and the treatment of various diseases (e.g., apnea in prematurity, hypersomnia). Two important applications of caffeine are liver function testing and metabolic phenotyping of cytochrome P450 1A2 (CYP1A2), N-acetyltransferase 2 (NA2), and xanthine oxidase (XO) (Wang et al., 1985; Jost et al., 1987; Wahlländer et al., 1990; Tripathi et al., 2015).
Caffeine is almost exclusively metabolized in the liver by the cytochrome P450 enzyme system with 3% or less being excreted unchanged in urine (Kot and Daniel, 2008). In humans, N-3 demethylation of caffeine (1,3,7-trimethylxanthine) to paraxanthine (1,7-dimethylxanthine) is the main reaction in the metabolism of caffeine, accounting for around 80–90% of caffeine demethylation. The reaction is exclusively mediated by the activity of the cytochrome P450 isoform 1A2 (CYP1A2) (Hakooz, 2009). The remainder of caffeine is metabolized to around 11 and 4% to the 1-demethylated product theobromine and 7-demethylated product theophylline, respectively (Lelo et al., 1986b; Kalow and Tang, 1993; Miners and Birkett, 1996; Amchin et al., 1999).
Large variation exists in the consumption of caffeine-containing beverages and food between individuals, which can induce CYP1A2 activity. In addition, CYP1A2 activity and protein amount are affected by environmental, genetic, and epigenetic factors (Klein et al., 2010) resulting in large variation between 5-6 fold in humans (Schrenk et al., 1998). These factors lead to a wide range of caffeine plasma concentrations and caffeine pharmacokinetics.
Sex does not significantly influence the CYP1A2 activity (Klein et al., 2010; Yang et al., 2010; Puri et al., 2020). A large heritability of CYP1A2 activity could be shown by two twin studies (Rasmussen et al., 2002; Matthaei et al., 2016). With excluded users of hormonal contraceptives and smokers, 89% of the variation in caffeine AUC was shown to be due to genetic effects and 8% specifically due to the CYP1A1/1A2 promoter polymorphism. In other studies, statistically significant genetic or epigenetic markers on the CYP1A locus on chromosome 15 could not be found (Moonen et al., 2004; Jiang et al., 2006; Ghotbi et al., 2007; Gunes and Dahl, 2008; Myrand et al., 2008; Klein et al., 2010; Yang et al., 2010). Genes regulating the expression and function of CYP1A2 and non-genetic factors could explain 42, 38, and 33% of CYP1A2 variation at activity, protein, and mRNA level, respectively (Klein et al., 2010). Lifestyle factors (e.g., smoking) and use of oral contraceptives have been shown to influence caffeine pharmacokinetics, as have pregnancy, obesity, alcohol consumption, and the coadministrations of drugs (e.g., fluvoxamine and pipmedic acid). Many diseases reduce the metabolic capabilities of patients. For caffeine which is predominantly metabolized by the liver, various liver diseases result in a strong reduction in caffeine clearance. The most profound reduction is observed in cirrhotic liver disease, correlating with the degree of hepatic impairment (Holstege et al., 1989; Park et al., 2003; Jodynis-Liebert et al., 2004; Tripathi et al., 2015).
Metabolic phenotyping of enzymes by probe drugs is a common method to evaluate the impact of lifestyle, drug-gene and drug-drug interactions, and other factors influencing enzyme activity. Caffeine is an established probe drug for CYP1A2, N-acetyltransferase 2 (NAT2), and xanthine oxidase (XO) metabolic activities (Fuhr et al., 1996; Miners and Birkett, 1996; Faber et al., 2005; Hakooz, 2009). It is rapidly and completely absorbed by the gastrointestinal tract, distributed throughout the total body water, has low plasma binding, as well as short half-life, negligible first-pass metabolism (Yesair et al., 1984), minimal renal elimination, excellent tolerability, and its biotransformation is virtually confined to the liver (Kalow and Tang, 1993; Amchin et al., 1999; Drozdzik et al., 2018). Caffeine is especially used for CYP1A2 phenotyping which contributes 5–20% to the hepatic P450 pool and is involved in the clearance of about 9% of clinically used drugs (Zanger and Schwab, 2013). The partial or systemic caffeine clearance measured in plasma is considered to be the gold standard for CYP1A2 phenotyping (Fuhr et al., 1996) since 95% of the systemic clearance of caffeine is estimated to be due to hepatic CYP1A2 (Amchin et al., 1999). Measurements in serum, saliva, and urine are extensively studied as well. In urine, sampling at multiple time points and precise timing are inherently difficult. Thus, clearance rates are typically calculated only from plasma, saliva, and serum samples. Measurements in saliva are not invasive and show good correlation with measurements in plasma (Callahan et al., 1982; Wahlländer et al., 1990). The metabolic ratio (MR) between various metabolites of caffeine is an established alternative measure for CYP1A2 enzyme activity (Hakooz, 2009). Analogously, the MR is measured in any of the above mentioned tissues though typically only at a single time point after drug administration. The MR of various metabolites at 4 h after dosing in plasma, saliva, and urine correlate well with the apparent caffeine clearance, 0.84, 0.82, 0.61, respectively (Carrillo et al., 2000). The MRs measured in plasma and urine have been historically most popular. However, measurements in saliva are routinely applied, especially in epidemiological studies (Tantcheva-Poór et al., 1999; Kukongviriyapan et al., 2004; Tripathi et al., 2015; Chia et al., 2016; Urry et al., 2016; Puri et al., 2020).
Despite the great potential of caffeine as a test substance for liver function tests and CYP1A2 based phenotyping, so far caffeine testing has not found widespread clinical adoption. For liver function tests, a major limiting factor is the large inter-individual variability. Data is urgently needed to understand and quantify confounding factors of caffeine pharmacokinetics such as lifestyle (e.g., smoking) and the effects of drug-drug interactions (e.g., drugs metabolized via CYP1A2) or how disease alters caffeine elimination. Based on such data, more accurate liver function tests and CYP1A2 phenotyping protocols could be established. Differences in clinical protocols (e.g., dosing amount, sampling tissue, and timing) have not been systematically analyzed in the literature. In addition, data on competing substances in the context of dynamical liver function tests (e.g., metacethin used in the LiMAx test (Rubin et al., 2017)) and CYP1A2 phenotyping is not accessible but absolutely imperative for a quantitative evaluation of these methods.
Caffeine pharmacokinetics have been investigated in a multitude of clinical trials, each with its own focus and research question. These studies have been reviewed in a broad scope, most recently in (Arnaud, 2011; Nehlig, 2018). Despite caffeine pharmacokinetics being highly studied in literature, no integrated pharmacokinetics data set exists so far and no systematic analysis of the reported data has been performed. The objective of this work was to fill this gap by providing the first comprehensive high-quality data set of reported data on caffeine pharmacokinetics and demonstrate its value via multiple example applications relevant for metabolic phenotyping and liver function testing based on caffeine.
2 Materials and Methods
This study is guided by the Preferred Reporting Items for Systematic reviews and Meta-Analysis (PRISMA) statement and its extension for Scoping Reviews (PRISMA-ScR) (Liberati et al., 2009; Tricco et al., 2018). This work was conducted with the aim to answer the following research questions. 1) What is the current state of research on caffeine pharmacokinetics in adult humans in the context of metabolic phenotyping and liver function testing? 2) How homogeneous is the reporting? 3) How do caffeine dose and route impact caffeine pharmacokinetics? 4) What is the effect of smoking and oral contraceptive use on caffeine pharmacokinetics with respect to the caffeine dose? 5) What is the effect of coadministrations on caffeine pharmacokinetics with respect to the dosing amount and what are the effect sizes of caffeine-drug interactions? 6) What is the effect of diseases on caffeine pharmacokinetics with respect to the caffeine dose and what are the effect sizes of caffeine-disease interactions? 7) How do sampling time and tissue influence pharmacokinetics and phenotyping? The search, screening and filtering process are depicted in the PRISMA flow diagram in Figure 1.
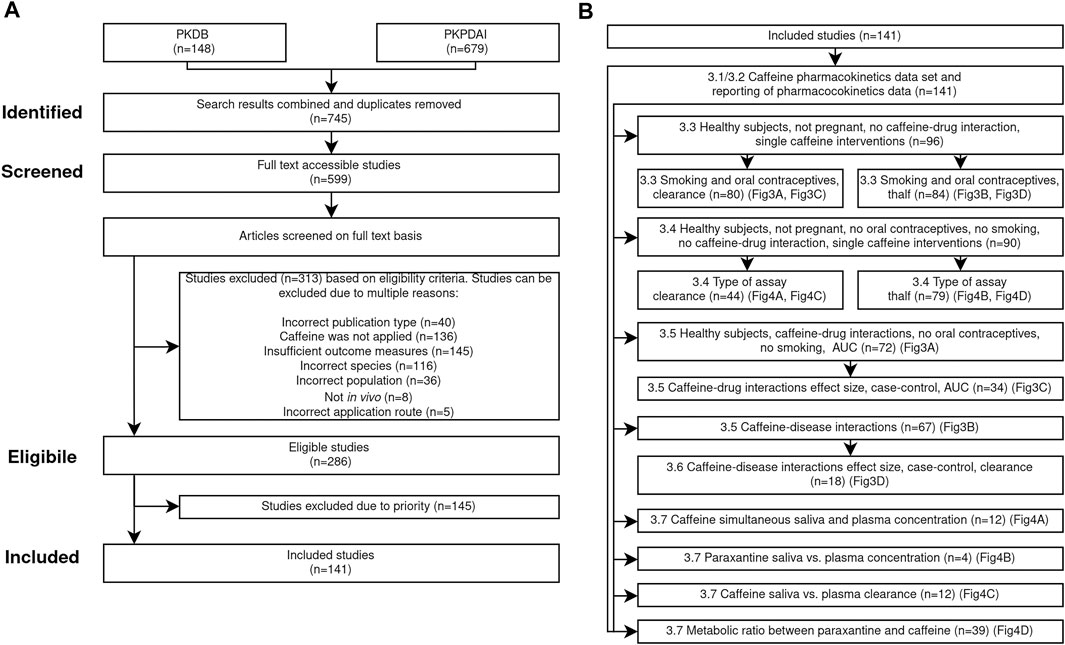
FIGURE 1. PRISMA flow diagram. (A) Overview of search strategy and inclusion/exclusion criteria applied in the systematic analysis of pharmacokinetics of caffeine. The applied workflow resulted in n = 141 included studies. (B) Subsets of the included studies were used for the various analyses. For details see the Section 2.
2.1 Search Strategy
We searched the general purpose pharmacokinetics database PK-DB with the search query https://pk-db.com/api/v1/filter/?concise=false&download=true&interventions__substance=caffeine on 2021–10–06 and PKPDAI (Hernandez et al., 2021) with the search query https://app.pkpdai.com/?term=caffeine on 2021–10–06. PKPDAI is a dedicated search engine for pharmacokinetics data based on PubMed. This search resulted in 745 studies from which 599 could be accessed as full text.
2.2 Eligibility Criteria
Studies were included or excluded based on the following eligibility criteria. Reviews or publications describing computational models were excluded. For a study to be eligible caffeine must be applied, it must report pharmacokinetics data for caffeine or its metabolites, data must be measured in humans, and individuals and groups must consisted of adults (age
2.3 Data Curation
Pharmacokinetics data was curated manually as part of the pharmacokinetics database PK-DB (https://pk-db.com/) (Grzegorzewski et al., 2020) using established workflows. The pharmacokinetics data was stored in combination with relevant metadata on groups, individuals, interventions, and outputs. PK-DB provided support in the curation process with strong validation rules, unit normalization, and automatic calculation of pharmacokinetic parameters from time-courses. As part of the curation process and the presented analyses, pharmacokinetic parameters and other commonly reported measurements were integrated from multiple studies. The meta-analyses and data integration allowed to identify and correct/remove outlier data which were mostly due to either curation errors or incorrect reporting. Pharmacokinetic parameters calculated from time-courses are included in the analyses. For more details see (Grzegorzewski et al., 2020).
2.4 Data Processing and Filtering
In Figures 3A,B, Figures 5A–D, and Figure 4 the data is displayed in a similar manner. For collectively reported subjects, the group size and standard error is displayed as the marker size and error-bar, respectively. In the legend, (I), (G), and (TI) stand for individual participant data, the number of groups, and the total number of subjects, respectively. Data points are depicted as circles if reported equivalently in the source, as squares if calculated from concentration-time profiles and as triangles if inferred from corresponding pharmacokinetic data and body weights of the subjects. Typically, dosing is reported in mass units, AUC in mass per volume units, clearance in volume per time units, and half-life in time units. Occasionally, dosage, AUC, and clearance are reported in body weight units. In case of reported subject weight, the data is harmonized to similar units by multiplying with the reported weights.
In Figure 3, the depicted subjects were healthy. Male subjects were assumed to not take any oral contraceptives. Substances with negligible caffeine-drug interactions were determined by an effect size analysis in Section 3.5. All other co-administrations and investigations containing multiple caffeine dosages were excluded.
In subplot Figure 5A, included subjects were healthy. The area under the caffeine concentration curves measured at least up to 12 h after a single application of caffeine and AUC extrapolated to infinity were included. Multiple subsequent caffeine dosages were excluded, other administrations and co-administrations included. In subplot Figure 5C, healthy and non-healthy subjects were included. Single applications of caffeine or caffeine administrated as a cocktail with negligible drug-drug interactions were included. All other co-administrations and investigations containing multiple caffeine dosages were excluded.
In subplots Figures 6A–C, no data was excluded. In subplot Figure 6D and Figure 4, included subjects were healthy, non-smoking, non-pregnant, and non-oral contraceptive consumers. Interventions with caffeine administrated as a cocktail with negligible caffeine-drug interactions were included. All other co-administrations and investigations containing multiple caffeine dosages were excluded.
3 Results
3.1 Caffeine Pharmacokinetics Data Set
Within this work, the first comprehensive open pharmacokinetics data set on caffeine was established. The data set integrates data from 141 publications (Figure 2 and Table 1), with most of the publications corresponding to a distinct clinical trial. Studies were identified and included or excluded following the systematic approach described in the PRISMA flow diagram (Figure 1A). The focus of data curation was on pharmacokinetics data of caffeine, caffeine metabolites, and caffeine metabolic ratios in human adults. Importantly, the data set is enriched with meta-data on 1) the characteristics of studied patient cohorts and subjects (e.g., age, body weight, smoking status, health status, fasting); 2) the applied interventions (e.g., dosing, substance, route of application); 3) measured pharmacokinetic time-courses; and 4) pharmacokinetic parameters (e.g., clearance, half-life, area under the curve). In summary, data from 500 groups and 4,714 individuals is reported under 387 interventions resulting in 24 ,571 pharmacokinetic outputs and 846 time-courses. The data set is available via the pharmacokinetics database PK-DB (https://pk-db.com/) with a detailed description of the data structure provided in (Grzegorzewski et al., 2020). We demonstrate the value of the data set by its application to multiple research questions relevant for metabolic phenotyping and liver function testing (Figure 1B): 1) the effect of smoking and oral contraceptive use on caffeine elimination (Section 3.3); 2) the effect of the type of assay (Section 3.4); 3) the interaction of caffeine with other drugs (Section 3.5), 4) alteration of caffeine pharmacokinetics in disease (Section 3.6); and 5) the applicability of caffeine as a salivary test substance by comparison of plasma and saliva data (Section 3.7). In the following, we summarize the quality of reporting and provide example applications of the data set.
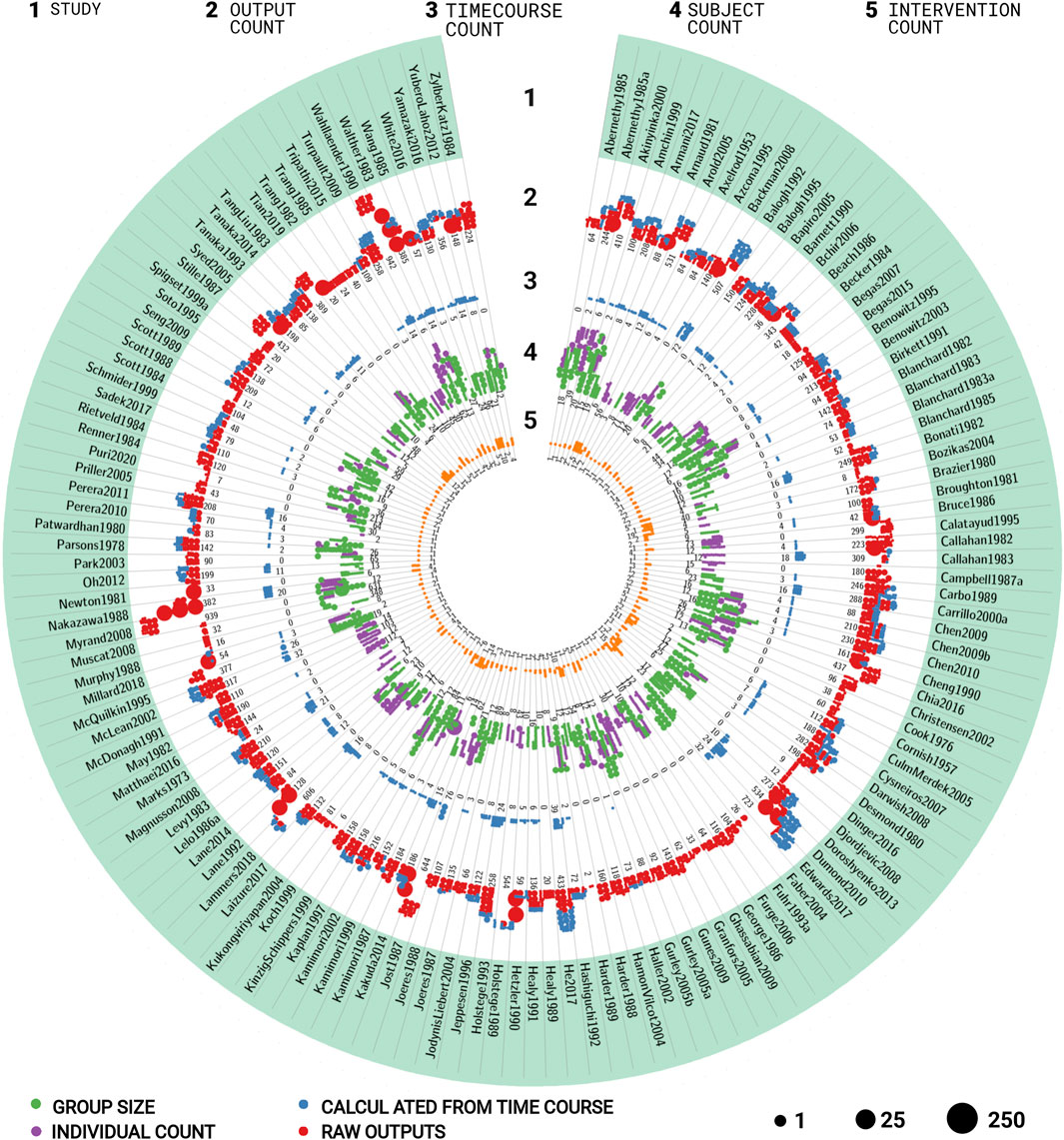
FIGURE 2. Overview of studies in the caffeine pharmacokinetics data set. The data set consists of 141 studies containing 500 groups, 4,714 individuals, 387 interventions, 24 ,571 outputs, and 846 time-courses. The circular plot is structured in stripes and rings. Each stripe represents a different study, each ring the amount of different data types for the respective study. The dots represent the respective amount of data with the dot size corresponding to the number of entries per dot. The rings contain the following information for the respective study (A) name of the study; (B) number of outputs (pharmacokinetics parameters and other measurements). Red dots represent reported data, blue dots data calculated from time-courses reported in the study; (C) number of time-courses; (D) number of participants. Purple dots represent participants with individual data, green dots represent collectively reported participants; (E) number of interventions applied to the participants in the study. For additional information see Table 1.
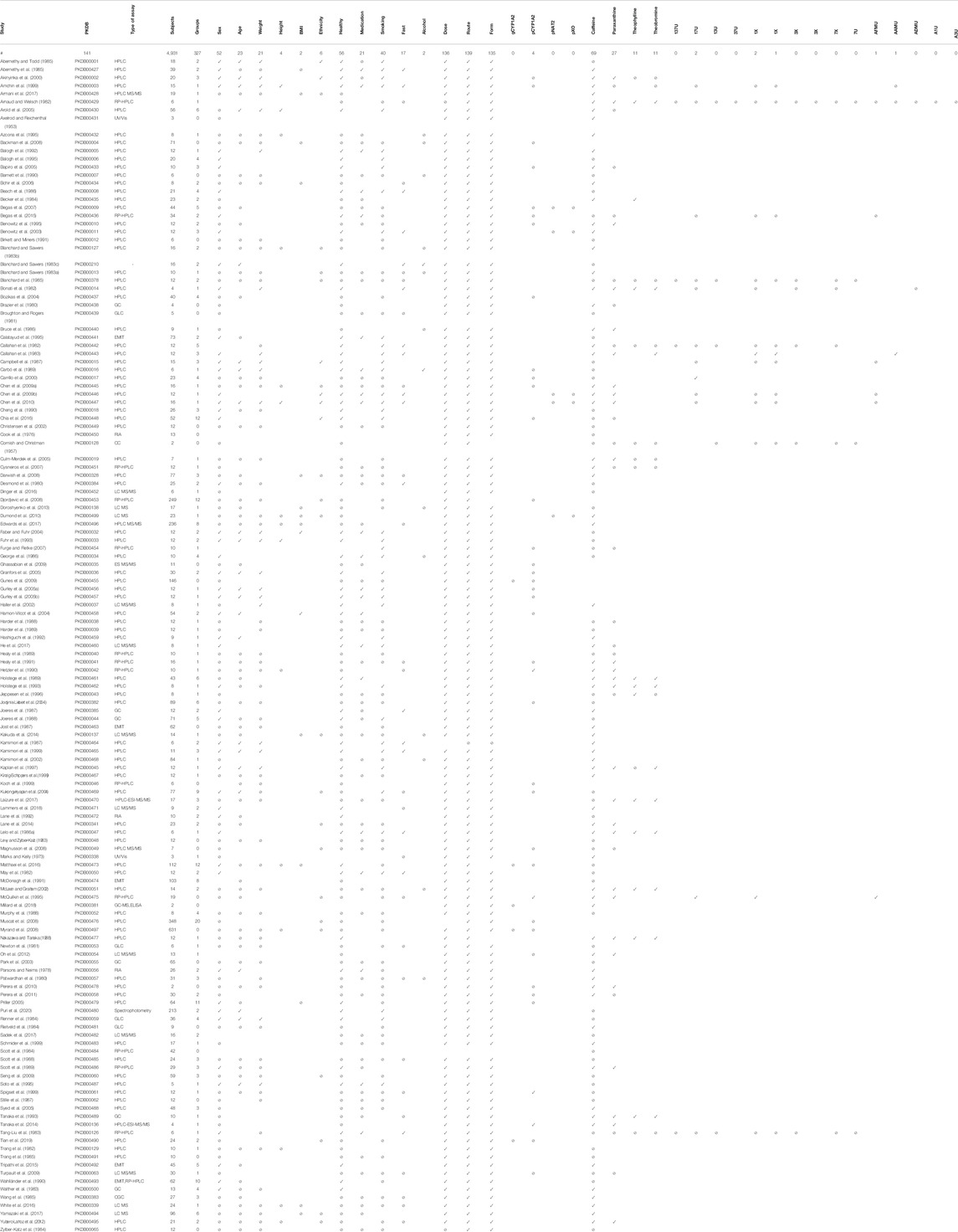
TABLE 1. Overview of curated studies. For each study the table shows which information was reported. Either, information was reported (✓), partially reported (⊘) or not reported at all (whitespace). The type of assay column contains reported information on quantification method for the concentration of caffeine or its metabolites (i.e., CC: Column chromatography, GC: Gas chromatography, CGC: Capillary gas chromatography, GLC: Gas-liquid chromatography, GC MS: Gas chromatography mass spectrometry, HPLC: High performance liquid chromatography, HPLC MS/MS: High performance liquid chromatography-tandem mass spectrometry, RP-HPLC: Reversed-phase high-performance liquid chromatography, LC MS Liquid chromatography mass spectrometry, LC MS/MS Liquid chromatography-tandem mass spectrometry, HPLC-ESI-MS/MS: High-performance liquid chromatography-electrospray ionisation tandem mass spectrometry, ES MS/MS: Electrospray ionisation tandem mass spectrometry, UV/Vis: UV–visible spectrophotometry, EMIT: Enzyme multiplied immunoassay technique, ELISA: enzyme-linked immunosorbent assay, RIA: Radioimmunoassay).
3.2 Reporting of Pharmacokinetics Data
The study design as well as quality and details of reporting of results were very heterogeneous between studies. Major differences exist in the study design, number of study participants, and number of reported time-courses. Many studies report some individual participants data (84/141) but only a minority of the studies report individual participant data for all study participants (6/84) (Axelrod and Reichenthal, 1953; Brazier et al., 1980; Rietveld et al., 1984; McQuilkin et al., 1995; Perera et al., 2010; Millard et al., 2018). Many studies report only aggregated data on group level (57/141). In most studies, the application of a single dose of caffeine was studied (129/141). In the case of multiple interventions (49/141), mostly one additional substance was co-administrated (33/141). The main categories of studies were either 1) case-control studies which compare caffeine pharmacokinetics in two groups (e.g., healthy vs Disease) (64/141), 2) crossover studies on caffeine-drug interactions (comparing caffeine alone vs caffeine and additional substance) (33/141) 3) studies on metabolic phenotypes (including drug cocktails) (42/141); or 4) methodological studies (e.g., establishing mass spectrometry protocol for quantification or new site of sampling).
Intervention protocols, i.e., the applied substances, form, dose, and timing of application was typically reported in good detail. In crossover studies, the difference between treatments was generally reported in good detail. For the dosing with caffeine, the amount (136/141), route (e.g., oral, intravenous) (140/141), form (tablet, capsule, solution) (136/141), and the substance (141/141) are typically reported. Co-administrations of medication and other substances are often mentioned qualitatively (28/49), skipping either the amount, route, form, or exact timing of application.
The quantification protocol, i.e., type of assay (e.g., high-performance liquid chromatography, gas-liquid chromatography) (140/141), the site of sampling (e.g., plasma, serum, saliva, urine) (141/141), and the time points when samples were taken (128/141), were mostly reported in good detail. However, in some studies the protocol is not mentioned explicitly but only via additional references, which complicates the curation. For a single study no information on the quantification method could be accessed (Blanchard and Sawers, 1983c). The reporting of type of assay is very heterogeneous with many studies not providing sufficient details (e.g., HPLC is often mentioned as the separation technique but the subsequent detection or quantification method is not stated).
The information on subject characteristics was less often reported in sufficient detail with large differences in the quality and quantity of reporting between studies. Any information on sex (131/141), weight (71/141), and age (89/141) was relatively often reported on group or individual level. However, age and weight were rarely reported on an individual level and often not even for all groups. Other anthropometric factors such as height (15/141), body mass index (BMI) (15/141), and ethnicity (25/141) are rarely reported. The genotype of CYP1A2 (gCYP1A2) is rarely measured or reported (5/141), even though there is evidence that genetic variation can play an important role in caffeine metabolism. Further, the nomenclature is not standardized. It is worth noting that low-cost genotyping methods were not available for early studies included in the data set. The phenotype (pCYP1A2, pXO, pNAT2) of enzymes involved in the metabolism of caffeine, i.e., CYP1A2, xanthine oxidase (XO), or N-acetyltransferase type 2 (NAT2) were investigated occasionally (38/141). The information on other factors influencing the pharmacokinetics of caffeine is reported very heterogeneously. The strong influence of smoking (105/141) and the use of oral contraceptives (42/141) on the enzyme activity of CYP1A2 and thereby on the apparent clearance of caffeine is covered relatively well in many publications. Health status and patient diseases are often covered (134/141). However, often categorized in broad and general disease classes, with more specific disease classification lacking. Markers related to cardiovascular health (e.g., blood pressure, cholesterol level, bilirubin level) are basically not reported in the context of caffeine pharmacokinetics. In case of cirrhosis, further information of severity is reported sparsely. Important information on the abstinence of caffeine or methylxanthines and consumption of caffeine or other caffeine-containing beverages is often missing.
Individual-level reporting is essential for subsequent pharmacokinetic modeling, as biological mechanisms responsible for the pharmacokinetics strongly correlate with these factors and large inter-individual variability exists in caffeine pharmacokinetics. Despite the importance of individual subject data, information on individuals is rarely provided.
Most studies report pharmacokinetics outputs on caffeine (126/141). Data on the main product paraxanthine (46/141) and secondary metabolites theobromine (20/141) and theophylline (19/141) are reported sometimes. Additional metabolites such as 137U, 17U, 13U, 37U, 1X, 1U, 3X, 3U, 7X, 7U, AFMU, AAMU, ADMU, A1U, and A3U are seldom reported, mostly as urinary measurements and not directly but as part of a metabolic ratio.
3.3 Smoking and Oral Contraceptives
In a first analysis, we were interested in the effect of smoking and oral contraceptive use on the pharmacokinetics of caffeine in healthy subjects (see Figure 3). Both have repeatedly been reported as key exogenous factors affecting caffeine elimination. A main question was how reproducible the effect is and if by integrating data from multiple studies a more consistent picture of the effects can be gained. For the analysis the data set was stratified into smokers, oral contraceptive users and a control group (neither smoking nor using oral contraceptives). Smoking results in increased caffeine clearance (Figures 3A,C) and decreased half-life of caffeine elimination (Figures 3B,D) whereas oral contraceptive use has the opposite effect over a wide dose range of caffeine. An important result from our analysis is that a consistent and reproducible effect can be found over more than 50 years of pharmacokinetic research. With exception of a few outlier studies probably from a single clinical trial (see methods) all data was highly consistent. This provides a strong argument for the applied methods and protocols.
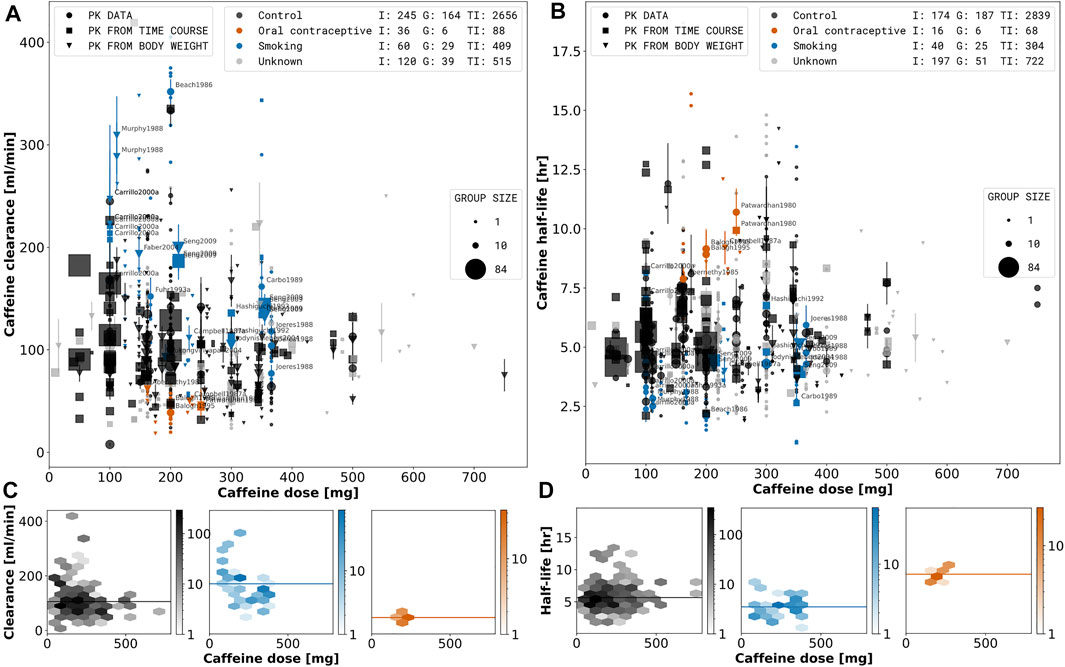
FIGURE 3. Dose-dependent effect of smoking and oral contraceptive use on caffeine pharmacokinetics. A stratified meta-analysis of caffeine clearance (A) and half-life (B) depending on reported smoking and oral contraceptive use and dose was performed. Black: Control subjects are non-smokers and not taking oral contraceptives; Orange: Oral contraceptive users independent of smoking status (smokers and non-smokers). Blue: Smoking are smokers not consuming oral contraceptives. Grey: Unknown data correspond to subjects with unreported smoking and oral contraceptive status. Marker shape, and size describe the datatype and group size, respectively. Data representing smokers or oral contraceptive consumers is labeled by the respective study name. The hexagonal bin plots in the lower panel (C, D) correspond to the subset of data for the control, smoking, and oral contraceptive consuming subjects. The color intensity of each bin represents the number of subjects falling in a given hexagonal bin area. Data selection criteria and visualization are described in the Section 2.
Despite the large effect of smoking and oral contraceptive use on the pharmacokinetics of caffeine, the information is only reported for a subset of studies. Smoking as well as oral contraceptive use should be an exclusion criteria for subjects in studies of caffeine pharmacokinetics, due to the possible confounding effects. Importantly, in many groups smokers and non-smokers were mixed without reporting data for smokers and non-smokers separately. Without reporting of data on individuals or subgroups no stratification could be performed, which could strongly affect results if not balanced between groups. In summary, the integrative data analysis showed a consistent strong activating effect of smoking on caffeine elimination and an inhibiting effect of oral contraceptive use on caffeine elimination.
3.4 Effect of Type of Assay
The reported type of assays were curated systematically (see Table 1 to study effects of the type of technique and assay on the reported pharmacokinetics data (see Figure 4, abbreviations explained in the legend of Table 1). Data affected by confounding factors (i.e., smoking, oral contraceptive use, caffeine-disease, and caffeine-disease interactions) was excluded. Based on our analysis no systematic difference between the reported type of assays could be detected. Immunoassays (RIA, EMIT, ELISA), mass spectrometry (LC MS, GC MS, LC MS/MS, HPLC MS/MS, HPLC-ESI-MS/MS, ES MS/MS), and chromatography with unspecified quantification method (HPLC, RP-HPLC, GLC, CGC, CC, GC) do not show any differences in half-life or clearance. Spectrophotometric methods (UV/Vis) are generally rare and were not applied in this data subset. Based on the analysis we conclude that no systematic correction was required to compare the data collected with the different types of assays.
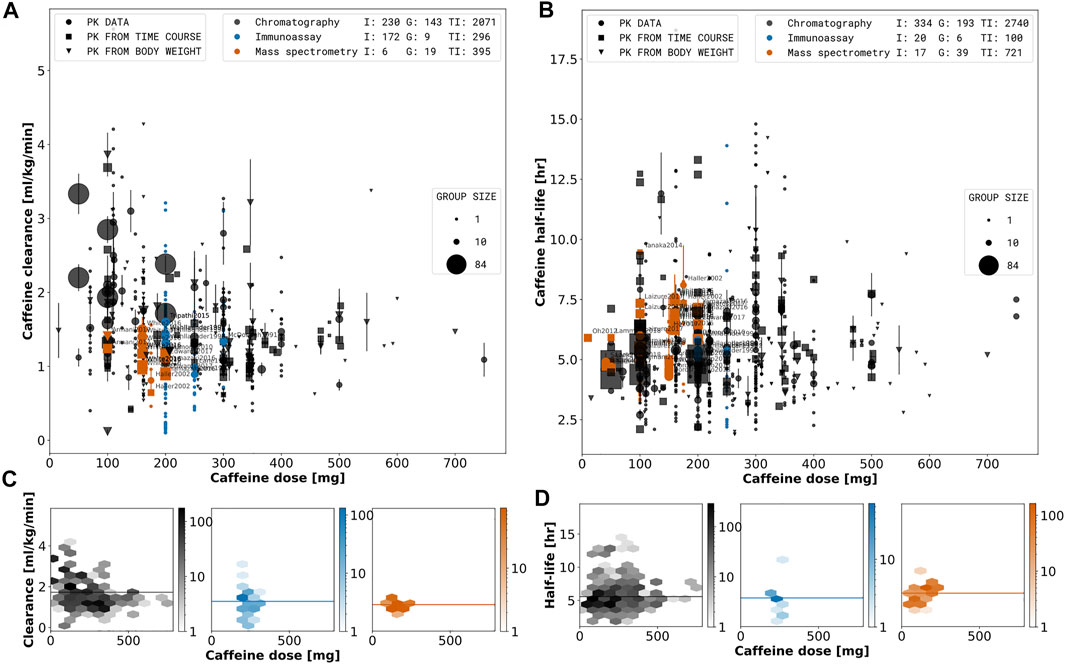
FIGURE 4. Effect of type of assay on pharmacokinetic parameters. A stratified meta-analysis of caffeine clearance (A) and half-life (B) depending on reported assay type and dose. Orange: Measurements performed with mass spectrometry (LC MS, LC MS/MS, HPLC MS/MS, HPLC-ESI-MS/MS, ES MS/MS). Blue: Measurements performed with immunoassay (RIA, EMIT). Black: Separation performed with chromatography but quantification assay was not reported (HPLC, RP-HPLC, GLC, CGC, CC, GC). Marker shape, and size describe the datatype and group size, respectively. Data with reported quantification method are labeled by the respective study name. The hexagonal bin plots in the lower panel (C, D) correspond to the subset of data for the mass spectrometry quantification, immunoassay quantification and chromatography. The color intensity of each bin represents the number of subjects falling in a given hexagonal bin area. Data selection criteria and visualization are described in the Section 2.
3.5 Caffeine-Drug Interactions
An important question for metabolic phenotyping and liver function testing with caffeine is how the coadministration of other drugs and compounds affects caffeine clearance. Consequently, we studied in the second analysis the reported caffeine-drug interactions in the data set. The impact of drugs was quantified using the change in AUC between a coadministration and a respective control
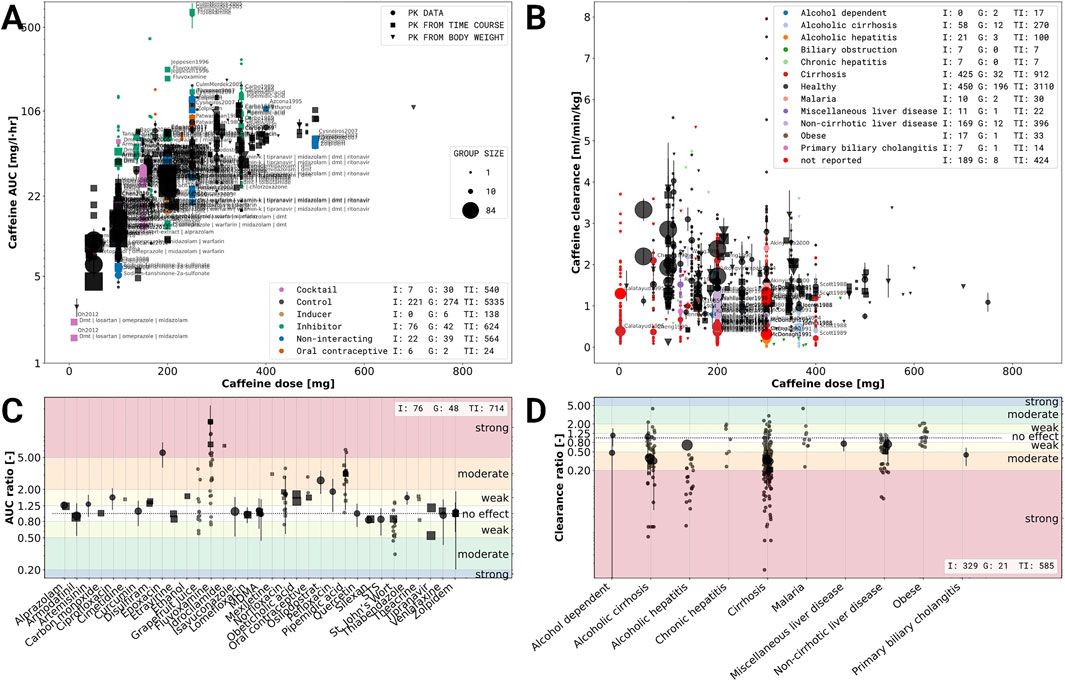
FIGURE 5. Effects of caffeine-drug and caffeine-disease interactions. (A) Caffeine-drug interactions based on the caffeine area under the concentration curve (AUC). Data is stratified based on co-administration of drugs with caffeine and dose. Violet: caffeine administrated as part of a drug cocktail. Common co-administrations are dextrometorphan, metoprolol, midazolam, omeprazole, and warfarin.; Black: single caffeine administration (no co-administration); Brown: co-administration with an inducing effect on the elimination of caffeine; Green: co-administration with an inhibiting effect on the elimination of caffeine; Blue: co-administrations with no effect on the pharmacokinetics of caffeine; Orange co-administration of oral contraceptives. (B) Caffeine-disease interactions based on caffeine clearance and dose. Data was stratified based on the health status and reported diseases, with black data points corresponding to healthy subjects. (C) Effect sizes of caffeine-drug interaction for studies with a controlled study design, mostly randomized control trials (RCT). The effect size is based on the log AUC ratio between caffeine application alone and caffeine with co-administration of the respective drug. The drugs were characterized as having either a strong, moderate, weak or no effect. Strong, moderate and weak inhibitors increase the AUC
Only case-controlled studies, mostly cross-over trials with a washout phase were included in the analysis. Corresponding controls were not matched across different studies. Overall coadministration data with AUC difference was available for 33 substances in our data set. In accordance with FDA, EMA and PMDA guidelines (Sudsakorn et al., 2020) we classified substances as inhibitors or inducers of caffeine clearance based on changes in AUC: FDA–Clinical DDI guidance: strong, moderate and weak inhibitors increase the AUC
Most substances do not affect the AUC of caffeine, with the exception of fluvoxamine, pipemidic acid and norfloxacin, which inhibit caffeine clearance. Tipranavir was the only substance showing a weak induction of caffeine clearance, but only in steady state dosing (not after a single dose) (Dumond et al., 2010)). Substances administered as a cocktail along side caffeine, aiming to phenotype several enzymes simultaneously did not affect the AUC of caffeine, substantiating the use of caffeine as part of a drug cocktail design (Turpault et al., 2009; Dumond et al., 2010; Oh et al., 2012; Doroshyenko et al., 2013; Kakuda et al., 2014; Tanaka et al., 2014; Armani et al., 2017; Edwards et al., 2017; Lammers et al., 2018). Our analysis shows that only a minority of studied drugs show an interaction with caffeine, confirming its value for phenotyping even under co-administration. As a side note, the protocols for studying caffeine-drug interactions were highly variable, e.g., the applied caffeine dose and the dose of the coadministrated substance varied between studies. Our results suggest that most medications can be safely consumed in combination with caffeine with exception of the antidepressant fluvoxamine, the antibacterial pipemidic acid and the antibiotic norfloxacin in which case caution is warranted.
3.6 Caffeine-Disease Interactions
An important question for using caffeine as a test substance for liver function testing and phenotyping, as well as for drugs metabolized via CYP1A2, is how disease affects the pharmacokinetics and elimination of caffeine. To study this question, we stratified caffeine clearance rates based on the reported disease of subjects and groups in the data set. To quantify the effect of disease the absolute clearance of caffeine (Figure 5B) and the logarithmic difference to a control group
None of the reported diseases increased the clearance rate of caffeine. Most of the diseases contained in this data set are diseases of the liver (e.g., alcoholic cirrhosis, primary biliary cholangitis) or are known to affect the liver (e.g., alcohol dependent). Cirrhotic liver disease had moderate to strong effects on the caffeine clearance with large variability in the reported data. Malaria and obesity had no effect on clearance with caffeine. An issue in the study of caffeine-disease interaction is that control group and disease group are different subjects (no cross-over design). In addition diseases were reported very heterogeneously (e.g., either only cirrhosis or with underlying cause such as alcoholic cirrhosis).
3.7 Metabolic Phenotyping
An important question for metabolic phenotyping and liver function tests with caffeine is how saliva measurements correlate with plasma or serum caffeine measurements, in the following referred to as blood-based measurements. A good correlation would allow simple non-invasive phenotyping using saliva samples. To study this question we analyzed 1) the relationship of blood-based concentrations of caffeine and paraxanthine with their respective saliva concentrations (Figures 6A,B); and 2) how the caffeine clearance measured in saliva correlate to blood-based measurements (Figure 6C).
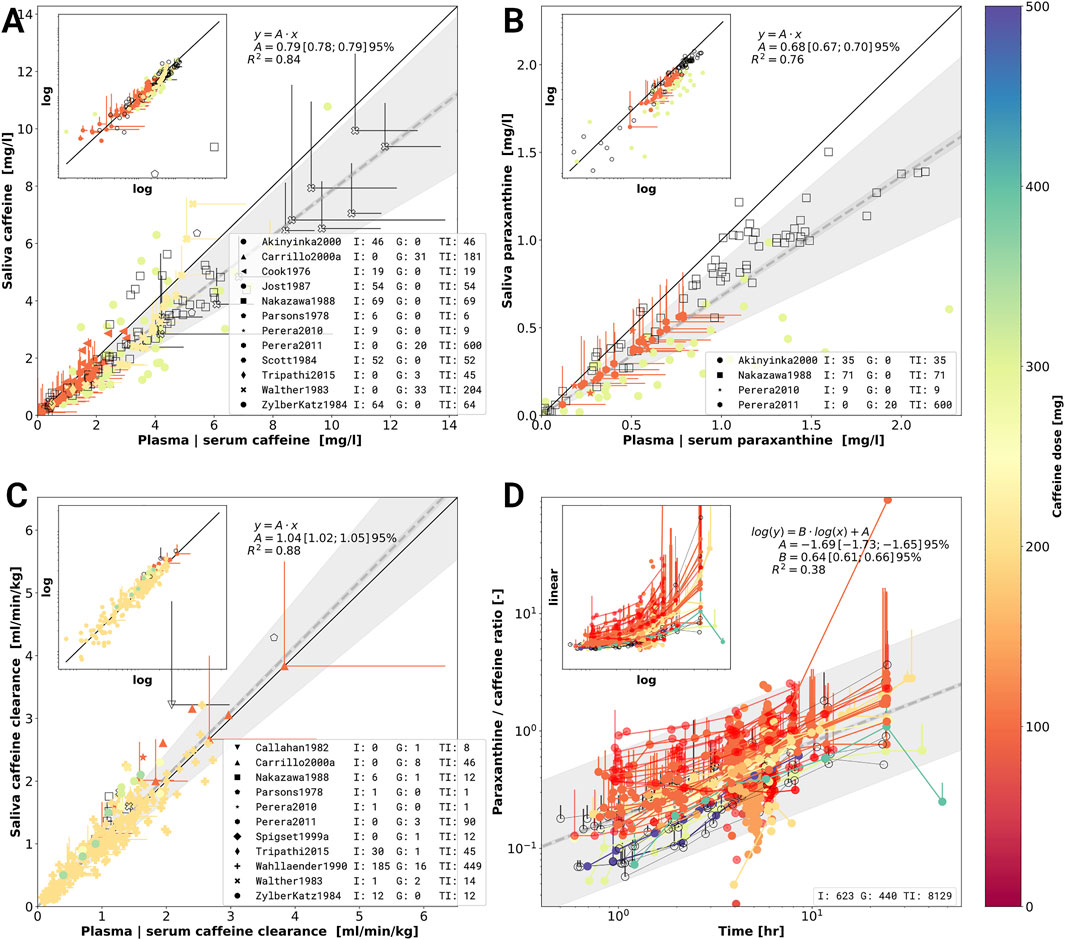
FIGURE 6. Meta-analysis of caffeine and paraxanthine concentrations in plasma, serum and saliva. (A) Caffeine concentrations in saliva versus caffeine concentrations in plasma or serum. Individual data points come from a single investigation taken at identical times after caffeine dosing. Marker shape encodes the different study. Markers are color-coded by caffeine dose in mg. Empty markers correspond to data in which dosage was reported per body weight but no information on the subject weight was available. (B) Paraxanthine concentrations in saliva versus paraxanthine concentrations in plasma or serum analogue to (A). (C) Caffeine clearance calculated from caffeine concentrations in saliva versus plasma or serum clearance. The panels A, B and C are in linear scale with a log-log inlet showing the same data. The dashed line in A, B and C represents a linear regression (y = A ⋅ x) with wide shaded area being 95% confidence interval of the sample variability and narrow shaded area the 95% confidence interval of the fitted mean of the scaling factor A. (D) Time dependency of the metabolic ratio paraxanthine/caffeine. Metabolic ratios are measured in plasma, serum, or saliva. Data points belonging to a single time course from a study are connected via a line. The dashed line corresponds to the linear regression log(y)= B ⋅ log(t)+ A. Jitter was applied on the time axes for better visibility of overlapping points. Data selection criteria and visualization are described in the Section 2.
Systematic errors due to different dosing protocols and different clinical investigation seem to be minimal as the data from multiple studies shows very consistent results. Linear regressions were performed to quantify the relation between saliva and blood-based caffeine and paraxanthine measurements (see Figures 6A–C). The resulting scaling factors of saliva to blood-based concentration of caffeine and paraxanthine are 0.79 ± 0.01 and 0.68 ± 0.02 (
When comparing saliva-based caffeine clearance against blood-based clearance (Figure 6C) an even stronger correlation of 0.88 with a scaling factor of 01.04 ± 0.02 (
Paraxanthine/caffeine ratios are mainly used for metabolic phenotyping based on caffeine. Whereas most studies use 6 h to phenotype no clear consensus exists in the literature and metabolic ratios are reported for varying time points after caffeine application. Paraxanthine/caffeine ratios for caffeine administered either as a single dose or in a cocktail to healthy, non-smoking, non-pregnant, and non-oral contraceptive consuming subjects were investigated (Figure 6D). By applying this strict data filtering, the variability due to either smoking and oral contraceptive use (see Section 3.3), caffeine-drug interactions (see Section 3.5) or disease (see Section 3.6) could be removed from the metabolic ratios.
Early and late time-sampling are least suitable for phenotyping. At these time points concentrations are low, resulting in relatively high random errors and thus low single to noise ratio. In an early stage, the outcome of metabolic ratios are further influenced by the distribution phase of the substance and its absorption kinetics, both affected by form and route of administration. Main results are that the metabolic phenotyping with paraxanthine/caffeine ratios is strongly time dependent with increasing ratios with time; and that a clear caffeine-dose dependency exists in the phenotyping with smaller caffeine doses increasing the metabolic ratio. Our results show the importance of clear standardized protocols for metabolic phenotyping.
In summary, by integrating data from multiple studies we could show a very good correlation between saliva and plasma caffeine concentrations, paraxanthine concentrations and pharmacokinetic parameters calculated from saliva versus plasma concentrations. This pooled data provides a strong argument for caffeine phenotyping based on saliva samples. Furthermore, we analyzed the time dependency of paraxanthine/caffeine ratios often used for phenotyping of CYP1A2. Our time dependent correlation allows to correct caffeine/paraxanthine ratios depending on the time after application and caffeine dose.
4 Discussion
Within this work, we performed a systematic data integration and multiple data analyses of reported data on caffeine pharmacokinetics in adults focusing on applications in metabolic phenotyping and liver function testing. To our knowledge, this is the largest open pharmacokinetics data set in humans with this kind of data being urgently needed to enable reproducible pharmacokinetics (Ioannidis, 2019). Data integration from multiple sources allows to solidify existing knowledge, increase statistical power, increase generalizability, and create new insights into the relationship between variables (Thacker, 1988). We show for instance, by systematically curating group and subject information on smoking status and oral contraceptive use a reproducible and consistent effect of smoking induction and inhibition via oral contraceptives over many studies and the complete dose regime of applied caffeine. Only a small subset of studies was specifically designed to study these questions (e.g., smoking by (Parsons and Neims, 1978; Benowitz et al., 2003; Backman et al., 2008; Gunes et al., 2009) and oral contraceptives by (Patwardhan et al., 1980; Rietveld et al., 1984; Abernethy and Todd, 1985)). Publicly providing comprehensive pharmacokinetics data in combination with detailed metadata allows to study new aspects of caffeine pharmacokinetics often not even anticipated by the original investigators. One example of such a new aspect is the assessment of dose and time-dependency of metabolic phenotyping via paraxanthine/caffeine ratios even if many of the data sets only report data for a single time point and dose.
Within this work we could confirm that oral contraceptives, smoking and drugs such as fluvoxamine or pipedemic acid alter the pharmacokinetics of caffeine, and that a saliva based metabolic phenotyping approach has very good correlation with blood based approaches, thereby solidifying existing knowledge. In addition novel aspects of the pharmacokinetics of caffeine could be elucidated. By data integration we could not only study the dose dependency of caffeine pharmacokinetics parameters, but also of the effects of smoking and oral contraceptives. For the first time we could show that both the induction of caffeine clearance by smoking and the reduction of caffeine clearance by oral contraceptives is an effect independent of the actual caffeine dose and that caffeine pharmacokinetics is affected by these confounding factors over a wide dose range. Important new results for the metabolic phenotyping with caffeine are our analysis of dose and time-dependency of paraxanthine/caffeine ratios. To our knowledge this time-dose-dependency has not been reported so far, and is especially relevant for phenotyping under very low doses such as cocktail approaches. Based on our results metabolic phenotyping data could be corrected for the dosage and time effects, thereby allowing to integrate data taken under various phenotyping protocols.
The dosage of a substance can have large effects on pharmacokinetic parameters. Using the data set we could evaluate the effect of caffeine dose on pharmacokinetic parameters systematically, e.g., on clearance (Figure 3A, Figure 4A, Figure 5B), half-life (Figure 3B, Figure 4B, Figure 5B), AUC (Figure 5A), or time-dependence of paraxanthine/caffeine ratios used in metabolic phenotyping (Figure 6D) by either directly using dose as a dimension of our analysis or by color coding based on dose. Caffeine dose has strong effects on the AUC of caffeine or the metabolic ratios of paraxanthine/caffeine whereas no clear effects on clearance or half-life could be observed. Our data set and analyses underline the importance of correcting for caffeine dose in the analysis of pharmacokinetic parameters and provide information for dosage corrections.
Various separation methods and detectors (mass spectrometry, UV/Vis, immunoassays or photodiode array) can be used for the quantification of caffeine metabolites and pharmacokinetics. The applied technique and assay can have large confounding effects. For instance cross-reactivity between caffeine and its metabolites have lead to false identification of concentrations by immunoassays (e.g., EMIT, ELISA) (Fligner and Opheim 1988). In earlier studies for example, EMIT methods showed a high cross-reactivity to paraxanthine (28%) Zysset et al. (1984). Newer methods showed low cross reactivity towards paraxanthine (0.08%), however, high cross-reactivity towards theophyline (16%) (Carvalho et al., 2012). Based on our analysis no systematic difference between the reported type of assays could be detected (see Figure 3, Figure 4). This result is limited by the details of reporting with many studies not providing sufficient information on the quantification method.
An important outcome of our analysis are the very good correlations between saliva- and blood-based measurements for caffeine with 0.77 R2 = 0.74 in very good agreement with data reported previously in individual studies 0.74 ± 0.1 R2 = 0.98 (Akinyinka et al., 2000), 0.79 ± 0.05 R2 = 0.96 (Zylber-Katz et al., 1984), 0.74 ± 0.08 (Newton et al., 1981), 0.74 ± 0.08 R2 = 0.90 (Jost et al., 1987), 0.71 R2 = 0.89 (Scott et al., 1984), 0.73 ± 0.06 (Walther et al., 1983), and for paraxanthine 0.68 R2 = 0.76 compared to 0.77 R2 = 0.91 (Nakazawa and Tanaka, 1988).
Several studies have shown that blood-derived pharmacokinetic parameters show excellent correlation with saliva-derived parameters (Newton et al., 1981; Akinyinka et al., 2000). We could confirm this observation when systematically analyzing the correlation between saliva- and plasma/serum-derived clearance. By integration of data from multiple studies we could increase the power of the conclusion and show the robustness of the reported correlation.
Overall we could show that the data from multiple studies are in very good agreement with each other after excluding data with confounding factors such as smoking or oral contraceptive use. These integrated results are a strong argument for saliva based metabolic phenotyping and liver function tests with caffeine, with sampling from saliva being convenient, painless, economical, without the requirement for special devices. Further, they allow simple repeated sampling as often required for pharmacokinetic research (Zylber-Katz et al., 1984).
Our systematic curation and analysis of reported caffeine data provided an overview of the current state and limitations of reporting of pharmacokinetic data. In summary, an accepted standard, minimum information guidelines, and standardized meta-data for the reporting of pharmacokinetics data of caffeine are missing. This finding is apparently not only true for the pharmacokinetics of caffeine but rather generally true for reporting of pharmacokinetics research. Major shortcomings in reporting are missing minimum information on factors that are known to influence the pharmacokinetics of caffeine (e.g., smoking and oral contraceptive status). Often not even basic subject information (e.g., weight, sex, or age) are reported. These factors are essential in the analysis of the pharmacokinetics of any substance in vivo (Stader et al., 2019). In general-purpose pharmacokinetic data sets, concentration-time profiles are the fundamental and most valuable data type. Common practice however is not to report the raw measured data but only derived pharmacokinetic parameters or metabolic ratios and not on individual participants level. Individual participants data have major advantages (Riley et al., 2010). We strongly advocate the reporting of all data on an individual level while including detailed anonymized meta information alongside the concentration-time profiles. Access to the individual raw data would enable data integration with different data sets and the stratification of the data under various aspects. There are recent efforts in creating a standard resource for that matter (Grzegorzewski et al., 2020).
Data integration and meta-analysis methods may be limited by selection bias, performance bias, detection bias, attrition bias, reporting bias and other biases (Higgins and Collaboration, 2020) but the extent of it in the field of pharmacokinetics is at large unknown (Ioannidis, 2019). Based on our data set we could evaluate the bias due to the type of assay and concluded that no bias could be found. Despite being the most comprehensive analysis so far, we could only present selected aspects mainly driven by the availability of reported data. Our focus in this study was on key factors affecting caffeine pharmacokinetics (smoking and oral contraceptive use), caffeine-drug and caffeine-disease interactions, as well as information relevant for the metabolic phenotyping and liver function testing with caffeine. Other important factors such as the pharmacogenetics of caffeine or urinary metabolic ratios have not been presented. Importantly, the corresponding data was curated and is readily available in PK-DB, but often very sparse (e.g., in case of genetic variants) or very heterogeneous (e.g., in case of urinary data). From our study (Table 1 and other investigations Koonrungsesomboon et al. (2018), it is striking, how little caffeine pharmacogenetics data is captured in the literature despite high heritability of CYP1A2 activity (Rasmussen et al., 2002; Matthaei et al., 2016). Our systematic analysis identified this gap of knowledge and more research in this area is needed.
Despite many implemented measures to ensure high data quality (e.g., validation rules and checking of studies by multiple curators), we are aware that the created data set may contain mistakes. Please report such instances so that these can be resolved.
The scope of the presented data set is limited to caffeine pharmacokinetics in human adults. As identified by the systemic search, the data set is far from comprehensive. At very least further 145 studies are eligible for inclusion, but were classified as low priority for the analyses. Future work will include this data and extend our data curation effort towards children and infants. Beyond the 145 missing studies, additional studies with relevant data exist. Please contact us in such cases so that we can include these additional studies. Contribution of missing data is highly appreciated. Also if you want to contribute a caffeine data set of your own please get in contact.
Importantly, our results are not only applicable to caffeine, but many aspects can be translated to other substances metabolized via CYP1A2, e.g., to the LiMAx liver function tests based on the CYP1A2 substrate methacetin (Rubin et al., 2017). For instance based on our analysis we expect smoking and oral contraceptive use to be confounding factors of LiMAx tests, which should be recorded and be accounted for in the evaluation of liver function.
The large inter-individual variability in caffeine pharmacokinetics is a major limitation for metabolic phenotyping and liver function tests. Our work allowed for the first time to systematically evaluate the effect of key factors on caffeine pharmacokinetics such as smoking, oral contraceptive usage or caffeine-drug interactions. An important next step will be the development of methods to quantify and correct for these confounding factors. This could allow to reduce variability in caffeine based testing. A promising tool in this context are physiological-based pharmacokinetics (PBPK) models (Jones and Rowland-Yeo, 2013) using information from the established data set as input for stratification and individualization. We could recently show that such an approach based on a similar data set for indocyanine green (ICG) allowed to account for important factors affecting ICG based liver function tests (Köller et al., 2021).
Data Availability Statement
Publicly available datasets were analyzed in this study. This data can be found here: https://pk-db.com.
Author Contributions
JG and MK designed the study, implemented and performed the analysis, curated the majority of caffeine studies and wrote the initial draft of the manuscript. FB and AK curated a subset of caffeine studies. All authors discussed the results. All authors contributed to and revised the manuscript critically.
Funding
JG, FB, AK, and JG were supported by the Federal Ministry of Education and Research (BMBF, Germany) within the research network Systems Medicine of the Liver (LiSyM, grant number 031L0054). MK, FB and AK were supported by the German Research Foundation (DFG) within the Research Unit Programme FOR 5151 “QuaLiPerF (Quantifying Liver Perfusion-Function Relationship in Complex Resection–A Systems Medicine Approach)” by Grant number 436 883 643. We acknowledge support by the German Research Foundation (DFG) and the Open Access Publication Fund of Humboldt-Universität zu Berlin.
Conflict of Interest
The authors declare that the research was conducted in the absence of any commercial or financial relationships that could be construed as a potential conflict of interest.
Publisher’s Note
All claims expressed in this article are solely those of the authors and do not necessarily represent those of their affiliated organizations, or those of the publisher, the editors, and the reviewers. Any product that may be evaluated in this article, or claim that may be made by its manufacturer, is not guaranteed or endorsed by the publisher.
Acknowledgments
We acknowledge support by the BMBF-funded de.NBI Cloud within the German Network for Bioinformatics Infrastructure (de.NBI) (031A537B, 031A533A, 031A538A, 031A533B, 031A535A, 031A537C, 031A534A, 031A532B) for the PK-DB infrastructure.
References
Abernethy, D. R., and Todd, E. L. (1985). Impairment of Caffeine Clearance by Chronic Use of Low-Dose Oestrogen-Containing Oral Contraceptives. Eur. J. Clin. Pharmacol. 28, 425–428. doi:10.1007/BF00544361
Abernethy, D. R., Todd, E. L., and Schwartz, J. B. (1985). Caffeine Disposition in Obesity. Br. J. Clin. Pharmacol. 20, 61–66. doi:10.1111/j.1365-2125.1985.tb02799.x
Akinyinka, O. O., Sowunmi, A., Honeywell, R., and Renwick, A. G. (2000). The Effects of Acute Falciparum Malaria on the Disposition of Caffeine and the Comparison of Saliva and Plasma-Derived Pharmacokinetic Parameters in Adult Nigerians. Eur. J. Clin. Pharmacol. 56, 159–165. doi:10.1007/s002280050735
Amchin, J., Zarycranski, W., Taylor, K. P., Albano, D., and Klockowski, P. M. (1999). Effect of Venlafaxine on Cyp1a2-dependent Pharmacokinetics and Metabolism of Caffeine. J. Clin. Pharmacol. 39, 252–259.
Armani, S., Ting, L., Sauter, N., Darstein, C., Tripathi, A. P., Wang, L., et al. (2017). Drug Interaction Potential of Osilodrostat (Lci699) Based on its Effect on the Pharmacokinetics of Probe Drugs of Cytochrome P450 Enzymes in Healthy Adults. Clin. Drug Investig. 37, 465–472. doi:10.1007/s40261-017-0497-0
Arnaud, M. J. (2011). Pharmacokinetics and Metabolism of Natural Methylxanthines in Animal and Man. Handb Exp. Pharmacol. 200, 33–91. doi:10.1007/978-3-642-13443-2_3
Arnaud, M. J., and Welsch, C. (1982). “Theophylline and Caffeine Metabolism in Man,” in Theophylline and other Methylxanthines/Theophyllin und andere Methylxanthine. Methods in Clinical Pharmacology. Editors N. Rietbrock, B. G. Woodcock, and A. H. Staib (Wiesbaden, Germany: Vieweg), 3, 135–148. doi:10.1007/978-3-663-05268-5_18
Arold, G., Donath, F., Maurer, A., Diefenbach, K., Bauer, S., Henneicke-von Zepelin, H. H., et al. (2005). No Relevant Interaction with Alprazolam, Caffeine, Tolbutamide, and Digoxin by Treatment with a Low-Hyperforin St John's Wort Extract. Planta Med. 71, 331–337. doi:10.1055/s-2005-864099
Axelrod, J., and Reichenthal, J. (1953). The Fate of Caffeine in Man and a Method for its Estimation in Biological Material. J. Pharmacol. Exp. Ther. 107, 519–523.
Azcona, O., Barbanoj, M. J., Torrent, J., and Jané, F. (1995). Evaluation of the central Effects of Alcohol and Caffeine Interaction. Br. J. Clin. Pharmacol. 40, 393–400. doi:10.1111/j.1365-2125.1995.tb04562.x
Backman, J. T., Schröder, M. T., and Neuvonen, P. J. (2008). Effects of Gender and Moderate Smoking on the Pharmacokinetics and Effects of the Cyp1a2 Substrate Tizanidine. Eur. J. Clin. Pharmacol. 64, 17–24. doi:10.1007/s00228-007-0389-y
Balogh, A., Harder, S., Vollandt, R., and Staib, A. H. (1992). Intra-individual Variability of Caffeine Elimination in Healthy Subjects. Int. J. Clin. Pharmacol. Ther. Toxicol. 30, 383–387.
Balogh, A., Klinger, G., Henschel, L., Börner, A., Vollanth, R., and Kuhnz, W. (1995). Influence of Ethinylestradiol-Containing Combination Oral Contraceptives with Gestodene or Levonorgestrel on Caffeine Elimination. Eur. J. Clin. Pharmacol. 48, 161–166. doi:10.1007/BF00192743
Bapiro, T. E., Sayi, J., Hasler, J. A., Jande, M., Rimoy, G., Masselle, A., et al. (2005). Artemisinin and Thiabendazole Are Potent Inhibitors of Cytochrome P450 1a2 (Cyp1a2) Activity in Humans. Eur. J. Clin. Pharmacol. 61, 755–761. doi:10.1007/s00228-005-0037-3
Barnett, G., Segura, J., de la Torre, R., and Carbó, M. (1990). Pharmacokinetic Determination of Relative Potency of Quinolone Inhibition of Caffeine Disposition. Eur. J. Clin. Pharmacol. 39, 63–69. doi:10.1007/BF02657060
Bchir, F., Dogui, M., Ben Fradj, R., Arnaud, M. J., and Saguem, S. (2006). Differences in Pharmacokinetic and Electroencephalographic Responses to Caffeine in Sleep-Sensitive and Non-sensitive Subjects. C R. Biol. 329, 512–519. doi:10.1016/j.crvi.2006.01.006
Beach, C. A., Mays, D. C., Guiler, R. C., Jacober, C. H., and Gerber, N. (1986). Inhibition of Elimination of Caffeine by Disulfiram in normal Subjects and Recovering Alcoholics. Clin. Pharmacol. Ther. 39, 265–270. doi:10.1038/clpt.1986.37
Becker, A. B., Simons, K. J., Gillespie, C. A., and Simons, F. E. (1984). The Bronchodilator Effects and Pharmacokinetics of Caffeine in Asthma. N. Engl. J. Med. 310, 743–746. doi:10.1056/NEJM198403223101202
Begas, E., Kouvaras, E., Tsakalof, A., Papakosta, S., and Asprodini, E. K. (2007). In Vivo evaluation of Cyp1a2, Cyp2a6, Nat-2 and Xanthine Oxidase Activities in a Greek Population Sample by the Rp-Hplc Monitoring of Caffeine Metabolic Ratios. Biomed. Chromatogr. 21, 190–200. doi:10.1002/bmc.736
Begas, E., Kouvaras, E., Tsakalof, A. K., Bounitsi, M., and Asprodini, E. K. (2015). Development and Validation of a Reversed-phase Hplc Method for Cyp1a2 Phenotyping by Use of a Caffeine Metabolite Ratio in Saliva. Biomed. Chromatogr. 29, 1657–1663. doi:10.1002/bmc.3475
Benowitz, N. L., Jacob, P., Mayan, H., and Denaro, C. (1995). Sympathomimetic Effects of Paraxanthine and Caffeine in Humans. Clin. Pharmacol. Ther. 58, 684–691. doi:10.1016/0009-9236(95)90025-X
Benowitz, N. L., Peng, M., and Jacob, P. (2003). Effects of Cigarette Smoking and Carbon Monoxide on Chlorzoxazone and Caffeine Metabolism. Clin. Pharmacol. Ther. 74, 468–474. doi:10.1016/j.clpt.2003.07.001
Birkett, D. J., and Miners, J. O. (1991). Caffeine Renal Clearance and Urine Caffeine Concentrations during Steady State Dosing. Implications for Monitoring Caffeine Intake during Sports Events. Br. J. Clin. Pharmacol. 31, 405–408. doi:10.1111/j.1365-2125.1991.tb05553.x
Blanchard, J., and Sawers, S. J. (1983b). Comparative Pharmacokinetics of Caffeine in Young and Elderly Men. J. Pharmacokinet. Biopharm. 11, 109–126. doi:10.1007/BF01061844
Blanchard, J., Sawers, S. J., Jonkman, J. H., and Tang-Liu, D. D. (1985). Comparison of the Urinary Metabolite Profile of Caffeine in Young and Elderly Males. Br. J. Clin. Pharmacol. 19, 225–232. doi:10.1111/j.1365-2125.1985.tb02635.x
Blanchard, J., and Sawers, S. J. (1983c). Relationship between Urine Flow Rate and Renal Clearance of Caffeine in Man. J. Clin. Pharmacol. 23, 134–138. doi:10.1002/j.1552-4604.1983.tb02716.x
Blanchard, J., and Sawers, S. J. (1983a). The Absolute Bioavailability of Caffeine in Man. Eur. J. Clin. Pharmacol. 24, 93–98. doi:10.1007/BF00613933
Bonati, M., Latini, R., Galletti, F., Young, J. F., Tognoni, G., and Garattini, S. (1982). Caffeine Disposition after Oral Doses. Clin. Pharmacol. Ther. 32, 98–106. doi:10.1038/clpt.1982.132
Bozikas, V. P., Papakosta, M., Niopas, I., Karavatos, A., and Mirtsou-Fidani, V. (2004). Smoking Impact on Cyp1a2 Activity in a Group of Patients with Schizophrenia. Eur. Neuropsychopharmacol. 14, 39–44. doi:10.1016/s0924-977x(03)00061-0
Brazier, J. L., Descotes, J., Lery, N., Ollagnier, M., and Evreux, J. C. (1980). Inhibition by Idrocilamide of the Disposition of Caffeine. Eur. J. Clin. Pharmacol. 17, 37–43. doi:10.1007/BF00561675
Broughton, L. J., and Rogers, H. J. (1981). Decreased Systemic Clearance of Caffeine Due to Cimetidine. Br. J. Clin. Pharmacol. 12, 155–159. doi:10.1111/j.1365-2125.1981.tb01194.x
Bruce, M., Scott, N., Lader, M., and Marks, V. (1986). The Psychopharmacological and Electrophysiological Effects of Single Doses of Caffeine in Healthy Human Subjects. Br. J. Clin. Pharmacol. 22, 81–87. doi:10.1111/j.1365-2125.1986.tb02883.x
Calatayud, O., Rodríguez, M., Sánchez-Alcaraz, A., and Ibáñez, P. (1995). Caffeine Test Assessment for Measuring Liver Function in Critically Ill Patients. J. Clin. Pharm. Ther. 20, 23–29. doi:10.1111/j.1365-2710.1995.tb00621.x
Callahan, M. M., Robertson, R. S., Arnaud, M. J., Branfman, A. R., McComish, M. F., and Yesair, D. W. (1982). Human Metabolism of [1-methyl-14c]- and [2-14c]caffeine after Oral Administration. Drug Metab. Dispos 10, 417–423.
Callahan, M. M., Robertson, R. S., Branfman, A. R., McComish, M. F., and Yesair, D. W. (1983). Comparison of Caffeine Metabolism in Three Nonsmoking Populations after Oral Administration of Radiolabeled Caffeine. Drug Metab. Dispos 11, 211–217.
Campbell, M. E., Spielberg, S. P., and Kalow, W. (1987). A Urinary Metabolite Ratio that Reflects Systemic Caffeine Clearance. Clin. Pharmacol. Ther. 42, 157–165. doi:10.1038/clpt.1987.126
Carbó, M., Segura, J., De la Torre, R., Badenas, J. M., and Camí, J. (1989). Effect of Quinolones on Caffeine Disposition. Clin. Pharmacol. Ther. 45, 234–240. doi:10.1038/clpt.1989.23
Carrillo, J. A., Christensen, M., Ramos, S. I., Alm, C., Dahl, M. L., Benitez, J., et al. (2000). Evaluation of Caffeine as an In Vivo Probe for Cyp1a2 Using Measurements in Plasma, Saliva, and Urine. Ther. Drug Monit. 22, 409–417. doi:10.1097/00007691-200008000-00008
Carvalho, J. J., Weller, M. G., Panne, U., and Schneider, R. J. (2012). Monitoring Caffeine in Human Saliva Using a Newly Developed ELISA. Anal. Lett. 45, 2549–2561. doi:10.1080/00032719.2012.696226
Chen, Y., Liu, W. H., Chen, B. L., Fan, L., Han, Y., Wang, G., et al. (2010). Plant Polyphenol Curcumin Significantly Affects Cyp1a2 and Cyp2a6 Activity in Healthy, Male Chinese Volunteers. Ann. Pharmacother. 44, 1038–1045. doi:10.1345/aph.1M533
Chen, Y., Tu, J. H., He, Y. J., Zhang, W., Wang, G., Tan, Z. R., et al. (2009a). Effect of Sodium Tanshinone Ii a Sulfonate on the Activity of Cyp1a2 in Healthy Volunteers. Xenobiotica 39, 508–513. doi:10.1080/00498250902951763
Chen, Y., Xiao, P., Ou-Yang, D. S., Fan, L., Guo, D., Wang, Y. N., et al. (2009b). Simultaneous Action of the Flavonoid Quercetin on Cytochrome P450 (Cyp) 1a2, Cyp2a6, N-Acetyltransferase and Xanthine Oxidase Activity in Healthy Volunteers. Clin. Exp. Pharmacol. Physiol. 36, 828–833. doi:10.1111/j.1440-1681.2009.05158.x
Cheng, W. S., Murphy, T. L., Smith, M. T., Cooksley, W. G., Halliday, J. W., and Powell, L. W. (1990). Dose-dependent Pharmacokinetics of Caffeine in Humans: Relevance as a Test of Quantitative Liver Function. Clin. Pharmacol. Ther. 47, 516–524. doi:10.1038/clpt.1990.66
Chia, H. Y., Yau, W. P., and Ho, H. K. (2016). Establishing Population Distribution of Drug-Metabolizing Enzyme Activities for the Use of Salivary Caffeine as a Dynamic Liver Function Marker in a Singaporean Chinese Population. Biopharm. Drug Dispos 37, 168–181. doi:10.1002/bdd.2006
Christensen, M., Tybring, G., Mihara, K., Yasui-Furokori, N., Carrillo, J. A., Ramos, S. I., et al. (2002). Low Daily 10-mg and 20-mg Doses of Fluvoxamine Inhibit the Metabolism of Both Caffeine (Cytochrome P4501a2) and Omeprazole (Cytochrome P4502c19). Clin. Pharmacol. Ther. 71, 141–152. doi:10.1067/mcp.2002.121788
Cook, C. E., Tallent, C. R., Amerson, E. W., Myers, M. W., Kepler, J. A., Taylor, G. F., et al. (1976). Caffeine in Plasma and Saliva by a Radioimmunoassay Procedure. J. Pharmacol. Exp. Ther. 199, 679–686.
Cornish, H. H., and Christman, A. A. (1957). A Study of the Metabolism of Theobromine, Theophylline, and Caffeine in Man. J. Biol. Chem. 228, 315–323. doi:10.1016/s0021-9258(18)70714-x
Culm-Merdek, K. E., von Moltke, L. L., Harmatz, J. S., and Greenblatt, D. J. (2005). Fluvoxamine Impairs Single-Dose Caffeine Clearance without Altering Caffeine Pharmacodynamics. Br. J. Clin. Pharmacol. 60, 486–493. doi:10.1111/j.1365-2125.2005.02467.x
Cysneiros, R. M., Farkas, D., Harmatz, J. S., von Moltke, L. L., and Greenblatt, D. J. (2007). Pharmacokinetic and Pharmacodynamic Interactions between Zolpidem and Caffeine. Clin. Pharmacol. Ther. 82, 54–62. doi:10.1038/sj.clpt.6100211
Darwish, M., Kirby, M., Robertson, P., and Hellriegel, E. T. (2008). Interaction Profile of Armodafinil with Medications Metabolized by Cytochrome P450 Enzymes 1a2, 3a4 and 2c19 in Healthy Subjects. Clin. Pharmacokinet. 47, 61–74. doi:10.2165/00003088-200847010-00006
Desmond, P. V., Patwardhan, R. V., Johnson, R. F., and Schenker, S. (1980). Impaired Elimination of Caffeine in Cirrhosis. Dig. Dis. Sci. 25, 193–197. doi:10.1007/BF01308138
Dinger, J., Woods, C., Brandt, S. D., Meyer, M. R., and Maurer, H. H. (2016). Cytochrome P450 Inhibition Potential of New Psychoactive Substances of the Tryptamine Class. Toxicol. Lett. 241, 82–94. doi:10.1016/j.toxlet.2015.11.013
Djordjevic, N., Ghotbi, R., Bertilsson, L., Jankovic, S., and Aklillu, E. (2008). Induction of Cyp1a2 by Heavy Coffee Consumption in Serbs and Swedes. Eur. J. Clin. Pharmacol. 64, 381–385. doi:10.1007/s00228-007-0438-6
Doroshyenko, O., Rokitta, D., Zadoyan, G., Klement, S., Schläfke, S., Dienel, A., et al. (2013). Drug Cocktail Interaction Study on the Effect of the Orally Administered Lavender Oil Preparation Silexan on Cytochrome P450 Enzymes in Healthy Volunteers. Drug Metab. Dispos 41, 987–993. doi:10.1124/dmd.112.050203
Drozdzik, M., Busch, D., Lapczuk, J., Müller, J., Ostrowski, M., Kurzawski, M., et al. (2018). Protein Abundance of Clinically Relevant Drug-Metabolizing Enzymes in the Human Liver and Intestine: A Comparative Analysis in Paired Tissue Specimens. Clin. Pharmacol. Ther. 104, 515–524. doi:10.1002/cpt.967
Dumond, J. B., Vourvahis, M., Rezk, N. L., Patterson, K. B., Tien, H. C., White, N., et al. (2010). A Phenotype-Genotype Approach to Predicting Cyp450 and P-Glycoprotein Drug Interactions with the Mixed Inhibitor/inducer Tipranavir/ritonavir. Clin. Pharmacol. Ther. 87, 735–742. doi:10.1038/clpt.2009.253
Edwards, J. E., Eliot, L., Parkinson, A., Karan, S., and MacConell, L. (2017). Assessment of Pharmacokinetic Interactions between Obeticholic Acid and Caffeine, Midazolam, Warfarin, Dextromethorphan, Omeprazole, Rosuvastatin, and Digoxin in Phase 1 Studies in Healthy Subjects. Adv. Ther. 34, 2120–2138. doi:10.1007/s12325-017-0601-0
Faber, M. S., and Fuhr, U. (2004). Time Response of Cytochrome P450 1a2 Activity on Cessation of Heavy Smoking. Clin. Pharmacol. Ther. 76, 178–184. doi:10.1016/j.clpt.2004.04.003
Faber, M. S., Jetter, A., and Fuhr, U. (2005). Assessment of Cyp1a2 Activity in Clinical Practice: Why, How, and when? Basic Clin. Pharmacol. Toxicol. 97, 125–134. doi:10.1111/j.1742-7843.2005.pto_973160.x
Fligner, C. L., and Opheim, K. E. (1988). Caffeine and its Dimethylxanthine Metabolites in Two Cases of Caffeine Overdose: a Cause of Falsely Elevated Theophylline Concentrations in Serum. J. Anal. Toxicol. 12, 339–343. doi:10.1093/jat/12.6.339
Frary, C. D., Johnson, R. K., and Wang, M. Q. (2005). Food Sources and Intakes of Caffeine in the Diets of Persons in the united states. J. Am. Diet. Assoc. 105, 110–113. doi:10.1016/j.jada.2004.10.027
Fuhr, U., Klittich, K., and Staib, A. H. (1993). Inhibitory Effect of Grapefruit Juice and its Bitter Principal, Naringenin, on Cyp1a2 Dependent Metabolism of Caffeine in Man. Br. J. Clin. Pharmacol. 35, 431–436. doi:10.1111/j.1365-2125.1993.tb04162.x
Fuhr, U., Rost, K. L., Engelhardt, R., Sachs, M., Liermann, D., Belloc, C., et al. (1996). Evaluation of caffeine as a test drug for cyp1a2, nat2 and cyp2e1 phenotyping in man by In Vivo versus In Vitro correlations. Pharmacogenetics 6, 159–176. doi:10.1097/00008571-199604000-00003
Furge, L. L., and Fletke, K. J. (2007). Hplc Determination of Caffeine and Paraxanthine in Urine: An Assay for Cytochrome P450 1a2 Activity. Biochem. Mol. Biol. Educ. 35, 138–144. doi:10.1002/bmb.28
George, J., Murphy, T., Roberts, R., Cooksley, W. G., Halliday, J. W., and Powell, L. W. (1986). Influence of Alcohol and Caffeine Consumption on Caffeine Elimination. Clin. Exp. Pharmacol. Physiol. 13, 731–736. doi:10.1111/j.1440-1681.1986.tb02414.x
Ghassabian, S., Chetty, M., Tattam, B. N., Chem, M. C., Glen, J., Rahme, J., et al. (2009). A High-Throughput Assay Using Liquid Chromatography-Tandem Mass Spectrometry for Simultaneous In Vivo Phenotyping of 5 Major Cytochrome P450 Enzymes in Patients. Ther. Drug Monit. 31, 239–246. doi:10.1097/FTD.0b013e318197e1bf
Ghotbi, R., Christensen, M., Roh, H. K., Ingelman-Sundberg, M., Aklillu, E., and Bertilsson, L. (2007). Comparisons of Cyp1a2 Genetic Polymorphisms, Enzyme Activity and the Genotype-Phenotype Relationship in Swedes and Koreans. Eur. J. Clin. Pharmacol. 63, 537–546. doi:10.1007/s00228-007-0288-2
Gilbert, R. (1984). The Methylxanthine Beverages and Foods: Chemistry, Consumption, and Health Effects. Prog. Clin. Biol. Res. 158, 1–413.
Gonzalez Hernandez, F., Carter, S. J., Iso-Sipilä, J., Goldsmith, P., Almousa, A. A., Gastine, S., et al. (2021). An Automated Approach to Identify Scientific Publications Reporting Pharmacokinetic Parameters. Wellcome Open Res. 6, 88. doi:10.12688/wellcomeopenres.16718.1
Granfors, M. T., Backman, J. T., Laitila, J., and Neuvonen, P. J. (2005). Oral Contraceptives Containing Ethinyl Estradiol and Gestodene Markedly Increase Plasma Concentrations and Effects of Tizanidine by Inhibiting Cytochrome P450 1a2. Clin. Pharmacol. Ther. 78, 400–411. doi:10.1016/j.clpt.2005.06.009
Grzegorzewski, J., Brandhorst, J., Green, K., Eleftheriadou, D., Duport, Y., Barthorscht, F., et al. (2020). Pk-db: Pharmacokinetics Database for Individualized and Stratified Computational Modeling. Nucleic Acids Res. 49, D1358–D1364. doi:10.1093/nar/gkaa990
Gunes, A., and Dahl, M. L. (2008). Variation in Cyp1a2 Activity and its Clinical Implications: Influence of Environmental Factors and Genetic Polymorphisms. Pharmacogenomics 9, 625–637. doi:10.2217/14622416.9.5.625
Gunes, A., Ozbey, G., Vural, E. H., Uluoglu, C., Scordo, M. G., Zengil, H., et al. (2009). Influence of Genetic Polymorphisms, Smoking, Gender and Age on Cyp1a2 Activity in a Turkish Population. Pharmacogenomics 10, 769–778. doi:10.2217/pgs.09.22
Gurley, B. J., Gardner, S. F., Hubbard, M. A., Williams, D. K., Gentry, W. B., Cui, Y., et al. (2005a). Clinical Assessment of Effects of Botanical Supplementation on Cytochrome P450 Phenotypes in the Elderly: St John's Wort, Garlic Oil, Panax Ginseng and Ginkgo Biloba. Drugs Aging 22, 525–539. doi:10.2165/00002512-200522060-00006
Gurley, B. J., Gardner, S. F., Hubbard, M. A., Williams, D. K., Gentry, W. B., Khan, I. A., et al. (2005b). In Vivo effects of goldenseal, kava kava, black cohosh, and valerian on human cytochrome p450 1a2, 2d6, 2e1, and 3a4/5 phenotypes. Clin. Pharmacol. Ther. 77, 415–426. doi:10.1016/j.clpt.2005.01.009
Hakooz, N. M. (2009). Caffeine Metabolic Ratios for the In Vivo Evaluation of Cyp1a2, N-Acetyltransferase 2, Xanthine Oxidase and Cyp2a6 Enzymatic Activities. Curr. Drug Metab. 10, 329–338. doi:10.2174/138920009788499003
Haller, C. A., Jacob, P., and Benowitz, N. L. (2002). Pharmacology of Ephedra Alkaloids and Caffeine after Single-Dose Dietary Supplement Use. Clin. Pharmacol. Ther. 71, 421–432. doi:10.1067/mcp.2002.124523
Hamon-Vilcot, B., Simon, T., Becquemont, L., Poirier, J. M., Piette, F., and Jaillon, P. (2004). Effects of Malnutrition on Cytochrome P450 1a2 Activity in Elderly Patients. Therapie 59, 247–251. doi:10.2515/therapie:2004048
Harder, S., Fuhr, U., Staib, A. H., and Wolff, T. (1989). Ciprofloxacin-caffeine: a Drug Interaction Established Using In Vivo and In Vitro Investigations. Am. J. Med. 87, 89S–91S. doi:10.1016/0002-9343(89)90031-4
Harder, S., Staib, A. H., Beer, C., Papenburg, A., Stille, W., and Shah, P. M. (1988). 4-quinolones Inhibit Biotransformation of Caffeine. Eur. J. Clin. Pharmacol. 35, 651–656. doi:10.1007/BF00637602
Hashiguchi, M., Fujimura, A., Ohashi, K., and Ebihara, A. (1992). Diurnal Effect on Caffeine Clearance. J. Clin. Pharmacol. 32, 184–187. doi:10.1002/j.1552-4604.1992.tb03824.x
He, H., Ma, D., Crone, L. B., Butawan, M., Meibohm, B., Bloomer, R. J., et al. (2017). Assessment of the Drug-Drug Interaction Potential between Theacrine and Caffeine in Humans. J. Caffeine Res. 7, 95–102. doi:10.1089/jcr.2017.0006
Healy, D. P., Polk, R. E., Kanawati, L., Rock, D. T., and Mooney, M. L. (1989). Interaction between Oral Ciprofloxacin and Caffeine in normal Volunteers. Antimicrob. Agents Chemother. 33, 474–478. doi:10.1128/aac.33.4.474
Healy, D. P., Schoenle, J. R., Stotka, J., and Polk, R. E. (1991). Lack of Interaction between Lomefloxacin and Caffeine in normal Volunteers. Antimicrob. Agents Chemother. 35, 660–664. doi:10.1128/aac.35.4.660
Hetzler, R. K., Knowlton, R. G., Somani, S. M., Brown, D. D., and Perkins, R. M. (19901985). Effect of Paraxanthine on Ffa Mobilization after Intravenous Caffeine Administration in Humans. J. Appl. Physiol. (1985) 68, 44–47. doi:10.1152/jappl.1990.68.1.44
Holstege, A., Kurz, M., Weinbeck, M., and Gerok, W. (1993). Excretion of Caffeine and its Primary Degradation Products into Bile. J. Hepatol. 17, 67–73. doi:10.1016/s0168-8278(05)80523-9
Holstege, A., Staiger, M., Haag, K., and Gerok, W. (1989). Correlation of Caffeine Elimination and Child's Classification in Liver Cirrhosis. Klin Wochenschr 67, 6–15. doi:10.1007/BF01736528
Ioannidis, J. P. A. (2019). Reproducible Pharmacokinetics. J. Pharmacokinet. Pharmacodyn 46, 111–116. doi:10.1007/s10928-019-09621-y
Jeppesen, U., Loft, S., Poulsen, H. E., and Brśen, K. (1996). A Fluvoxamine-Caffeine Interaction Study. Pharmacogenetics 6, 213–222. doi:10.1097/00008571-199606000-00003
Jiang, Z., Dragin, N., Jorge-Nebert, L. F., Martin, M. V., Guengerich, F. P., Aklillu, E., et al. (2006). Search for an Association between the Human Cyp1a2 Genotype and Cyp1a2 Metabolic Phenotype. Pharmacogenet Genomics 16, 359–367. doi:10.1097/01.fpc.0000204994.99429.46
Jodynis-Liebert, J., Flieger, J., Matuszewska, A., and Juszczyk, J. (2004). Serum Metabolite/caffeine Ratios as a Test for Liver Function. J. Clin. Pharmacol. 44, 338–347. doi:10.1177/0091270004263468
Joeres, R., Klinker, H., Heusler, H., Epping, J., and Richter, E. (1987). Influence of Mexiletine on Caffeine Elimination. Pharmacol. Ther. 33, 163–169. doi:10.1016/0163-7258(87)90046-5
Joeres, R., Klinker, H., Heusler, H., Epping, J., Zilly, W., and Richter, E. (1988). Influence of Smoking on Caffeine Elimination in Healthy Volunteers and in Patients with Alcoholic Liver Cirrhosis. Hepatology 8, 575–579. doi:10.1002/hep.1840080323
Jones, H., and Rowland-Yeo, K. (2013). Basic Concepts in Physiologically Based Pharmacokinetic Modeling in Drug Discovery and Development. CPT Pharmacometrics Syst. Pharmacol. 2, e63. doi:10.1038/psp.2013.41
Jost, G., Wahlländer, A., von Mandach, U., and Preisig, R. (1987). Overnight Salivary Caffeine Clearance: a Liver Function Test Suitable for Routine Use. Hepatology 7, 338–344. doi:10.1002/hep.1840070221
J. P. T. Higgins, and C. Collaboration (Editors) (2020). Cochrane Handbook for Systematic Reviews of Interventions. second edition edn (Hoboken, NJ: Wiley-Blackwell).
Kakuda, T. N., Van Solingen-Ristea, R. M., Onkelinx, J., Stevens, T., Aharchi, F., De Smedt, G., et al. (2014). The Effect of Single- and Multiple-Dose Etravirine on a Drug Cocktail of Representative Cytochrome P450 Probes and Digoxin in Healthy Subjects. J. Clin. Pharmacol. 54, 422–431. doi:10.1002/jcph.214
Kalow, W., and Tang, B. K. (1993). The Use of Caffeine for Enzyme Assays: a Critical Appraisal. Clin. Pharmacol. Ther. 53, 503–514. doi:10.1038/clpt.1993.63
Kamimori, G. H., Joubert, A., Otterstetter, R., Santaromana, M., and Eddington, N. D. (1999). The Effect of the Menstrual Cycle on the Pharmacokinetics of Caffeine in normal, Healthy Eumenorrheic Females. Eur. J. Clin. Pharmacol. 55, 445–449. doi:10.1007/s002280050654
Kamimori, G. H., Karyekar, C. S., Otterstetter, R., Cox, D. S., Balkin, T. J., Belenky, G. L., et al. (2002). The Rate of Absorption and Relative Bioavailability of Caffeine Administered in Chewing Gum versus Capsules to normal Healthy Volunteers. Int. J. Pharm. 234, 159–167. doi:10.1016/s0378-5173(01)00958-9
Kamimori, G. H., Somani, S. M., Knowlton, R. G., and Perkins, R. M. (1987). The Effects of Obesity and Exercise on the Pharmacokinetics of Caffeine in Lean and Obese Volunteers. Eur. J. Clin. Pharmacol. 31, 595–600. doi:10.1007/BF00606637
Kaplan, G. B., Greenblatt, D. J., Ehrenberg, B. L., Goddard, J. E., Cotreau, M. M., Harmatz, J. S., et al. (1997). Dose-dependent Pharmacokinetics and Psychomotor Effects of Caffeine in Humans. J. Clin. Pharmacol. 37, 693–703. doi:10.1002/j.1552-4604.1997.tb04356.x
Kinzig-Schippers, M., Fuhr, U., Zaigler, M., Dammeyer, J., Rüsing, G., Labedzki, A., et al. (1999). Interaction of Pefloxacin and Enoxacin with the Human Cytochrome P450 Enzyme Cyp1a2. Clin. Pharmacol. Ther. 65, 262–274. doi:10.1016/S0009-9236(99)70105-0
Klein, K., Winter, S., Turpeinen, M., Schwab, M., and Zanger, U. M. (2010). Pathway-targeted Pharmacogenomics of Cyp1a2 in Human Liver. Front. Pharmacol. 1, 129. doi:10.3389/fphar.2010.00129
Koch, J. P., ten Tusscher, G. W., Koppe, J. G., and Guchelaar, H. J. (1999). Validation of a High-Performance Liquid Chromatography Assay for Quantification of Caffeine and Paraxanthine in Human Serum in the Context of Cyp1a2 Phenotyping. Biomed. Chromatogr. 13, 309–314. doi:10.1002/(SICI)1099-0801(199906)13:4<309:AID-BMC881>3.0.CO;2-J
Köller, A., Grzegorzewski, J., Tautenhahn, H.-M., and König, M. (2021). Prediction of Survival after Partial Hepatectomy Using a Physiologically Based Pharmacokinetic Model of Indocyanine green Liver Function Tests. bioRxiv. doi:10.1101/2021.06.15.448411
Koonrungsesomboon, N., Khatsri, R., Wongchompoo, P., and Teekachunhatean, S. (2018). The Impact of Genetic Polymorphisms on CYP1A2 Activity in Humans: A Systematic Review and Meta-Analysis. Pharmacogenomics J. 18, 760–768. doi:10.1038/s41397-017-0011-3
Kot, M., and Daniel, W. A. (2008). Caffeine as a Marker Substrate for Testing Cytochrome P450 Activity in Human and Rat. Pharmacol. Rep. 60, 789–797.
Kukongviriyapan, V., Senggunprai, L., Prawan, A., Gaysornsiri, D., Kukongviriyapan, U., and Aiemsa-Ard, J. (2004). Salivary Caffeine Metabolic Ratio in Alcohol-dependent Subjects. Eur. J. Clin. Pharmacol. 60, 103–107. doi:10.1007/s00228-004-0734-3
Laizure, S. C., Meibohm, B., Nelson, K., Chen, F., Hu, Z. Y., and Parker, R. B. (2017). Comparison of Caffeine Disposition Following Administration by Oral Solution (Energy Drink) and Inspired Powder (Aeroshot) in Human Subjects. Br. J. Clin. Pharmacol. 83, 2687–2694. doi:10.1111/bcp.13389
Lammers, L. A., Achterbergh, R., Romijn, J. A., and Mathôt, R. A. A. (2018). Short-term Fasting Alters Pharmacokinetics of Cytochrome P450 Probe Drugs: Does Protein Binding Play a Role? Eur. J. Drug Metab. Pharmacokinet. 43, 251–257. doi:10.1007/s13318-017-0437-7
Lane, J. D., Steege, J. F., Rupp, S. L., and Kuhn, C. M. (1992). Menstrual Cycle Effects on Caffeine Elimination in the Human Female. Eur. J. Clin. Pharmacol. 43, 543–546. doi:10.1007/BF02285099
Lane, S. D., Green, C. E., Schmitz, J. M., Rathnayaka, N., Fang, W. B., Ferré, S., et al. (2014). Comparison of Caffeine and D-Amphetamine in Cocaine-dependent Subjects: Differential Outcomes on Subjective and Cardiovascular Effects, Reward Learning, and Salivary Paraxanthine. J. Addict. Res. Ther. 5, 176. doi:10.4172/2155-6105.1000176
Lelo, A., Birkett, D. J., Robson, R. A., and Miners, J. O. (1986a). Comparative Pharmacokinetics of Caffeine and its Primary Demethylated Metabolites Paraxanthine, Theobromine and Theophylline in Man. Br. J. Clin. Pharmacol. 22, 177–182. doi:10.1111/j.1365-2125.1986.tb05246.x
Lelo, A., Miners, J. O., Robson, R. A., and Birkett, D. J. (1986b). Quantitative Assessment of Caffeine Partial Clearances in Man. Br. J. Clin. Pharmacol. 22, 183–186. doi:10.1111/j.1365-2125.1986.tb05247.x
Levy, M., and Zylber-Katz, E. (1983). Caffeine Metabolism and Coffee-Attributed Sleep Disturbances. Clin. Pharmacol. Ther. 33, 770–775. doi:10.1038/clpt.1983.105
Liberati, A., Altman, D. G., Tetzlaff, J., Mulrow, C., Gøtzsche, P. C., Ioannidis, J. P., et al. (2009). The PRISMA Statement for Reporting Systematic Reviews and Meta-Analyses of Studies that Evaluate Healthcare Interventions: Explanation and Elaboration. BMJ 339, b2700. doi:10.1136/bmj.b2700
Magnusson, M. O., Dahl, M. L., Cederberg, J., Karlsson, M. O., and Sandström, R. (2008). Pharmacodynamics of Carbamazepine-Mediated Induction of Cyp3a4, Cyp1a2, and Pgp as Assessed by Probe Substrates Midazolam, Caffeine, and Digoxin. Clin. Pharmacol. Ther. 84, 52–62. doi:10.1038/sj.clpt.6100431
Marks, V., and Kelly, J. F. (1973). Absorption of Caffeine from tea, Coffee, and Coca Cola. Lancet 1, 827. doi:10.1016/s0140-6736(73)90625-9
Matthaei, J., Tzvetkov, M. V., Strube, J., Sehrt, D., Sachse-Seeboth, C., Hjelmborg, J. B., et al. (2016). Heritability of Caffeine Metabolism: Environmental Effects Masking Genetic Effects on Cyp1a2 Activity but Not on Nat2. Clin. Pharmacol. Ther. 100, 606–616. doi:10.1002/cpt.444
May, D. C., Jarboe, C. H., VanBakel, A. B., and Williams, W. M. (1982). Effects of Cimetidine on Caffeine Disposition in Smokers and Nonsmokers. Clin. Pharmacol. Ther. 31, 656–661. doi:10.1038/clpt.1982.91
McDonagh, J. E., Nathan, V. V., Bonavia, I. C., Moyle, G. R., and Tanner, A. R. (1991). Caffeine Clearance by Enzyme Multiplied Immunoassay Technique: a Simple, Inexpensive, and Useful Indicator of Liver Function. Gut 32, 681–684. doi:10.1136/gut.32.6.681
McLean, C., and Graham, T. E. (2002). Effects of Exercise and thermal Stress on Caffeine Pharmacokinetics in Men and Eumenorrheic Women. J. Appl. Physiol.93, 1471–1478. doi:10.1152/japplphysiol.00762.2000
McQuilkin, S. H., Nierenberg, D. W., and Bresnick, E. (1995). Analysis of Within-Subject Variation of Caffeine Metabolism when Used to Determine Cytochrome P4501a2 and N-Acetyltransferase-2 Activities. Cancer Epidemiol. Biomarkers Prev. 4, 139–146.
Millard, J. T., Passang, T., Ye, J., Kline, G. M., Beachy, T. M., Hepburn, V. L., et al. (2018). Genotype and Phenotype of Caffeine Metabolism: A Biochemistry Laboratory experiment. J. Chem. Educ. 95, 1856–1860. doi:10.1021/acs.jchemed.8b00318
Miners, J. O., and Birkett, D. J. (1996). The Use of Caffeine as a Metabolic Probe for Human Drug Metabolizing Enzymes. Gen. Pharmacol. 27, 245–249. doi:10.1016/0306-3623(95)02014-4
Mitchell, D. C., Knight, C. A., Hockenberry, J., Teplansky, R., and Hartman, T. J. (2014). Beverage Caffeine Intakes in the u.S. Food Chem. Toxicol. 63, 136–142. doi:10.1016/j.fct.2013.10.042
Moonen, H. J., Moonen, E. J., Maas, L., Dallinga, J. W., Kleinjans, J. C., and de Kok, T. M. (2004). CYP1A2 and NAT2 Genotype/phenotype Relations and Urinary Excretion of 2-Amino-1-Methyl-6-Phenylimidazo[4,5-B]pyridine (PhIP) in a Human Dietary Intervention Study. Food Chem. Toxicol. 42, 869–878. doi:10.1016/j.fct.2004.01.010
Murphy, T. L., McIvor, C., Yap, A., Cooksley, W. G., Halliday, J. W., and Powell, L. W. (1988). The Effect of Smoking on Caffeine Elimination: Implications for its Use as a Semiquantitative Test of Liver Function. Clin. Exp. Pharmacol. Physiol. 15, 9–13. doi:10.1111/j.1440-1681.1988.tb01003.x
Muscat, J. E., Pittman, B., Kleinman, W., Lazarus, P., Stellman, S. D., and Richie, J. P. (2008). Comparison of Cyp1a2 and Nat2 Phenotypes between Black and white Smokers. Biochem. Pharmacol. 76, 929–937. doi:10.1016/j.bcp.2008.07.024
Myrand, S. P., Sekiguchi, K., Man, M. Z., Lin, X., Tzeng, R. Y., Teng, C. H., et al. (2008). Pharmacokinetics/genotype Associations for Major Cytochrome P450 Enzymes in Native and First- and Third-Generation Japanese Populations: Comparison with Korean, Chinese, and Caucasian Populations. Clin. Pharmacol. Ther. 84, 347–361. doi:10.1038/sj.clpt.6100482
Nakazawa, K., and Tanaka, H. (1988). pharmacokinetics of Caffeine and Dimethylxanthines in Plasma and Saliva. Yakugaku Zasshi 108, 653–658. doi:10.1248/yakushi1947.108.7_653
Nehlig, A. (2018). Interindividual Differences in Caffeine Metabolism and Factors Driving Caffeine Consumption. Pharmacol. Rev. 70, 384–411. doi:10.1124/pr.117.014407
Newton, R., Broughton, L. J., Lind, M. J., Morrison, P. J., Rogers, H. J., and Bradbrook, I. D. (1981). Plasma and Salivary Pharmacokinetics of Caffeine in Man. Eur. J. Clin. Pharmacol. 21, 45–52. doi:10.1007/BF00609587
Oh, K. S., Park, S. J., Shinde, D. D., Shin, J. G., and Kim, D. H. (2012). High-sensitivity Liquid Chromatography-Tandem Mass Spectrometry for the Simultaneous Determination of Five Drugs and Their Cytochrome P450-specific Probe Metabolites in Human Plasma. J. Chromatogr. B Analyt Technol. Biomed. Life Sci. 895-896, 56–64. doi:10.1016/j.jchromb.2012.03.014
Park, G. J., Katelaris, P. H., Jones, D. B., Seow, F., Le Couteur, D. G., and Ngu, M. C. (2003). Validity of the 13c-Caffeine Breath Test as a Noninvasive, Quantitative Test of Liver Function. Hepatology 38, 1227–1236. doi:10.1053/jhep.2003.50475
Parsons, W. D., and Neims, A. H. (1978). Effect of Smoking on Caffeine Clearance. Clin. Pharmacol. Ther. 24, 40–45. doi:10.1002/cpt197824140
Patwardhan, R. V., Desmond, P. V., Johnson, R. F., and Schenker, S. (1980). Impaired Elimination of Caffeine by Oral Contraceptive Steroids. J. Lab. Clin. Med. 95, 603–608.
Perera, V., Gross, A. S., and McLachlan, A. J. (2010). Caffeine and Paraxanthine Hplc Assay for Cyp1a2 Phenotype Assessment Using Saliva and Plasma. Biomed. Chromatogr. 24, 1136–1144. doi:10.1002/bmc.1419
Perera, V., Gross, A. S., Xu, H., and McLachlan, A. J. (2011). Pharmacokinetics of Caffeine in Plasma and Saliva, and the Influence of Caffeine Abstinence on Cyp1a2 Metrics. J. Pharm. Pharmacol. 63, 1161–1168. doi:10.1111/j.2042-7158.2011.01326.x
Priller, S. (2005). Cytochrome P450 1a2 Phenotyping for Student Laboratories. Pharm. Education J. doi:10.1080/15602210500193219
Puri, B. K., Heard, C. R., and Monro, J. A. (2020). Is There a Sex Difference in Adult Salivary Clearance of Caffeine (1,3,7-Trimethylpurine-2,6-Dione)? J. Oral Biol. Craniofac. Res. 10, 20–22. doi:10.1016/j.jobcr.2020.01.010
Rasmussen, B. B., Brix, T. H., Kyvik, K. O., and Brøsen, K. (2002). The Interindividual Differences in the 3-demthylation of Caffeine Alias Cyp1a2 Is Determined by Both Genetic and Environmental Factors. Pharmacogenetics 12, 473–478. doi:10.1097/00008571-200208000-00008
Renner, E., Wietholtz, H., Huguenin, P., Arnaud, M. J., and Preisig, R. (1984). Caffeine: a Model Compound for Measuring Liver Function. Hepatology 4, 38–46. doi:10.1002/hep.1840040107
Rietveld, E. C., Broekman, M. M., Houben, J. J., Eskes, T. K., and van Rossum, J. M. (1984). Rapid Onset of an Increase in Caffeine Residence Time in Young Women Due to Oral Contraceptive Steroids. Eur. J. Clin. Pharmacol. 26, 371–373. doi:10.1007/BF00548769
Riley, R. D., Lambert, P. C., and Abo-Zaid, G. (2010). Meta-analysis of Individual Participant Data: Rationale, Conduct, and Reporting. BMJ 340, c221. doi:10.1136/bmj.c221
Rubin, T. M., Heyne, K., Luchterhand, A., Jan Bednarsch, J., W R Vondran, F., Polychronidis, G., et al. (2017). Kinetic Validation of the LiMAx Test during 10 000 Intravenous 13C-Methacetin Breath Tests. J. Breath Res. 12, 016005. doi:10.1088/1752-7163/aa820b
Sadek, P., Pan, X., Shepherd, P., Malandain, E., Carney, J., and Coleman, H. (2017). A Randomized, Two-Way Crossover Study to Evaluate the Pharmacokinetics of Caffeine Delivered Using Caffeinated Chewing Gum versus a Marketed Caffeinated Beverage in Healthy Adult Volunteers. J. Caffeine Res. 7, 125–132. doi:10.1089/jcr.2017.0025
Schmider, J., Brockmöller, J., Arold, G., Bauer, S., and Roots, I. (1999). Simultaneous Assessment of Cyp3a4 and Cyp1a2 Activity In Vivo with Alprazolam and Caffeine. Pharmacogenetics 9, 725–734. doi:10.1097/01213011-199912000-00007
Schrenk, D., Brockmeier, D., Mörike, K., Bock, K. W., and Eichelbaum, M. (1998). A Distribution Study of Cyp1a2 Phenotypes Among Smokers and Non-smokers in a Cohort of Healthy Caucasian Volunteers. Eur. J. Clin. Pharmacol. 53, 361–367. doi:10.1007/s002280050394
Scott, N. R., Chakraborty, J., and Marks, V. (1984). Determination of Caffeine, Theophylline and Theobromine in Serum and Saliva Using High-Performance Liquid Chromatography. Ann. Clin. Biochem. 21 (Pt 2), 120–124. doi:10.1177/000456328402100208
Scott, N. R., Stambuk, D., Chakraborty, J., Marks, V., and Morgan, M. Y. (1989). The Pharmacokinetics of Caffeine and its Dimethylxanthine Metabolites in Patients with Chronic Liver Disease. Br. J. Clin. Pharmacol. 27, 205–213. doi:10.1111/j.1365-2125.1989.tb05352.x
Scott, N. R., Stambuk, D., Chakraborty, J., Marks, V., and Morgan, M. Y. (1988). Caffeine Clearance and Biotransformation in Patients with Chronic Liver Disease. Clin. Sci. (Lond) 74, 377–384. doi:10.1042/cs0740377
Seng, K. Y., Fun, C. Y., Law, Y. L., Lim, W. M., Fan, W., and Lim, C. L. (2009). Population Pharmacokinetics of Caffeine in Healthy Male Adults Using Mixed-Effects Models. J. Clin. Pharm. Ther. 34, 103–114. doi:10.1111/j.1365-2710.2008.00976.x
Soto, J., Alsar, M. J., and Sacristan, J. A. (1995). Assessment of the Time Course of Drugs with Inhibitory Effects on Hepatic Metabolic Activity Using Successive Salivary Caffeine Tests. Pharmacotherapy 15, 781–784.
Spigset, O., Hägg, S., Söderström, E., and Dahlqvist, R. (1999). The Paraxanthine:caffeine Ratio in Serum or in Saliva as a Measure of Cyp1a2 Activity: when Should the Sample Be Obtained? Pharmacogenetics 9, 409–412. doi:10.1097/00008571-199906000-00019
Stader, F., Siccardi, M., Battegay, M., Kinvig, H., Penny, M. A., and Marzolini, C. (2019). Repository Describing an Aging Population to Inform Physiologically Based Pharmacokinetic Models Considering Anatomical, Physiological, and Biological Age-dependent Changes. Clin. Pharmacokinet. 58, 483–501. doi:10.1007/s40262-018-0709-7
Stille, W., Harder, S., Mieke, S., Beer, C., Shah, P. M., Frech, K., et al. (1987). Decrease of Caffeine Elimination in Man during Co-administration of 4-quinolones. J. Antimicrob. Chemother. 20, 729–734. doi:10.1093/jac/20.5.729
Sudsakorn, S., Bahadduri, P., Fretland, J., and Lu, C. (2020). 2020 Fda Drug-Drug Interaction Guidance: A Comparison Analysis and Action Plan by Pharmaceutical Industrial Scientists. Curr. Drug Metab. 21, 403–426. doi:10.2174/1389200221666200620210522
Syed, S. A., Kamimori, G. H., Kelly, W., and Eddington, N. D. (2005). Multiple Dose Pharmacokinetics of Caffeine Administered in Chewing Gum to normal Healthy Volunteers. Biopharm. Drug Dispos 26, 403–409. doi:10.1002/bdd.469
Tanaka, E., Ishikawa, A., Yamamoto, Y., Osada, A., Tsuji, K., Fukao, K., et al. (1993). Comparison of Hepatic Drug-Oxidizing Activity after Simultaneous Administration of Two Probe Drugs, Caffeine and Trimethadione, to Human Subjects. Pharmacol. Toxicol. 72, 31–33. doi:10.1111/j.1600-0773.1993.tb01335.x
Tanaka, S., Uchida, S., Inui, N., Takeuchi, K., Watanabe, H., and Namiki, N. (2014). Simultaneous Lc-Ms/ms Analysis of the Plasma Concentrations of a Cocktail of 5 Cytochrome P450 Substrate Drugs and Their Metabolites. Biol. Pharm. Bull. 37, 18–25. doi:10.1248/bpb.b13-00401
Tang-Liu, D. D., Williams, R. L., and Riegelman, S. (1983). Disposition of Caffeine and its Metabolites in Man. J. Pharmacol. Exp. Ther. 224, 180–185.
Tantcheva-Poór, I., Zaigler, M., Rietbrock, S., and Fuhr, U. (1999). Estimation of Cytochrome P-450 Cyp1a2 Activity in 863 Healthy Caucasians Using a Saliva-Based Caffeine Test. Pharmacogenetics 9, 131–144.
Thacker, S. B. (1988). Meta-analysis. A Quantitative Approach to Research Integration. JAMA 259, 1685–1689. doi:10.1001/jama.259.11.1685
Tian, D. D., Natesan, S., White, J. R., Paine, M. F., and Paine, M. F. (2019). Effects of Common Cyp1a2 Genotypes and Other Key Factors on Intraindividual Variation in the Caffeine Metabolic Ratio: An Exploratory Analysis. Clin. Transl Sci. 12, 39–46. doi:10.1111/cts.12598
Trang, J. M., Blanchard, J., Conrad, K. A., and Harrison, G. G. (1985). Relationship between Total Body Clearance of Caffeine and Urine Flow Rate in Elderly Men. Biopharm. Drug Dispos 6, 51–56. doi:10.1002/bdd.2510060107
Trang, J. M., Blanchard, J., Conrad, K. A., and Harrison, G. G. (1982). The Effect of Vitamin C on the Pharmacokinetics of Caffeine in Elderly Men. Am. J. Clin. Nutr. 35, 487–494. doi:10.1093/ajcn/35.3.487
Tricco, A. C., Lillie, E., Zarin, W., O'Brien, K. K., Colquhoun, H., Levac, D., et al. (2018). PRISMA Extension for Scoping Reviews (PRISMA-ScR): Checklist and Explanation. Ann. Intern. Med. 169, 467–473. doi:10.7326/M18-0850
Tripathi, A., Tiwari, B., Patil, R., Khanna, V., and Singh, V. (2015). The Role of Salivary Caffeine Clearance in the Diagnosis of Chronic Liver Disease. J. Oral Biol. Craniofac. Res. 5, 28–33. doi:10.1016/j.jobcr.2014.12.003
Turpault, S., Brian, W., Van Horn, R., Santoni, A., Poitiers, F., Donazzolo, Y., et al. (2009). Pharmacokinetic Assessment of a Five-Probe Cocktail for Cyps 1a2, 2c9, 2c19, 2d6 and 3a. Br. J. Clin. Pharmacol. 68, 928–935. doi:10.1111/j.1365-2125.2009.03548.x
Urry, E., Jetter, A., and Landolt, H. P. (2016). Assessment of Cyp1a2 Enzyme Activity in Relation to Type-2 Diabetes and Habitual Caffeine Intake. Nutr. Metab. (Lond) 13, 66. doi:10.1186/s12986-016-0126-6
Wahlländer, A., Mohr, S., and Paumgartner, G. (1990). Assessment of Hepatic Function. Comparison of Caffeine Clearance in Serum and Saliva during the Day and at Night. J. Hepatol. 10, 129–137. doi:10.1016/0168-8278(90)90041-o
Walther, H., Banditt, P., and Köhler, E. (1983). Significance of Caffeine Values in Serum, Saliva and Urine-Ddetermination of Pharmacokinetic Data by Non-invasive Methods in Psychopharmacologic Studies. Pharmacopsychiatria 16, 166–170. doi:10.1055/s-2007-1019492
Wang, T., Kleber, G., Stellaard, F., and Paumgartner, G. (1985). Caffeine Elimination: a Test of Liver Function. Klin Wochenschr 63, 1124–1128. doi:10.1007/BF02291094
White, J. R., Padowski, J. M., Zhong, Y., Chen, G., Luo, S., Lazarus, P., et al. (2016). Pharmacokinetic Analysis and Comparison of Caffeine Administered Rapidly or Slowly in Coffee Chilled or Hot versus Chilled Energy Drink in Healthy Young Adults. Clin. Toxicol. 54, 308–312. doi:10.3109/15563650.2016.1146740
Yamazaki, T., Desai, A., Goldwater, R., Han, D., Howieson, C., Akhtar, S., et al. (2017). Pharmacokinetic Effects of Isavuconazole Coadministration with the Cytochrome P450 Enzyme Substrates Bupropion, Repaglinide, Caffeine, Dextromethorphan, and Methadone in Healthy Subjects. Clin. Pharmacol. Drug Dev. 6, 54–65. doi:10.1002/cpdd.281
Yang, X., Zhang, B., Molony, C., Chudin, E., Hao, K., Zhu, J., et al. (2010). Systematic Genetic and Genomic Analysis of Cytochrome P450 Enzyme Activities in Human Liver. Genome Res. 20, 1020–1036. doi:10.1101/gr.103341.109
Yesair, D. W., Branfman, A. R., and Callahan, M. M. (1984). Human Disposition and Some Biochemical Aspects of Methylxanthines. Prog. Clin. Biol. Res. 158, 215–233.
Yubero-Lahoz, S., Pardo, R., Farre, M., Mathuna, B. Ó., Torrens, M., Mustata, C., et al. (2012). Changes in Cyp1a2 Activity in Humans after 3,4-methylenedioxymethamphetamine (Mdma, Ecstasy) Administration Using Caffeine as a Probe Drug. Drug Metab. Pharmacokinet. 27, 605–613. doi:10.2133/dmpk.dmpk-12-rg-032
Zanger, U. M., and Schwab, M. (2013). Cytochrome P450 Enzymes in Drug Metabolism: Regulation of Gene Expression, Enzyme Activities, and Impact of Genetic Variation. Pharmacol. Ther. 138, 103–141. doi:10.1016/j.pharmthera.2012.12.007
Zylber-Katz, E., Granit, L., and Levy, M. (1984). Relationship between Caffeine Concentrations in Plasma and Saliva. Clin. Pharmacol. Ther. 36, 133–137. doi:10.1038/clpt.1984.151
Keywords: caffeine, pharmacokinetics, smoking, oral contraceptives, drug-drug interactions, drug-disease interactions, CYP1A2, liver function test
Citation: Grzegorzewski J, Bartsch F, Köller A and König M (2022) Pharmacokinetics of Caffeine: A Systematic Analysis of Reported Data for Application in Metabolic Phenotyping and Liver Function Testing. Front. Pharmacol. 12:752826. doi: 10.3389/fphar.2021.752826
Received: 03 August 2021; Accepted: 03 December 2021;
Published: 25 February 2022.
Edited by:
Feng Li, Baylor College of Medicine, United StatesReviewed by:
Duygu Türközü Agagündüz, Gazi University, TurkeyAmelia Filippelli, University of Salerno, Italy
Copyright © 2022 Grzegorzewski, Bartsch , Köller and König. This is an open-access article distributed under the terms of the Creative Commons Attribution License (CC BY). The use, distribution or reproduction in other forums is permitted, provided the original author(s) and the copyright owner(s) are credited and that the original publication in this journal is cited, in accordance with accepted academic practice. No use, distribution or reproduction is permitted which does not comply with these terms.
*Correspondence: Jan Grzegorzewski, grzegorj@hu-berlin.de