- 1Division of Pharmacogenomics and Personalized Medicine, Department of Pathology, Faculty of Medicine Ramathibodi Hospital, Mahidol University, Bangkok, Thailand
- 2Laboratory for Pharmacogenomics, Somdech Phra Debaratana Medical Center (SDMC), Ramathibodi Hospital, Bangkok, Thailand
- 3Department of Pharmacy, University of Rajshahi, Rajshahi, Bangladesh
- 4Department of Pathology, School of Medicine, Mae Fah Luang University, Chiang Rai, Thailand
- 5Structural and Computational Biology Research Unit, Department of Biochemistry, Faculty of Science, Chulalongkorn University, Bangkok, Thailand
- 6Program in Bioinformatics and Computational Biology, Graduate School, Chulalongkorn University, Bangkok, Thailand
- 7Pharmacogenomics and Precision Medicine, The Preventive Genomics and Family Check-up Services Center, Bumrungrad International Hospital, Bangkok, Thailand
- 8MRC Centre for Drug Safety Science, Department of Pharmacology and Therapeutics, Institute of Systems, Molecular and Integrative Biology, University of Liverpool, Liverpool, United Kingdom
Many drugs are being administered to tackle coronavirus disease 2019 (COVID-19) pandemic situations without establishing clinical effectiveness or tailoring safety. A repurposing strategy might be more effective and successful if pharmacogenetic interventions are being considered in future clinical studies/trials. Although it is very unlikely that there are almost no pharmacogenetic data for COVID-19 drugs, however, from inferring the pharmacokinetic (PK)/pharmacodynamic(PD) properties and some pharmacogenetic evidence in other diseases/clinical conditions, it is highly likely that pharmacogenetic associations are also feasible in at least some COVID-19 drugs. We strongly mandate to undertake a pharmacogenetic assessment for at least these drug–gene pairs (atazanavir–UGT1A1, ABCB1, SLCO1B1, APOA5; efavirenz–CYP2B6; nevirapine–HLA, CYP2B6, ABCB1; lopinavir–SLCO1B3, ABCC2; ribavirin–SLC28A2; tocilizumab–FCGR3A; ivermectin–ABCB1; oseltamivir–CES1, ABCB1; clopidogrel–CYP2C19, ABCB1, warfarin–CYP2C9, VKORC1; non-steroidal anti-inflammatory drugs (NSAIDs)–CYP2C9) in COVID-19 patients for advancing precision medicine. Molecular docking and computational studies are promising to achieve new therapeutics against SARS-CoV-2 infection. The current situation in the discovery of anti-SARS-CoV-2 agents at four important targets from in silico studies has been described and summarized in this review. Although natural occurring compounds from different herbs against SARS-CoV-2 infection are favorable, however, accurate experimental investigation of these compounds is warranted to provide insightful information. Moreover, clinical considerations of drug–drug interactions (DDIs) and drug–herb interactions (DHIs) of the existing repurposed drugs along with pharmacogenetic (e.g., efavirenz and CYP2B6) and herbogenetic (e.g., andrographolide and CYP2C9) interventions, collectively called multifactorial drug–gene interactions (DGIs), may further accelerate the development of precision COVID-19 therapies in the real-world clinical settings.
1 Introduction for Pharmacogenetics of COVID-19 Treatment
At the end of 2019, a novel coronavirus, severe acute respiratory syndrome coronavirus 2 (SARS-CoV-2) started as an emerging pathogen for humans, first appeared in Wuhan, China, in December 2019. This novel virus causes coronavirus disease 2019 (COVID-19), named by the WHO on 11 February 2020, and it has been characterized as a pandemic on 11 March 2020. COVID-19 has become the leading cause of death globally, resulting in huge economic and social disruption internationally (Hodgson et al., 2021). As of 10 September 2021 as declared by the WHO, over 223 million confirmed cases of SARS-CoV-2 infection have been detected globally in which ∼4.6 million deaths occurred (WHO, 2021). It is alarming that still now considerably a large number of patients are dying due to COVID-19. The existence of this pandemic virus had been confirmed in over 200 countries or territories, indicating that corona virus was exponentially spread out throughout the world.
One of the leading causes of morbidity and mortality might be the adverse drug reactions (ADRs) associated with current medications administered for the management of COVID-19 since the mortality rate was significantly higher in COVID-19 patients with multiple comorbidities and particularly in older patients (Manjaly Thomas et al., 2020; Ramírez et al., 2020; Biswas et al., 2021a; Falcão et al., 2021; Melo et al., 2021; Rezaee et al., 2021). Polypharmacy is highly predictable in multiple comorbid patients, and also, age-related degradation of organ function in older patients is placing them highly vulnerable to drug–drug interactions (DDIs) and consequently the most notorious ADRs or toxicities of the COVID-19 therapeutics. A recent pharmacovigilance study conducted in Spain reported the 4.75-fold higher incidence of severe ADRs in the COVID-19 patients compared to non-COVID-19 patients, in which the prevalence of severe ADRs was the highest with tocilizumab (59.8%) followed by dexketoprofen (13.9%), azithromycin (8.4%), dexamethasone (7.6%), lopinavir–ritonavir (7.4%), and chloroquine (CQ)/hydroxychloroquine (HCQ) (6.9%) (Ramírez et al., 2020). Another pharmacovigilance study conducted in Brazil with 402 COVID-19 patients indicated that chloroquine (CQ) (OR = 5.4; 95% CI: 1.9–15.6) and HCQ (OR = 2.1; 95% CI: 1.2–3.6) were the only culprit drugs associated with severe ADRs (Melo et al., 2021). A prospective observational study identified a total of 102 ADRs in 149 COVID-19 patients where the incidence of ADRs was significantly higher in patients who have taken HCQ than in the patients who have taken remdesivir (RDV) (47.5 vs. 12.5%; p < 0.001), as evidenced recently (Falcão et al., 2021). This is consistent with a predictive study showing that at least 329 DDIs are feasible in patients taking HCQ, and at the very least, 29 severe DDIs were identified from different reputed international interaction resources, predicted to cause severe toxicity of HCQ (Biswas and Roy, 2021). A hospital-based pharmacovigilance study conducted in China identified ∼38% ADRs in COVID-19 patients, where drug-induced gastrointestinal disorders were 23% and liver system disorders were ∼14%. These ADRs were mainly associated with the use of lopinavir/ritonavir (∼64%) and umifenovir (∼18%). Multivariate logistic analysis indicated that the number of drugs used while COVID-19 patients were staying in the hospital was one of the strongest independent risk factors for these ADRs (OR: 3.17; 95% CI 1.60–6.27; p = 0.001), as reported in this observational study (Sun et al., 2020).
Although there are no specific therapeutic recommendations for treating COVID-19, however, many off-label drugs are being currently administered for the management of COVID-19 and severe ADRs; for example, QT prolongation, cardiac arrhythmias, thrombosis, retinopathy, hepatotoxicity, and increased risk of infection due to DDIs are feasible in these patients as evidenced and suggested elsewhere (Biswas et al., 2020a; Lemaitre et al., 2020; Manjaly Thomas et al., 2020; Ramírez et al., 2020; Sun et al., 2020; Biswas and Roy, 2021; Falcão et al., 2021; Melo et al., 2021; Rezaee et al., 2021).
While it is increasingly true of DDIs for COVID-19 therapeutics, it is likely that the cytochrome P450 (CYP) enzymes or transporter proteins affecting the pharmacokinetics (PK) or pharmacodynamic (PD) properties were mostly involved in the reported and predicted DDIs of drugs used in the treatment of COVID-19. However, the genetic variants modulating the PK/PD profiles of COVID-19 drugs regulating the safety or effectiveness are not clinically studied yet, posing serious scarcity of pharmacogenomic data in the literature.
The PK and PD properties of the COVID-19 drugs are very potential factors to explore the pharmacogenetics association study; however, it appears that drug-developing authorities and scientists did not consider the pharmacogenetics interference in drug response variability, which could affect either the safety/effectiveness of COVID-19 drugs or the severity of COVID-19 progression. In this review, we will discuss in detail the pharmacogenetics of COVID-19 therapeutics with a particular focus on drugs targeting SARS-CoV-2 life cycle, drug–drug interactions (DDIs), and drug–herb interactions (DHIs) potentially affecting the pharmacogenetic interventions. We will also discuss some of the genetic variants potentially affecting the severity of COVID-19 progression.
2 Virology of SARS-CoV-2 and its Entry Into Human Cells
SARS-CoV-2 is a positive-sense, single-stranded RNA-enveloped virus in the Betacoronavirus genus (Attaway et al., 2021). Bats and pangolins may be the animal hosts of SARS-CoV-2 as there is a >90% gene homology to SARS-CoV-2 found to infect humans (Hu et al., 2021a). Currently, it remains unclear if SARS-CoV-2 was directly transferred from bat/pangolins to humans or an intermediate host was required for transmission. In light of the current pandemic, researchers first compared SARS-CoV-2 with the previous endemic SARS-CoV (2002–2003) and MERS-CoV (2012) (da Costa et al., 2020). SARS-CoV-2 propagates and migrates down the respiratory tract along the conducting airways. The entry process of SARS-CoV-2 into host cells is via the binding of the S protein to the ACE2 receptor (Figure 1).
The virion releases its RNA. Some RNA is translated into proteins by the host cell’s machinery. Proteins and RNA are assembled into a new virion in the Golgi and released. ACE2 receptors are highly expressed in the upper respiratory tract of humans (Lan et al., 2020). Proteolytic cleavage of the S protein by serine proteases including transmembrane protease serine 2 (TMPRSS2), cathepsin L, and furin is required for binding to the ACE2 receptor (Wang et al., 2020b). In the lower respiratory tract, type II pneumocytes and alveolar macrophages also express ACE2 receptors and can be infected, and release several chemokines/cytokines. Once the virus attaches to the host cell receptors, it undergoes endocytosis, viral maturation, replication, and release of more virus within the cytoplasm of the host cell. SARS-CoV-2 infection begins with viral replication and partially avoids host recognition during the initial infection and before the host innate response is enabled (Bergmann and Silverman, 2020).
Angiotensin-converting enzyme 2 (ACE2) functions as a master regulator of the renin-angiotensin system (RAS) mainly by converting Ang (angiotensin) I and Ang II into Ang 1–9 and Ang 1–7, respectively. The ACE2 system is a critical protective pathway against heart failure, myocardial infarction, and hypertension, and against lung disease and diabetes mellitus. ACE2 is widely expressed, including in the lungs, cardiovascular system, gut, kidneys, central nervous system, and adipose tissue. ACE2 has recently been identified as the SARS-CoV-2 receptor (Kuhn et al., 2004). The loss of ACE2 function following binding by SARS-CoV-2 is driven by endocytosis and activation of proteolytic cleavage. Ang II levels elevate with increased activity of angiotensin 1 receptors (AT1R) at the cost of ACE2/Ang 1–7-driven pathways, leading to adverse fibrosis, hypertrophy, increased reactive oxygen species (ROS), vasoconstriction, and gut dysbiosis. ADAM17 (a disintegrin and metalloproteinase 17)-mediated proteolytic cleavage of ACE2 is upregulated by endocytosed SARS-CoV-2 spike proteins. The activation of the AT1R by elevated Ang II levels also further increases ADAM17 activity. ADAM17 correspondingly also cleaves its primary substrate releasing soluble TNF-α (tumor necrosis factor-α) into the extracellular region where it has auto- and paracrine functionality. TNF-α activation of its tumor necrosis factor receptor (TNFR) represents a third pathway elevating ADAM17 activity (Gheblawi et al., 2020). TNF-α along with systemic cytokines released due to SARS-CoV-2 infection can lead to a cytokine storm.
3 Immune Response, Pathogenesis, and Clinical Manifestation of COVID-19
The cells of the airway epithelium are the first line of defense (innate immune system), providing a mechanical barrier (mucociliary escalator) that expels particles and pathogens via cilia, mucus, and induced coughing. This barrier includes cells of the pulmonary epithelium, alveolar macrophages (AMs), and dendritic cells (DCs). The AMs and DCs express pattern recognition receptors (PRRs), including Toll-like receptors (TLRs) and RIG-I-like receptors (RLRs), that can detect molecules from pathogens (pathogen-associated molecular patterns—PAMPs) or molecules released from damaged cells (damage or danger-associated molecular patterns—DAMPs) (Figure 2–I).
Upon recognition, these sensors recruit the adaptor proteins, MyD88 and MAVS, respectively, and induce downstream signaling. Ultimately, this leads to the activation of the transcription factors, IRF3/7 and NF-κB, and the subsequent production of type I interferons (IFN-α and IFN-β) and pro-inflammatory cytokines (e.g., IL-6 and TNF-α), respectively. Additionally, the virus is thought to activate the inflammasome sensor, NLRP3, resulting in the secretion of the highly inflammatory cytokine IL-1β and the induction of pyroptosis, an inflammatory form of cell death (Lim et al., 2016).
T cells and B cells are activated (adaptive immune system) by antigen presentation and cytokines from DCs and AMs, and activation of the complement system. IL-6 promotes the differentiation to cytotoxic T cells, helper T cells (Th), and plasma cells. IL-1β promotes the differentiation of Th17, which functions by stimulating neutrophil recruitment and inflammation (Figure 2–II). Cytotoxic T cells play a crucial role in SARS-CoV-2 clearance due to their ability to selectively eliminate virus-infected cells by inducing apoptosis via ligands such as Fas ligand (FasL) and tumor necrosis factor-related apoptosis-inducing ligand (TRAIL), and perforin/granzyme-mediated pathway (Ranasinghe et al., 2016; Huang et al., 2019). Th1 helps in the activation of cytotoxic T cells. Th2 activates B cells to produce antibodies and become plasma cells. These antibodies contribute to SARS-CoV-2 clearance. There are memory T and B cells that can help against the recurrent infection (Figure 1).
Neutrophils are attracted by chemokines/cytokines swarm to the site of infection. Subsequently, activated neutrophils undergo degranulation and (neutrophil extracellular traps) NET formation, releasing intracellular DAMPs, DNA, histones, and neutrophil elastase that activates the PRRs of surrounding immune and non-immune cells to induce cytokine secretion (Figure 2–III). Neutrophils and NETs drive necro-inflammation in COVID-19 (Barnes et al., 2020). The extracellular DNA released by NETs activates platelets, and aggregated NETs provide a scaffold for binding of erythrocytes and activated platelets that promote thrombus formation (Mozzini and Girelli, 2020).
In a later phase of SARS-CoV-2 infection, the complement system will be triggered via antibodies bound to the virus (Noris et al., 2020). C3 can be converted into C3a and C3b. C3b mediates pathogen opsonization and activates the conversion of C5 into C5a and C5b. C5b mediates the formation of the membrane attack complex (MAC), which leads to cell lysis. C3a and C5a promote immune cell recruitment to the site of infection (Figure 2–IV).
Excessive cytokines produced by macrophages and DCs, that is, IL-1β, IFN-I, CXCL10, CXCL11, IL-6, IP-10, and TNF-α, are called cytokine storm (Ahmed-Hassan et al., 2020) (Figure 2–V). Cytokine storm and C5a lead to the influx of immune cells (e.g., granulocytes, monocytes, T cells, B cells, and NK cells) into the infected site (Wang et al., 2015) (Figure 2–VI). The overwhelming infiltrate of immune cells causes excessive pulmonary inflammation (severe pneumonia) with destructive effects on human tissue, resulting in destabilization of endothelial cell to cell interactions, damage of vascular barrier, capillary damage, diffuse alveolar damage (DAD), pulmonary fibrosis, systemic inflammation, hyperferritinemia, hemodynamic instability, and multi-organ failure, and if left untreated, it leads to death (Ackermann et al., 2021; Chen and Pan, 2021) (Figure 2–VII). Acute respiratory distress syndrome (ARDS), as a result of DAD, leads to low oxygen saturation levels and is a major cause of mortality in COVID-19. SARS-CoV-2 also can infect the endothelial cells, causing endothelial injury, endotheliitis, and microthrombus formation in several organs, especially in alveolar capillary (Varga et al., 2020). The electron microscopy shows new vessel growth through a mechanism of intussusceptive angiogenesis, especially in patients with a long duration of hospitalization (Ackermann et al., 2020). These microangiopathies could be the factors, which are worsening the ARDS. Although the exact mechanism of ARDS in COVID-19 patients is not fully understood, the excessive production of pro-inflammatory cytokines is considered to be one of the major contributing factors (Chen et al., 2020).
A common characteristic of SARS-CoV-2 is asymptomatic transmission, which is likely the cause of rampant spread and transmission. Given SARS-CoV-2 entry is primarily via the respiratory tract, upper and lower respiratory tract involvement is the most common manifestation. About one-third of patients hospitalized with SARS-CoV-2 infection meet the criteria for acute respiratory distress syndrome (Attaway et al., 2021). The main clinical manifestations of COVID-19 are fever (90% or more), cough (around 75%), and dyspnea (up to 50%) (Jiang et al., 2020). A small but significant subset has gastrointestinal symptoms. Preliminary estimates of case fatality, likely to fall as better early diagnostic efforts come into play, are about 2%, mostly due to ARDS, acute kidney injury, and myocardial injury (Jiang et al., 2020). The clinical manifestations are summarized in Figure 3 as described elsewhere (Huang et al., 2020; Jiang et al., 2020; Alizadehsani et al., 2021).
4 Treatments of COVID-19
It is well recognized that COVID-19 has four stages of progression in which the first stage is initiated by upper respiratory tract infection. In the second stage, the symptoms of dyspnea and pneumonia appeared. In the third stage of COVID-19, cytokine storm followed by the hyperinflammatory state predominantly worsens the clinical scenario. The final stage of COVID-19 progression is either death or recovery. While as many as 800 clinical trials are ongoing and some of these have already been completed worldwide, currently, no treatment was found to be clinically effective to act selectively against the SARS-CoV-2 infection (Becker, 2020; Stasi et al., 2020). Currently, different therapeutics are being applied to treat moderate-to-severe COVID-19 patients considering the pathological features and various stages of COVID-19, of which repurposed drugs are being used predominantly (Becker, 2020; Song et al., 2020; Stasi et al., 2020; Gavriatopoulou et al., 2021).
5 Repurposing Drugs for COVID-19: Concept and Mechanism of Action
Gilead Sciences first developed remdesivir (RDV) in 2017 for the treatment of infection caused by Ebola virus. In the United States, South Korea, and China, RDV was clinically evaluated in moderate-to-severe COVID-19 patients through several phase 3 clinical trials (Gavriatopoulou et al., 2021). Based on reviewing current evidence from randomized, double-blinded, placebo-controlled clinical trials, the FDA has been persuaded to believe the potential benefits of RDV over potential risks for the treatment of severe hospitalized COVID-19 patients (FDA, 2020). Henceforth, the FDA issued an Emergency Use Authorization (EUA) for emergency use of RDV for the treatment of hospitalized severe COVID-19 adult and children patients where severity of COVID-19 has been defined as SpO2 ≤ 94% on room air, requiring supplemental oxygen, mechanical ventilation, or extracorporeal membrane oxygenation (FDA, 2020). It is reported that RDV can inhibit RNA-dependent polymerase and may therefore be effective in the treatment of SARS-CoV-2 infection. It is actually a phosphoramidate prodrug having broad-spectrum activity against various viruses, for example, Paramyxoviridae, Filoviridae, Pneumoviridae, and Orthocoronavirinae, that is, SARS-CoV and Middle East respiratory syndrome coronavirus (MERS-CoV), as described elsewhere (Sheahan et al., 2017; Martinez, 2020; Gavriatopoulou et al., 2021). Although the FDA has recommended emergency use of RDV in severe COVID-19 patients, however, the safety and efficacy of RDV in COVID-19 patients as evidenced in multiple recent meta-analyses are controversial and inconsistent (Angamo et al., 2021; Elsawah et al., 2021; Tao et al., 2021).
Chloroquine (CQ) and hydroxychloroquine (HCQ) were included on the essential lists of medications of the World Health Organization (WHO) and used for several decades for the prophylaxis of malaria. They are also used for the treatment of rheumatoid arthritis, systemic lupus erythematosus, Sjogren’s syndrome, and post-Lyme’s disease arthritis (Shippey et al., 2018; Schrezenmeier and Dörner, 2020; Gavriatopoulou et al., 2021). Although HCQ and CQ may exhibit anti-inflammatory, immunomodulating, anti-infective, antithrombotic, and metabolic effects, however, they can also inhibit SARS-CoV-2 host entry by binding to the host cell angiotensin-converting enzyme-2 (ACE2) receptor, thereby impairing SARS-CoV-2 spike protein recognition (Becker, 2020; Fantini et al., 2020). These drugs act by blocking 2019-nCoV entry into host cells by inhibiting glycosylation of host receptors, proteolytic processing, and endosomal acidification (Sanders et al., 2020). Several clinical trials had assessed the safety and efficacy of CQ/HCQ in COVID-19 patients. Based on primary results from some clinical trials, the U.S. FDA had approved emergency use of CQ/HCQ for the treatment of COVID-19 patients on 28 March 2020. Later, the U.S. FDA issued a cautioning statement against CQ/HCQ use in COVID-19 patients due to serious cardiac toxic effects, for example, arrhythmias on April 24, 2020. Finally, on 15 June 2020, the FDA revoked the EUA use of CQ/HCQ as a potential COVID-19 therapy after accumulating negative data from clinical trials (Gavriatopoulou et al., 2021).
Lopinavir (LPV) and ritonavir (RTV) are HIV protease inhibitors and used in combination with or without other antiviral drugs for the treatment of human immunodeficiency virus (HIV-1)-infected patients older than 2 years. Due to its inhibiting nature of viral DNA-dependent RNA polymerase, either combination of LPV/RTV or alone has been recommended for the treatment of COVID-19 patients; however, the results of clinical trials are not favoring the clinical outcomes and are limited in use nowadays (Cao et al., 2020; Gavriatopoulou et al., 2021). Although LPV/RTV was suggested primarily by the National Health Commission (NHC) of China as an antiviral therapy in COVID-19 patients, however, it is not recommended by the U.S. National Institute of Health (NIH) due to lack of proven clinical effectiveness in these patients (Song et al., 2020).
Arbidol was first marketed in Russia and China as a synthetic antiviral drug for the treatment of seasonal influenza. A previous study demonstrated that arbidol was broadly effective against some other viruses including SARS-CoV and was generally well tolerated in treating these viruses (Gavriatopoulou et al., 2021). Initially, the in vitro test found arbidol to be an effective inhibitor of SARS-CoV-2 infection, and it was therefore recommended by the China’s NHC guide on COVID-19 treatment option. It appeared that arbidol was found ineffective against SARS-CoV-2 infection in ongoing clinical studies, although it had significant limitations in study design and sample size in these studies (Song et al., 2020).
Favipiravir was first approved in Japan for the treatment of influenza and was later found effective against Ebola virus infection also. Although several clinical trials were undertaken in China, Japan, Canada, and Russia evaluating the safety and efficacy of favipiravir alone or in combination with other antivirals against SARS-CoV-2 infection, however, the results were not persuading the clinicians for further considerations in treating COVID-19 patients with favipiravir (Gavriatopoulou et al., 2021).
Originally, darunavir/cobicistat was developed for the treatment of HIV-1 infection. Due to its protease inhibiting activity, the clinical trial had assessed the safety and efficacy of darunavir/cobicistat in SARS-CoV-2 infection and found that darunavir/cobicistat was not effective in the treatment of COVID-19 patients (Gavriatopoulou et al., 2021).
It is worth mentioning here that there are no supporting data from clinical trials that could favor the use of any HIV protease inhibitors to treat COVID-19 patients. Followingly, recently, the NIH panel for COVID-19 treatment guidelines did not recommend the use of any HIV protease inhibitors in the treatment of COVID-19 infection due to lack of clear clinical benefit in these patients (Amanat and Krammer, 2020; Gavriatopoulou et al., 2021).
Atazanavir (ATV) was discovered early in the 2000s as an antiretroviral drug for treating HIV instead of LPV/RTV because of lesser side effects of this drug. Evidence from in silico and in vitro studies suggests that by inhibiting viral major protease, ATV would inhibit SARS-CoV-2 replication even better than LPV/RTV (Fintelman-Rodrigues et al., 2020; Stasi et al., 2020; Alavian et al., 2021).
As part of highly active antiretroviral therapy (HAART), efavirenz (EVZ) and nevirapine are mainly used in the treatment of HIV/AIDS; however, these drugs could also be used for treating SARS-CoV-2 infection because of their ability to inhibit viral RNA-dependent RNA polymerase (RdRp) (Nastri, et al., 2020). Also, nelfinavir mesylate (NFV) being an antiretroviral drug may have potential efficacy against SARS-CoV-2 infection. Recent studies suggest that it can inhibit spike protein (S) medicated cell fusion of SARS-CoV-2 and may eventually block the transfer and cell-to-cell spread of SARS-CoV-2 (Yousefi et al., 2021).
Ribavirin is a nucleoside analog and was found effective against many RNA viruses, including SARS-CoV and MERS-CoV. It mainly inhibits RNA polymerase and synthesis of viral protein. Ribavirin was widely used with or without steroids against SARS infection, outbreak in 2003. Although intravenous ribavirin in combination with LPV/RTV or interferon was suggested by China’s NHC for the treatment of patients with COVID-19, it is not recommended by the NIH (Song et al., 2020).
It has well established that severe COVID-19 patients are generally associated with an increased cytokine-release syndrome, which further elevated interleukin-6 (IL-6). Tocilizumab, an IL-6 receptor antagonist, is commonly used for the treatment of rheumatoid arthritis and patients having cytokine-release syndrome (Takahashi et al., 2020). It was authorized by the Agenzia Italiana del Farmaco (AIFA), the Italian Medicines Agency, to investigate its safety and efficacy in COVID-19 patients. However, a recent clinical trial did not find significant clinical benefits, for example, reduced mortality or increased survival rate in using this drug (Stasi et al., 2020; Salama et al., 2021).
Molnupiravir, a ribonucleoside prodrug of N-hydroxycytidine (NHC), was originally developed as a potent inhibitor of respiratory syncytial virus (RSV), influenza B viruses, and influenza A viruses (IAVs) of human, avian, and swine origins (Yoon et al., 2018; Jayk Bernal et al., 2021). Later, it was found to be effective as a first oral and direct-acting anti-SARS-CoV-2 agent in both in vitro and in vivo studies (Jayk Bernal et al., 2021; Kabinger et al., 2021). When molnupiravir in the form of NHC prodrug is administered orally, it circulates systemically and is phosphorylated intracellularly to NHC triphosphate, an active form of molnupravir. This active form subsequently interferes in viral replication by inducing RNA mutagenesis through incorporation of 5′-monophosphate metabolite into viral RdRp. The active compound of molnupiravir, NHC 5′-triphosphate (NHC-TP), increases “G” to “A” and “C” to “U” transition mutations in replicating coronaviruses that lead to increased antiviral effects (Ehteshami et al., 2017; Gordon et al., 2021; Jayk Bernal et al., 2021; Kabinger et al., 2021).
Another important treatment strategy against SARS-CoV-2 infection was to develop selective targets that may neutralize monoclonal antibodies (mAbs) since previous studies reported a large number of antibodies by disturbing the receptors of either SARS-CoV or MERS coronavirus (MERS-CoV) that showed neutralization activities (Du et al., 2009; de Wit et al., 2016). Generation of virus-neutralizing antibodies or neutralizing mAbs (from the B cells of convalescent patients or humanized mice sources) are being developed from B cells of convalescent patients or humanized mice against viral infections, by targeting the receptor-binding domain (RBD) of the spike (S) protein of SARS-CoV-2 by some mechanisms—directly through triggering the phagocytosis by binding to virons or infected cells—and also by two different types of distance mechanisms in an antibody-dependent enhancement (ADE) process:
a) ADE via enhanced infection-expanded viral disease and replication by viral uptake into Fc gamma receptor IIa (FcγRIIa)-expressing phagocytic cells.
b) ADE via enhanced immune activation by excessive antibody Fc-mediated effector functions or immune complex formation in an antibody-dependent manner (Lee et al., 2020; Taylor et al., 2021).
To date, seven mAb neutralizing drugs including bamlanivimab, etesevimab, casirivimab, imdevimab, sotrovimab, cilgavimab, and tixagevimab either as monotherapy or as combination therapy have been approved or received EUAs from the U.S. FDA for the treatment of COVID-19 (Agarwal et al., 2020; Shi et al., 2020a; Zhang et al., 2021c; Nathan et al., 2021). All repurposed drugs with their mechanism of actions against SARS-CoV-2 infections are shown in Table 1.
Also, the different drugs acting on different phases of SARS-CoV-2 life cycle are shown in Figure 4
6 Potential Risk of COVID-19 and Supportive Treatments
6.1 Anticoagulants
One of the most emerging prevalent risks associated with SARS-CoV-2 severe infection is venous thromboembolism (VTE), particularly pulmonary embolism (PE). The reported prevalence of VTE is ∼25–30% in severe COVID-19 patients, which is considerably higher than that of other viral infections (Bikdeli et al., 2020; Klok et al., 2020; Gavriatopoulou et al., 2021). Regulatory-approved drugs such as direct oral anticoagulants (DOACs), for example, rivaroxaban, apixaban, and dabigatran, and vitamin K antagonists, for example, warfarin, could be used to minimize the risk of VTE in severe COVID-19 patients. These supportive therapies should be continued for at least 3 months if VTE is suspected or confirmed in COVID-19 patients (Bikdeli et al., 2020; Gavriatopoulou et al., 2021).
6.2 Angiotensin-Converting Enzyme Inhibitors/Angiotensin Receptor Blockers
Initially, there was a great concern whether angiotensin-converting enzyme inhibitors (ACEIs)/angiotensin receptor blockers (ARBs) should or should not be continued in COVID-19 patients, especially with hypertension and diabetes mellitus. This is because SARS-CoV-2 binds to the ACE2 receptor to gain entry into the host cells. However, recent meta-analyses established that COVID-19 patients taking ACEIs/ARBs were not associated with an increased risk of mortality compared to those not taking ACEIs/ARBs. The risk of composite severe clinical manifestations was not significantly different between the positive patients with or without ACEI/ARB users and also found evidence of beneficial effects for using ACEIs/ARBs especially in hypertensive COVID-19 patients. These results strongly suggest continuing with renin angiotensin aldosterone system (RAAS) inhibitors during the COVID-19 pandemic (Baral et al., 2020; Biswas and Kali, 2021a; Wang et al., 2021).
6.3 Antiplatelets
A recent meta-analysis indicated that the risk of acute stroke was significantly higher in severe COVID-19 patients than in non-severe COVID-19 patients (RR = 4.18, 95% CI: 1.7–10.25; p = 0.002) (Siepmann et al., 2021). Clinical studies also showed that heart failure/myocardial infarction (MI) is prevalently higher in severe COVID-19 patients. The P2Y12 receptor antagonists, for example, clopidogrel, prasugrel, and ticagrelor, are widely used as first-line therapy in patients with stroke or coronary artery disease (CAD) (Bikdeli et al., 2020; Sivaloganathan et al., 2020; Zhao et al., 2021).
6.4 Antifibrotics
Idiopathic pulmonary fibrosis is one of the major risk factors associated with the severity of COVID-19. Magnitude and intensity of lung fibrosis may increase the risk for severe clinical outcomes in patients with COVID-19. It is proposed that antifibrotics such as pirfenidone and nintedanib may reduce the severity of SARS-CoV-2 infection and might be an integral part of COVID-19 therapeutics (Bikdeli et al., 2020; Gavriatopoulou et al., 2021).
6.5 Systemic Corticosteroids
During severe acute respiratory syndrome coronavirus (SARS-CoV) outbreak in 2002–2004, steroid therapy was commonly administered along with other medications. Initially, the WHO did not support their use without the results of clinical trials being assessed and only recommended their strict use in especial clinical circumstances. However, with the progression of the pandemic, robust evidence for the associations of corticosteroids with the clinical outcomes in COVID-19 is becoming available since steroids are being currently administered in many parts of the world (Mattos-Silva et al., 2020).
A recent retrospective study indicated that methylprednisolone was associated with a decreased risk of death (HR 0.38; 95% CI 0.20–0.72) in patients with severe COVID-19, who developed ARDS (Wu et al., 2020). Another retrospective study revealed that COVID-19-hospitalized patients taking steroids were associated with a significantly lower mortality rate than those who did not take steroids (13.9 vs. 23.9%; HR 0.51, 95% CI 0.27–0.96, p = 0.044) (Fernández-Cruz et al., 2020). Very recently, an open-label randomized controlled trial (RCT) showed that COVID-19-hospitalized patients taking dexamethasone were associated with a significantly lower rates of 28-day mortality than the patients taking standard of care (RR 0.83, 95% CI 0.74–0.92, p = 0.0007). This study further revealed that dexamethasone reduced mortality significantly in ventilated COVID-19 patients (RR 0.65, 95% CI 0.48–0.88, p = 0.0003) as well as in patients who have taken supplemental oxygen (RR 0.80, 95% CI 0.67–0.96, p = 0.0021) (Horby et al., 2021).
Systemic steroids especially dexamethasone in specific COVID-19 patients, for example, critically ill or require supplemental oxygen, may be considered based on the current available evidence. The clinical benefits of dexamethasone use may be apparent in COVID-19 patients if they were treated for greater than 7 days after the onset of COVID-19-related symptoms (Gavriatopoulou et al., 2021).
6.6 Bronchodilators/Vasodilators
Bronchodilators may be administered whenever indicated in COVID-19 patients. Severe COVID-19 patients with hypoxemia may be particularly benefited from the pulmonary vasodilators. Although the lack of rigorous evidence did not favor the use of pulmonary vasodilators, for example, nitric oxide in COVID-19 patients, a recent, open-label, parallel-group, phase 2, RCT indicated that early inhalation of budesonide reduced the risk of urgent medical care support and also reduced the time to recover from early COVID-19 diagnosis (Gavriatopoulou et al., 2021; Ramakrishnan et al., 2021).
6.7 Non-Steroidal Anti-Inflammatory Drugs
Since fever and pain are common in SARS-CoV-2 infection, paracetamol should be generally considered as a first-line antipyretic and analgesic agent if not contraindicated due to other clinical conditions. However, ibuprofen may be reserved for patients who are unable to tolerate paracetamol until further studies clarify the adverse and beneficial effects of non-steroidal anti-inflammatory drugs (NSAIDs) in patients with COVID-19 (Robb et al., 2020; Gavriatopoulou et al., 2021).
7 Pharmacogenomics and Precision Medicine for COVID-19
Pharmacogenomic considerations of currently used COVID-19 therapeutics may help clinicians to optimize the efficacy or safety of these drugs, and may accelerate the development of precision COVID-19 medicine. To mitigate devastating catastrophe associated with COVID-19, many drugs without establishing robust evidence of efficacy or magnitude of toxicities have been used in these patients either as an off-label/compassionate use or as a clinical trial under these unprecedented health situations as an urgent attempt. Although pharmacogenomic determinants are very important considerations in optimizing the efficacy or toxicity of many repurposed antiviral drugs or supportive treatments, they are the most neglected issues in COVID-19 therapeutics since there are almost no pharmacogenomics data for these drugs (Takahashi et al., 2020). It is very unlikely that pharmacogenomic associations of COVID-19 therapeutics with the efficacy or safety have not been considered yet in any clinical studies.
8 Pharmacogenomic Landscape of COVID-19 Therapies
8.1 Genetic Variants Affecting the Safety or Efficacy of COVID-19 Therapies
8.1.1 Drug-Metabolizing Enzymes
Many drugs either repurposed antivirals or supporting medications that are used in the treatment of COVID-19 are metabolized by a number of drug-metabolizing enzymes called cytochrome P450 (CYP) enzymes. Genetic variants of the CYP genes encoding these important CYP enzymes may regulate their expression and may also contribute to drug response variability by altering the PK properties of the respective drugs. Therefore, for achieving optimal efficacy or safety of COVID-19 therapeutics, CYP genes of interest should be considered in future clinical studies to investigate such genetic associations. Here, we will summarize all CYP genes with potential interest of single-nucleotide polymorphisms (SNPs) as well as other genes affecting the PK profile of COVID-19 therapeutics with potential considerations of pharmacogenomics (PGx) of these drugs in other clinical conditions as evidenced from the literature (Table 2).
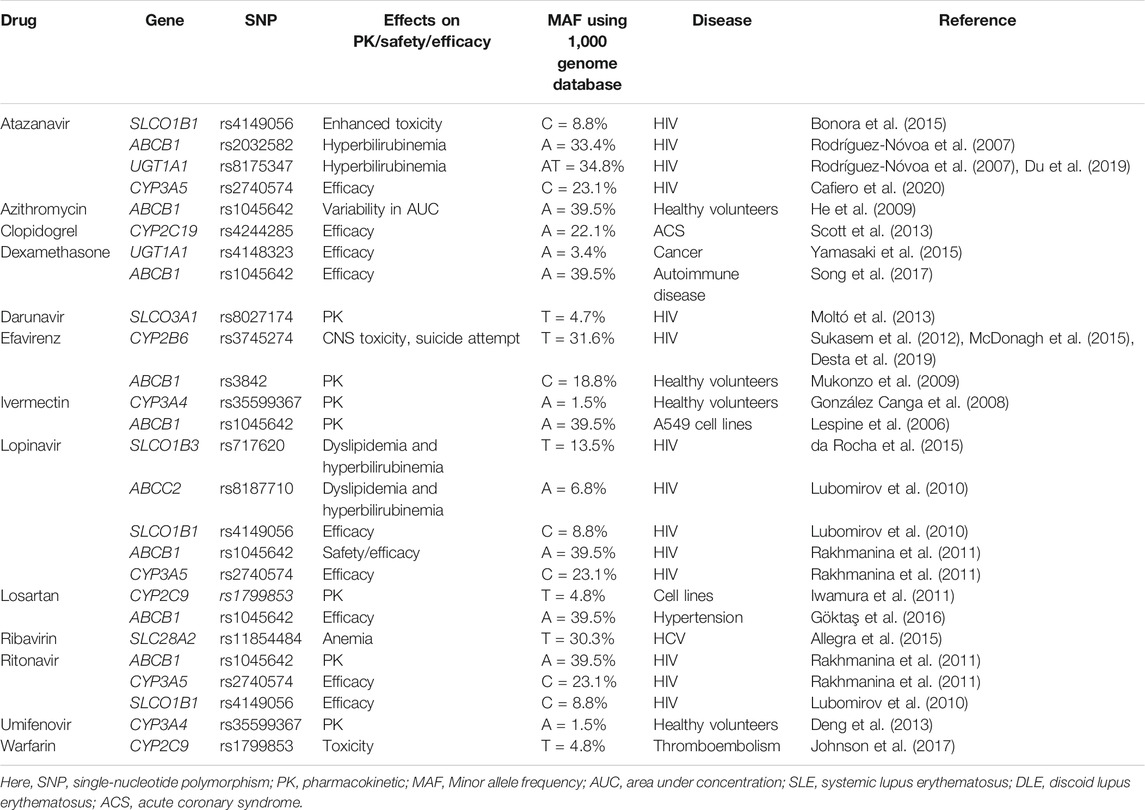
TABLE 2. Evidence of pharmacogenetic associations of COVID-19 therapies in other disease conditions.
It is important to recognize that CYP genetic variants are highly polymorphic and only few of these genetic variants are associated with the safety or efficacy of the respective drugs. The most prevalent and studied genetic variants of the CYP3A4/5, CYP2B6, CYP2C8, CYP2C9, CYP2C19, and CYP2D6 pharmacogenes have wide inter-ethnic variability. For example, CYP2D6*4 is highly prevalent in American population, whereas CYP2C19*2, *3 is highly prevalent in South Asians (Sukasem et al., 2013; Medhasi et al., 2016; Zhou et al., 2017; Biswas, 2021a; Sukprasong et al., 2021).
8.1.2 Transporters
In addition to CYP genes, some other efflux or uptake transporter proteins encoded by the transporter genes may also modify the PK properties of COVID-19 therapeutics and may be associated with the efficacy or safety variability. Like CYP genetic variants, transporter genes such as ABCB1, SLCOIB1, and ABCC2 also have interindividual variabilities and may affect the safety or efficacy of drugs accordingly (Sensorn et al., 2013; Medhasi et al., 2016; Atasilp et al., 2020; Biswas, 2021b). The list of transporter genes with relevant COVID-19 drugs with potential considerations of PGx in other clinical conditions as evidenced from the literature is shown in Table 2.
8.2 Other Genes Affecting the Severity of SARS-CoV-2 Infection
8.2.1 HLA
The human leukocyte antigen (HLA) encoded by the HLA gene is located on chromosome 6p21 which contains crucial regulators of immune response. The classical genes HLA-A, HLA-B, and HLA-C are in Class I and the classical genes HLA-DP, HLA-DQ, and HLA-DR are in Class II (Wang et al., 2015). HLA Class I has a role to present pathogen peptides to CD8+ T cell, becoming cytotoxic T cell which can directly destroy infected cell by inducing apoptosis (cellular immunity), whereas HLA class II has a role to present pathogen peptides to CD4+ T cell, activating B cell to become plasma cell and produce antibodies (humoral immunity) (Figure 1). However, in the case of viral infection such as COVID-19, cellular immunity is more important than humoral immunity to clear out the viruses which are staying inside the host cells (Le Bert et al., 2020).
Some variation of HLA alleles also has an association with some certain disease. The recently introduced genome-wide association study (GWAS) has suggested that several genes converging in common pathways contribute to the genetic susceptibility in several disorders, such as ankylosing spondylitis, psoriasis, chronic beryllium disease, rheumatoid arthritis, celiac disease, type 1 diabetes, and multiple sclerosis (Fiorillo et al., 2017). For example, 90–95% of patients with ankylosing spondylitis have HLA-B27 (Zhu et al., 2019). HLA alleles are not only associated with a set of autoimmune disease; the study in Thai children with autism showed that HLA-B*13:02, HLA-B*38:02, HLA-B*44:03, and HLA-B*56:01 alleles were significantly increased in autistic subjects compared with normal subjects (Puangpetch et al., 2015).
In addition to an association with some diseases, HLA alleles are also associated with an increased risk of certain drug allergy (Sukasem et al., 2014b; 2018b). The study in Thai population shows that HLA-B*15:02 is strongly associated with aromatic antiepileptic drug (AED)-induced Stevens-Johnson syndrome (SJS)/toxic epidermal necrolysis (TEN) (Sukasem et al., 2021b); HLA-B*15:02 and HLA-C*08:01 alleles are significantly associated with co-trimoxazole (CTX)-induced SJS/TEN, whereas the HLA-B*13:01 allele was significantly associated with CTX-induced drug reaction with eosinophilia and systemic symptoms (DRESS) (Sukasem et al., 2020a); HLA-B*46:01, HLA-B*56:02/04, and HLA-B*40:01 alleles contribute to the risk of phenytoin-induced cutaneous adverse drug reactions (PHT-induced cADRs) (Sukasem et al., 2020b); carbamazepine-induced SJS/TEN shows an association with HLA-B∗15:21 allele (Jaruthamsophon et al., 2017; Sukasem et al., 2018a); HLA-A∗02:07 and HLA-B∗15:02 are associated with lamotrigine (LTG)-induced cutaneous adverse drug reactions (cADRs) (Koomdee et al., 2017); HLA-B*13:01 is associated with dapsone-induced severe cutaneous adverse reactions including SJS/TEN and DRESS (Tempark et al., 2017); and HLA-B*58:01 is associated with allopurinol-induced SJS/TEN, and screening for HLA-B*58:01 alleles in patients who will be treated with allopurinol would be clinically helpful in preventing the risk of developing cADRs (Sukasem et al., 2016b). The future of pharmacogenomics-guided therapy in clinical settings across Thailand appears promising because of the availability of evidence of clinical validity of the pharmacogenomics testing (Sukasem et al., 2021a). The effectiveness of HLA screening on a wider scale in clinical practice requires significant resources, including state-of-the-art laboratory; multidisciplinary team approach, and healthcare provider education and engagement; clinical decision support alert system via electronic medical record (EMR); laboratory standards and quality assurance; evidence of cost-effectiveness; and cost of pharmacogenomic tests and reimbursement (Jantararoungtong et al., 2021a).
The severity of COVID-19 ranges from being asymptomatic to developing into a fatal acute respiratory syndrome and varies between populations. This can be linked to the variations in the HLA. The set of HLA alleles might determine the immune responses to viruses according to the selected peptides that can bind to the peptide-binding groove. A recent study from Italy showed an increase in the frequency of HLA-B*27:07, HLA-DRB1*15:01, and HLA-DQB1*06:02 among severe COVID-19 patients in a cohort of 99 Italians (Novelli et al., 2020). However, another study from Sardinia in Italy showed a negative influence on the disease course in the presence of the HLA-DRB1*08:01 allele (Littera et al., 2020). A study from Spain showed that the HLA-A*11, HLA-C*01, and HLA-DQB1*04 were associated with higher mortality in a cohort of 72 patients (Lorente et al., 2021). A study from 95 South Asian patients showed an increase in the frequency of HLA-B*51 and HLA-DRB1*13 in the fatal group compared to the mild infection group, while increase in the frequency of HLA-B*35 among the mildly infected group (Naemi et al., 2021). A study in 147 individuals of European descent experiencing variable clinical outcomes following COVID-19 infection showed that a significant difference in the allele frequency of HLA-DRB1*04:01 was found in the severe patient compared to the asymptomatic group, whereas a significantly lower frequency of the HLA-DQA1*01:01, HLA-DQB1*05:01, and HLA-DRB1*01:01 alleles was found in the asymptomatic group compared to the background population (Langton et al., 2021). A discrepancy between the studies can be attributed to many factors, including sample size and ethnic variations.
In addition to the prediction of certain diseases and certain adverse drug effects, an association between polymorphism in the HLA system and COVID-19 severity might have an impact on the implementation of a screening program to identify individuals at risk for COVID-19. In Thailand, the top ranked HLA alleles include HLA-A*11:01 (26.06%), HLA-B*46:01 (14.04%), HLA-C*01:02 (17.13%), HLA-DRB1*12:02 (15.32%), HLA-DQA1*01:01 (24.89%), and HLA-DQB1*05:02 (21.28%), and when focusing on HLA-B, the most frequent alleles were HLA-B*46:01 (11.51%), HLA-B*58:01 (8.62%), HLA-B*40:01 (8.22%), HLA-B*15:02 (8.16%), HLA-B*13:01 (6.95%), and HLA-B*44:03 (4.21%) (Puangpetch et al., 2014; Satapornpong et al., 2020). According to the study from Spain, Thai people should be alert because HLA-A*11and HLA-C*01 alleles are associated with high mortality. Moreover, Thai population has less frequency of a good prognostic marker such as HLA-B*35 studied from South Asia. However, clinical analysis of the association between HLA allele frequency and COVID-19 severity in Thailand is needed to validate the HLA alleles as the appropriate prognostic markers used in Thai clinical practice.
8.2.2 ACE2
Angiotensin-converting enzyme-2 (ACE2) is a protein consisting of 805 amino acids encoded by the ACE2 gene and is expressed in many parts of human cells including oral mucosa and nasopharynx. It is well recognized that ACE2 serves as an entry binding receptor of SARS-CoV-2 through interactions with specific amino acids of this enzyme (Sanders et al., 2020). A recent in silico model of possible ACE2 genetic variants with its interaction with the SARS-CoV-2 spike (S) protein has been analyzed, and it revealed that both rs73635825 (S19P) and rs143936283 (E329G) were shown to interfere with the ACE2 interaction with the S protein of SARS-CoV-2 (Ambrocio-Ortiz et al., 2021). After analyzing SNPs of ACE2 with susceptibility to SARS-CoV-1 or MERS, recent studies predicted that certain SNPs of ACE2 should consider COVID-19 patients for assessing the correlation with severity. It was also predicted that COVID-19 severity would vary around the world since the prevalence of the ACE2 genetic variants was significantly different in various ethnic groups (Benetti et al., 2020; Gemmati and Tisato, 2020; Ambrocio-Ortiz et al., 2021; Bakhshandeh et al., 2021; Biswas, 2021c; Choudhary et al., 2021).
8.2.3 TMPRSS2
Transmembrane protease, serine 2 (TMPRSS2) is an enzyme of serine protease family encoded by the TMPRSS2 gene. During membrane fusion, SARS-CoV-2 “S” protein is activated by the TMPRSS2; therefore, it is postulated that TMPRSS2 variants might have been correlated to COVID-19 severity. Genetic variants of TMPRSS2 augmenting TMPRSS2 activity might play an important role in the progression of COVID-19 severity and may be considered as a genetic risk factor (Hou et al., 2020; Choudhary et al., 2021).
9 Pharmacogenetics Considerations of COVID-19 Therapeutics: Implications for Efficacy and Safety
9.1 Antiparasitics
Due to proven ineffectiveness and exclusion from COVID-19 treatment protocols, we have excluded hydroxychloroquine and chloroquine from further analysis in this review.
9.1.1 Ivermectin
Ivermectin underwent extensive metabolism via CYP enzymes, predominantly by the CYP3A4 isoform, converting ivermectin into at least 10 metabolites, most of which are hydroxylated and demethylated products (Zeng et al., 1998; González Canga et al., 2008). Ivermectin is also a substrate of P-gp encoded by the ABCB1, and genetic polymorphisms of ABCB1 have linked to severe neurologic ADRs (Lespine et al., 2006; Baudou et al., 2020). Also, ivermectin is transported by the OATP1A2 and OATP2B1 encoded by the SLCO1A2 and SLCO2B1, respectively, although no pharmacogenetic study was identified for this association to date in the literature (Fricke-Galindo and Falfán-Valencia, 2021; Telbisz et al., 2021).
9.2 Antiviral Drugs
9.2.1 Remdesivir
Second, although remdesivir (RDV) is a promising investigational drug proving its activity in cell culture and animal models against SARS-CoV, Middle East respiratory syndrome corona virus (MERS-CoV), and SARS-CoV-2, it is currently not approved for any indication (FDA, 2020). In vitro studies suggest that RDV is a substrate for multiple drug metabolizing enzymes, for example, CYP2C8, CYP2D6, and CYP3A4, and also a substrate of OATP1B1 and P-glycoprotein (P-gp) transporters (Takahashi et al., 2020; Deb et al., 2021). Although the pharmacogenomic study of RDV has not been undertaken yet, it is predicted that known variants of these metabolic/transporter genes could affect the safety or efficacy of remdesivir and should assess COVID-19 patients. It is important to note that all of these genes were considered very important pharmacogenes (VIPs) by PharmGKB (Takahashi et al., 2020).
In addition to CYP/transporters’ involvement in the PK, RDV prodrug undergoes an intra-cellular sequential metabolism predominantly mediated by hydrolase activity to an active C-adenosine nucleoside triphosphate analog (Eastman et al., 2020; European Medicines Agency, 2020). Upon diffusion of RDV into the cell, the conversion of RDV into the nucleoside monophosphate form is presumably initiated by carboxylesterase (CES)-mediated hydrolysis of the amino acid ester that liberates a carboxylate and then converted to cyclic anhydride (Eastman et al., 2020; McCreary and Pogue, 2020; Yang, 2020). Cyclic anhydride is very unstable and hydrolyzed by water to the alanine metabolite GS-704277 which is further hydrolyzed by phosphoramidase to the nucleoside monophosphate. Nucleoside monophosphate is further phosphorylated in the presence of nucleoside phosphate kinase enzyme, yielding the active nucleoside triphosphate analog which may act as an analog of adenosine triphosphate (ATP) and competes with the natural ATP substrate to selectively inhibit RNA-dependent RNA polymerase (RdRp). Since the conversion of RDV to pharmacologically active nucleoside triphosphate analog is initiated extensively by the intracellular CES, we predict that genetic variability of CES gene-regulating expression may affect the safety or efficacy of RDV, and it is therefore hypothesized that “COVID-19 patients inheriting CES genetic polymorphisms might potentially modify efficacy or safety of RDV warranting clinical studies to be assessed for the achievement of precision medicine of RDV.”
9.2.2 Ribavirin
A genetic association study of ribavirin in some other viral infections except SARS-CoV-2 revealed that some genetic polymorphisms may result in up to 30% variability of ribavirin trough concentrations, affecting its safety and efficacy. It was found that patients who carried the homozygous variant of the SLC29A1, encoding influx transporter, were associated with significantly higher trough concentrations than wild-type variants (2,070 ng/ml vs. 1,837 ng/ml; p = 0.02). By contrast, patients who carried the homozygous variant of the SLC28A2 were associated with significantly lower trough concentrations than wild types (homozygous 1,595 ng/ml vs. heterozygous 1,933 ng/ml vs. wild-type 2,229 ng/ml; p = 0.04). This is also consistent with SLC28A3 variant (homozygous variant 2,294 ng/ml vs. heterozygous variant 1,813 ng/ml; p = 0.01) (Allegra et al., 2015). It is also well-established that hemolytic anemia, the most common dose-limiting toxic effect of ribavirin, is protected by various genetic variants of ITPA, encoding inosine triphosphatase (D’Avolio et al., 2016). In a meta-analysis of 20 studies, hemoglobin was significantly reduced in patients with wild-type alleles of ITPA compared to the patients having single-nucleotide polymorphisms (SNPs) as reported in a meta-analysis consisting of 20 studies (OR: 12.8, 95% CI: 7.4–22.1 for rs1127354 CC; OR: 3.4, 95% CI: 2.1–5.6 for rs7270101 AA; OR: 4.4, 95% CI: 2.8–7.0 for rs6051702 AA) (Pineda-Tenor et al., 2015). It was established that reduced activity of ITPA due to genetic variants governs to the deposition of inosine triphosphate and safeguard against ribavirin-induced very toxic effects, that is, hemolysis. Hemolytic anemia was also found for a short-term use of ribavirin in respiratory viral infection (Burrows et al., 2015). By contrast, a genome-wide association study (GWAS) of 303 patients with hepatitis C viral infection who were administered ribavirin with other therapy showed that the risk of thrombocytopenia was significantly higher in patients with rs6139030 SNP of ITPA (OR: 3.9, 95% CI: 2.8–5.5, p = 1.33 × 10–15) (Tanaka et al., 2011). Approximately 25% of the Thai patients carried ITPA genetic polymorphisms (Jantararoungtong et al., 2021b), which revealed that considerable proportion of Thai population taking ribavirin would be affected by the ITPA genetic variabilitites.
While the association of influx transporter genetic variants or ITPA with the safety or efficacy of ribavirin was not investigated in COVID-19 patients, such genetic association assessments are warranted in future clinical studies.
9.2.3 Favipiravir
Favipiravir (FPV) is one of the most potential antiviral drugs currently under considerations in several clinical trials to evaluate its efficacy and safety in patients with COVID-19 (Du and Chen, 2020). The National Medical Products Administration (NMDA) of China approved emergency use of FPV for a clinical trial in adult patients with COVID-19. Being a prodrug, FPV is ribosylated and phosphorylated to form active metabolite called FPV ibofuranosyl-5′-triphosphate, which then competes with purine nucleosides and interferes the viral replication by potentially inhibiting the RdRp of RNA viruses, for example, SARS-CoV-2 (Du and Chen, 2020). FPV is metabolized mainly by aldehyde oxidase and to a less extent by the xanthine oxidase (Takahashi et al., 2020). Although there are no published studies that have specifically assessed the pharmacogenomic influence of FPV, genetic variants of aldehyde oxidase were associated with pharmacodynamic outcomes in other drugs which are substrates of aldehyde oxidase such as azathioprine or allopurinol, and suggesting that PGx of FPV should also be taken into considerations in COVID-19 patients (Takahashi et al., 2020).
9.2.4 Oseltamivir
Oseltamivir is a prodrug which is converted to the active metabolite via carboxylesterase 1 (CES1) encoded by the CES1 (Shi et al., 2006). The SNP rs71647871 of CES1 has been found to be associated with variation in plasma concentration–time curve of oseltamivir (Tarkiainen et al., 2012) The PK of oseltamivir may also be affected by the ABCB1, CES1, NEU2, and SLC15A1 genetic variants. The SNP rs1045642 of ABCB1 was associated with neurologic ADRs developed by oseltamivir (Bermúdez de León et al., 2020; Fricke-Galindo and Falfán-Valencia, 2021).
9.2.5 Nevirapine
Certain genetic polymorphisms of HLA and CYP2B6 may be associated with increased risk of SJS/TEN when treated with nevirapine as reported elsewhere (Martin et al., 2005; Badary, 2021). Also, selective ABCB1 genetic variants may also be responsible for developing hepatotoxicity as evidenced elsewhere when treated with nevirapine (Vitezica et al., 2008; Badary, 2021).
9.3 Antiretroviral Agents
9.3.1 Lopinavir/Ritonavir
After pharmacogenomic analysis of 1,380 variants in 638 HIV-infected Caucasian patients taking LPV/RTV, four significant variants were identified. LPV/RTV clearance was higher in patients who carried SLCO1B1*4/*4 homozygous variants and was lower in patients who carried two or more variant alleles of the SLCO1B1*5, ABCC2, or CYP3A tag than in the patients of the reference group (Lubomirov et al., 2010). GWAS after analyzing 290 variants with the toxicity of LPV/RTV among 104 Caucasian patients with HIV revealed that dyslipidemia and hyperbilirubinemia were significantly associated with some genetic variants of the CETP, MCP-1, ABCC2, LEP, and SLCO1B3 genes. Also, a genetic variant of the IL-6 gene was significantly associated with resulting in diarrhea (all p < 0.01) (Aspiroz et al., 2014; Takahashi et al., 2020).
In addition to these, LPV/RTV being a substrate of P-gp was encoded by the ABCB1 gene. The efficacy and safety of these drug combinations may also be affected by the genetic polymorphisms of ABCB1 encoding P-gp expression. Over 30% of the Thai patients inherited C3435T ABCB1 genetic polymorphisms (Sensorn et al., 2013, 2016), suggesting that considerable proportion of Thai population be affected by the C3435T ABCB1 genetic variant if taking LPV/RTV for combating COVID-19. A recent review hypothesized that the safety or efficacy of LPV/RTV may be affected by the C3435T SNP of ABCB1, and the risk phenotypes due to carrying this SNP were prevalently highest in Europe (76.8%), followed by America (67%), Asia (63.5%), and Africa (41.4%) (Biswas, 2021b).
9.3.2 Darunavir/Cobicistat
Darunavir being a substrate of CYP3A4 was used simultaneously with cobicistat, a CYP3A4 inhibitor in a clinical trial for COVID-19 for increasing the exposure of darunavir (Takahashi et al., 2020). Genetic variants of CYP3A4 regulating the function or expression of CYP3A4 may affect the safety or efficacy of darunavir/cobicistat in COVID-19 patients and should be considered in future studies (Takahashi et al., 2020). Although there is no direct evidence that darunavir is a substrate of SLCO3A1, a 12% significantly lower Darunavir clearance was reduced in patients with SLCO3A1 variant, suggesting that this might be a substrate of darunavir and should assess COVID-19 patients (p < 0.05) (Moltó et al., 2013).
9.3.3 Atazanavir
Atazanavir (ATV) is metabolized by UGT1A and is also an inhibitor of CYP3A. Several genetic polymorphisms of UGT1A1, for example, UGT1A1*6, *28, *36, *37, and *80, may affect the PK of ATV and may produce toxicity as outlined in the CPIC dosing guidelines. The CPIC pharmacogenomic-based dosing guidelines have recommended to counseling the patients carrying these variants because of possibility for developing severe hyperbilirubinemia (Gammal et al., 2016). A rapid, reliable, cost-effective, and simple assay to detect UGT1A1 genetic polymorphisms in has already been developed for adoption in routine clinical practice (Sukasem et al., 2016a). The metabolism of ATV is also partially governed by the P-gp encoded by the ABCB1, and patients carrying C3435T ABCB1 SNP may be at risk of hyperbilirubinemia and severe jaundice as well. Numerous studies showed that certain genetic polymorphisms of APOA5, APOC3, ABCA1, and APOE genes were associated with increased risk of dyslipidemia in patients taking atazanavir (Zanone Poma et al., 2008; Suwalak et al., 2015; Badary, 2021).
9.3.4 Efavirenz
Since efavirenz is predominantly detoxified by the CYP2B6, therefore, patients may be at increased risk for toxicity such as depression and suicidal tendencies with some CYP2B6 genetic variants, reducing the function of CYP2B6 (McDonagh et al., 2015; Desta et al., 2019). Pharmacogenomics for this drug have been extensively studied including in Thai HIV patients, and the CPIC guideline has already been developed for guiding patients with CYP2B6 genetic variants (Sukasem et al., 2012; Sukasem et al., 2014a; Manosuthi et al., 2014; Desta et al., 2019). The SNP rs4803419 of CYP2B6 was independently associated with increased plasma efavirenz concentration as found in a GWAS (Holzinger et al., 2012). Serious toxic effects of efavirenz, for example, depression and suicidal tendencies, can be optimized by adjusting the dose based on CYP2B6 genotyping results of patients (Desta et al., 2019).
9.4 Interferon β-1b
An interferon (INF) regulatory factor (IRF6) encoded by the IRF6 was significantly associated with increased risk of liver injury as identified in a case–control study of IFN-β1b-treated multiple sclerosis patients (OR: 8.3, 95% CI: 3.6–19.2; p = 2.3 × 10–8). The results were subsequently confirmed in an independent cohort study of patients with multiple sclerosis in which liver injury was proved with significantly increased aspartate aminotransferase and alkaline phosphatase concentrations for those who carried IRF6 genetic variants (Kowalec et al., 2018; Takahashi et al., 2020).
9.5 IL-6 and IL-1 Antagonists
Genetic polymorphisms of the FCGR3A, IL6R, CD69, and GALNT18 genes may affect the efficacy of tocilizumab in RA as reported elsewhere (Maldonado-Montoro et al., 2016; Maldonado-Montoro et al., 2018; Jiménez Morales et al., 2019). It was reported that the FCGR3A rs396991TT genotype had a higher response rate at 12 months therapy of tocilizumab in 87 patients with RA (OR: 5.1; 95% CI: 1.2–21.3; p = 0.03). Specific Fc fragment of the IgG receptor binding to tocilizumab may be altered by this selective genetic variant and may change systemic clearance of this drug (Jiménez Morales et al., 2019). Polymorphisms of other genes, for example, IL6R, CD69, and GALNT18, have limited direct effects on the safety or efficacy of tocilizumab (Maldonado-Montoro et al., 2016, 2018). Also, relevant pharmacogenomic data affecting either safety or efficacy of other IL-6 or IL-1 antagonists, that is, sarilumab, siltuximab, and anakinra, were not found in the literature (Takahashi et al., 2020). Although considerations of all of these pharmacogene are highly speculative, at least FCGR3A rs396991TT SNP should be replicated in COVID-19 patients.
9.6 Inhibitors of the Renin Angiotensin Aldosterone System
Renin angiotensin aldosterone system (RAAS) inhibitors are affected by the CYP2C9 and ABCB1 genetic variabilities. For example, patients carrying reduced function alleles of CYP2C9, that is, *2, *3, may develop toxicity if taking losartan and dose adjustment based on genotyping of CYP2C9 could be beneficial to reducing toxicity (Iwamura et al., 2011; Gemmati and Tisato, 2020; Sriram and Insel, 2020; Badary, 2021). Therapeutic response of losartan may also be affected by the C3435T SNP of ABCB1 since a recent study found a significantly increased absorption of losartan in the early phase in patients who carried this variant (Shin et al., 2020).
9.7 Janus Kinase Inhibitors
Ruxolitinib is metabolized via CYP3A4 and CYP2C9, while baricitinib is metabolized partially by CYP3A4 (Umehara et al., 2019; Takahashi et al., 2020; Veeravalli et al., 2020). Both CYP3A4 and CYP2C9 genes are tabulated as VIPs in the PharmGKB database, and certain genetic polymorphisms of these genes may affect the safety or efficacy of the, respective, drugs. Also, the PK of baricitinib may be affected by OAT3 transporter encoded by the SLC22A8 (Takahashi et al., 2020).
9.8 Antibiotics
The PK properties of azithromycin may have interindividual variability due to the variation P-gp expression encoded by the ABCB1 gene. A single dose of azithromycin had ∼2-fold lower peak concentrations for those who carried rs1045642 SNP of ABCB1 (TT vs. CC: 468.0 vs. 911.2 ng/ml, p = 0.013), as found in 20 healthy volunteers (He et al., 2009). It is important to note that genetic variants of ABCB1 causing increased concentration of azithromycin may be of particular concern when concomitantly used with HCQ/CQ since the additive effects on QT prolongation may exert fatal arrhythmias (Scherrmann, 2020; Takahashi et al., 2020).
9.9 Corticosteroids
Efficacy and toxicities of corticosteroids have been linked to many genetic variants as assessed in various disease conditions. Genes of receptor binding (e.g., CRHR1 and NR3C1), folding proteins (e.g., ST13, STIP1, and FKBP5), metabolic enzymes (e.g., CYP3A4, CYP3A5, CYP3A7, and GSTT1), and efflux transporters (e.g., MDR1 and ABCB1) may have various genetic polymorphisms accounting for modulating the safety or efficacy of corticosteroids (Song et al., 2017). Pharmacogenetic studies assessing either safety or effectiveness of corticosteroids in either ARDS or COVID-19 were not found in the literature, and it is suggested that the impacts of genetic variants of the genes of interest should focus in future studies in patients with COVID-19 (Takahashi et al., 2020; Vohra et al., 2021).
9.10 Antiplatelets
The effects of CYP2C19 genetic variants on widely used antiplatelets, for example, clopidogrel in either CAD or stroke patients has been well-established including in Thai patients. The findings of these studies suggest that due to the high risk of major adverse cardiovascular events (MACE) such as death, recurrent MI, stroke, and stent thrombosis for patients carrying CYP2C19 loss-of-function (LoF) alleles, alternative antiplatelets such as prasugrel or ticagrelor not affected by the CYP2C19 genetic variants should be prescribed in order to reduce the risk of MACE (Sukasem et al., 2013; Biswas et al., 2020a; Biswas et al., 2021b; Biswas et al., 2022; Biswas and Kali, 2021b; Jafrin et al., 2021). The CPIC dosing guidelines already provided clinical recommendations for clopidogrel in acute coronary syndrome (ACS) patients with CYP2C19*2, *3, *17 variants (Scott et al., 2013). In addition to CYP2C19 genetic variability, magnitude of P-gp expression regulated by the ABCB1 genetic variants especially C3435T SNP of ABCB1 may also increase the risk of MACE as established in a recent meta-analysis (Biswas et al., 2020b). The episode of stroke or CAD especially MI is considerably high in severe COVID-19 patients (Bikdeli et al., 2020), and it is generally assumed that antiplatelets, for example, clopidogrel is used in these patients as a supportive care; therefore, it is suggested that pharmacogenomic considerations of antiplatelets are highly desirable to optimize the safety or efficacy of these life-saving drugs in severe COVID-19 patients.
9.11 Anticoagulants
Anticoagulants, for example, warfarin, have wide interindividual response variability due to the presence of CYP2C9 and VKORC1 genetic variants as reviewed elsewhere (Jorgensen et al., 2012; Takeuchi et al., 2020). Both the FDA and CPIC have recommended to consider both the CYP2C9*2, *3 and VKORC1 (rs9934438) genetic variants for optimizing its safety, that is, bleeding or efficacy in order to achieve precision medicine of warfarin (Dean, 2012; Johnson et al., 2017). Other new oral anticoagulants such as debigatran, rivaroxaban, and apixaban are affected by the ABCB1 genetic variants and should be considered clinically for optimizing the safety and efficacy (Xie et al., 2018; Kanuri and Kreutz, 2019).
9.12 Non-Steroidal Anti-inflammatory Drugs
Non-steroidal anti-inflammatory drugs such as celecoxib, flurbiprofen, ibuprofen, and lornoxicam are predominantly metabolized by CYP2C9 and to a lesser extent by CYP1A2 and CYP3A4. Gastrointestinal (GI) bleeding, myocardial infarction, renal damage, etc. are the most common serious adverse effects of NSAIDs; however, many NSAIDs are considered safe and are frequently used as the over-the-counter medicine (Theken et al., 2020). A recent meta-analysis showed that individuals with CYP2C9 poor metabolizers were associated with significantly increased risk of NSAID-related gastrointestinal bleeding (OR: 1.90, p = 0.003) and indicated that CYP2C9*2 was a poor risk predictor, while CYP2C9*3 was a highly significant predictor of GI bleeding (Macías et al., 2020). The CPIC guidelines provided clinical recommendations based on the CYP2C9 genotype and suggested to consider CYP2C9*2 and CYP2C9*3 variants for patients taking celecoxib, flurbiprofen, ibuprofen, and lornoxicam for optimizing the safety (Theken et al., 2020). Summary of the pharmacogenomics associations of some of the COVID-19 therapeutics with the safety or efficacy is illustrated in Table 3.
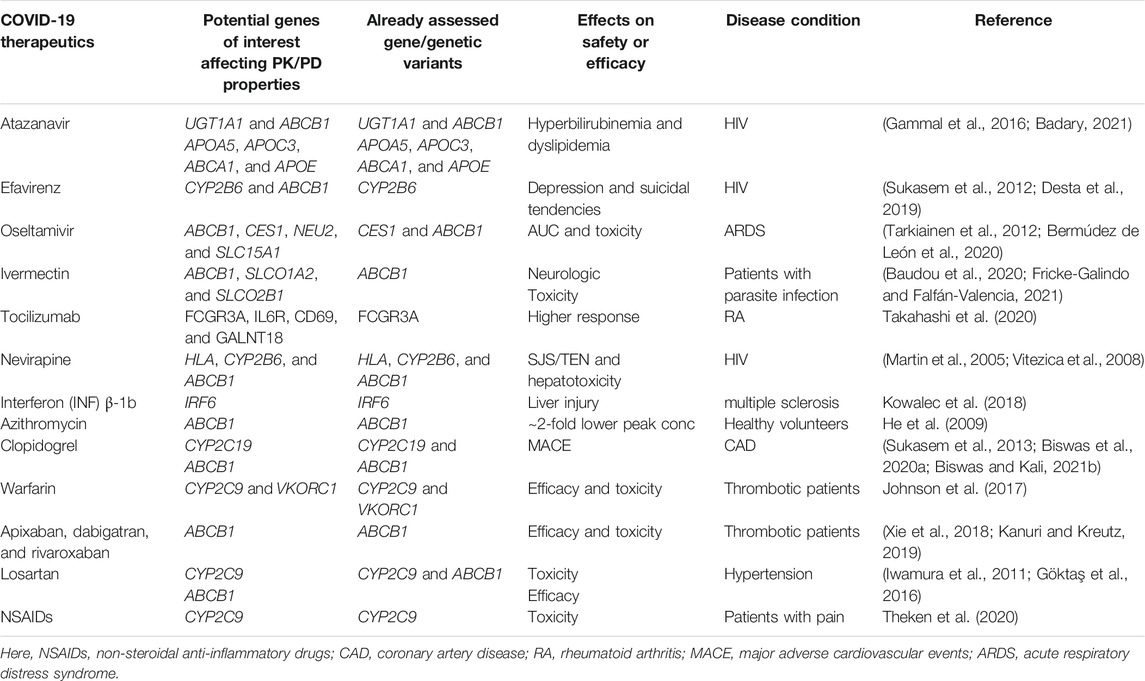
TABLE 3. Summary of the pharmacogenomic studies affecting the safety or efficacy of COVID-19 therapeutics in other clinical conditions.
10 In Silico Prediction of Drug Effects in Treatments for COVID-19
To combat COVID-19, computational aided-drug design and screening have been rapidly applied to identify FDA-approved drugs and newly potent compounds from available databases. Using in silico approaches, extensive research works have been carried out to acquire an understanding of mechanisms of action and SARS-CoV-2’s activities. However, there are still many foundations to be established for developing novel therapeutics agents for the treatment of COVID-19 (Amin and Jha, 2020). Herein, the current situation in the discovery of anti-SARS-CoV-2 agents at four important targets (Table 4) from in silico studies is described and summarized as follows.
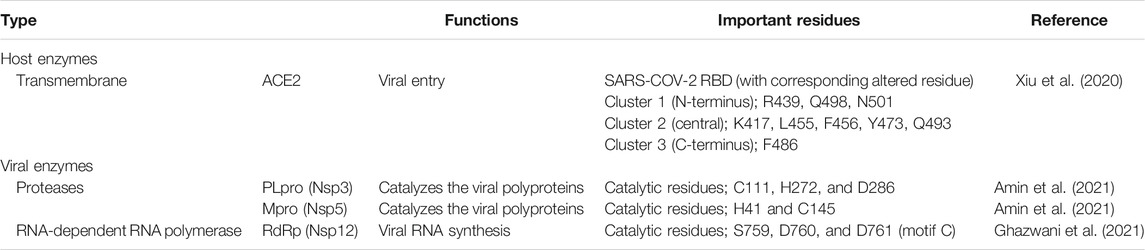
TABLE 4. Lists of important targets involved in SARS-CoV-2 life cycle mostly used in in silico study.
10.1 Spike Protein
SARS-CoV-2 enters into host cells by transmembrane spike (S) glycoprotein that forms homotrimers protruding from the viral surface. The S glycoprotein consists of two subunits responsible for either host cell receptor binding (S1 subunit including the receptor-binding domain, RBD) or the virus fusion (S2 subunit) (Yang et al., 2020). The ACE2 receptor on the host cell is required for viral entering; however, following entry processes vary depending on the cell type (Xiu et al., 2020). The interface can be divided into three parts by mainly polar and is close to the SARS-CoV-2 S/ACE2 complex (Li et al., 2005; Song et al., 2018). In Figure 5A, the extended loop of RBD contacts with ACE2 mainly at the arch-like helix α1 of the proteolytic domain via N-terminal, central, and C-terminal (clusters 1–3), and partially at the helix α2 and β loops 3–4 (Xiu et al., 2020). The protein–protein binding is likely found at both terminals: 1) formed hydrogen bonds at the α1 N terminus (cluster 1) between the RBD residues Q498, T500, and N501, and the ACE2 residues Y41, Q42, K353, and R357; and 2) van der Waals interactions of Q474 (RBD)--Q24 (ACE2) and F486 (RBD)--M82 (ACE2) at another end (cluster 3). However, only the residue Y453 from the middle cluster 2 contacts with the ACE2 proteolytic domain at residue H34. The SSAA09E2 from the Maybridge HitFinder small-molecule library can inhibit the S-RBD/ACE2 binding (Adedeji et al., 2013), while the chloroquine (Vincent et al., 2005; Wang et al., 2020a) and hydroxychloroquine (Rainsford et al., 2015) used to treat several human diseases including COVID-19 were found to interfere the ACE2.
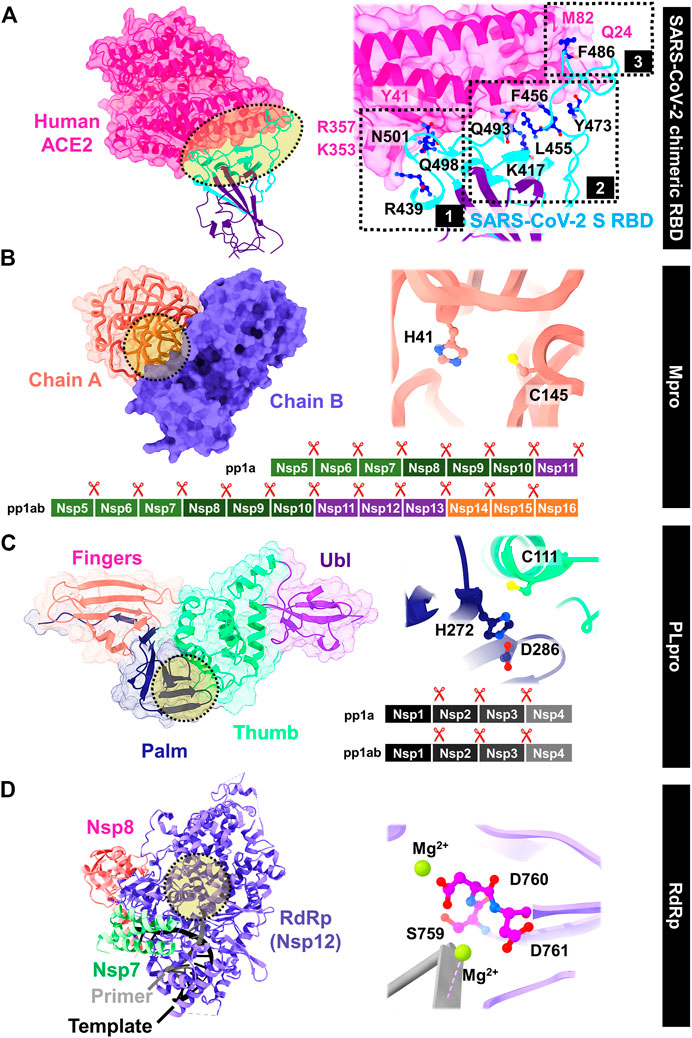
FIGURE 5. Viral targets for drug development against SARS-CoV-2: (A) S RBD/ACE2 binding, (B) Mpro, (C) PLpro, and (D) RdRp, whereas the important residues are also labeled.
From molecular docking study on ∼4,000 known drugs from the DrugCentral database (Br et al., 2020) and ∼7,000 antiviral agents from the Asinex database (Farouk et al., 2021) on the S/ACE2 interface followed by molecular dynamic (MD) simulation of screened compounds, the glycyrrhizic acid and the compound 6,612 with the highest binding affinity from the two following databases, respectively, were suggested for further in vitro and/or in vivo tests. The molecular docking, and physicochemical, pharmacokinetic, and MD studies indicated the solanine, acetoside, and rutin from plant-based natural compounds as the S and Mpro dual inhibitors (Teli et al., 2020; Deetanya et al., 2021). Moreover, several natural herbal compounds such as luteolin, andrographolide, zhebeirine, 3-dehydroverticine, ophiopogonin D, glycyrrhizin, saikosaponin C, crocin-1, and militarine formed strong hydrogen bonds at RBD could prevent the viral binding to ACE2 receptor (Stalin et al., 2021). In addition, the peptide antibiotics (polymyxin B, colistin, and daptomycin), pressure regulators (terlipressin and lypressin), hormone peptides (alarelin and leuprorelin), and immunostimulants (thymopentin) able to hamper the RBD/ACE2 interaction were identified by an in silico study. Aurintricarboxylic acid and heparin sodium with binding inhibition of 80 and 63% interacted with RBD at clusters 1 and 2, respectively (David et al., 2021). Computational results could help to demonstrate how geraniin can block the viral entry to human cells by preferentially binding at SARS-CoV-2 S RBD in agreement with the biolayer interferometry-based analysis (Kim et al., 2021).
10.2 Proteases
After virion entry into host cells, two polyproteins (pp1a and pp1ab) are translated, which are then divided by two viral proteases: main protease (Mpro) and papain-like protease (PLpro) (Freitas et al., 2020; Goyal and Goyal, 2020). The Mpro, also known as 3C-like protease (3CLpro), has received great attention because of its important involvement in enzymatic activity and post-translational processing of replicase polyproteins. This enzyme has high structural and sequence similarity with SARS-CoV Mpro (Peele et al., 2020; Das et al., 2021b). It contains two catalytic dyad residues C145 and H41 in the active site (Figure 5B) (Zhang et al., 2020c), whereas the residues H41, M49, G143, S144, H163, H164, M165, E166, L167, D187, R188, Q189, T190, A191, and Q192 are involved in substrate binding. The hydrophobic side chains are mainly present at the S2 and S4 sites (Amin et al., 2021). Figure 5C shows the structure of papain-like protease (PLpro) containing the catalytic triad residues C111, H272, and D286 in the active site. The C111 residue engages in Michael addition to the warhead of inhibitors with a formation of a covalent thioether linkage, while the residues Y268, M208, P247, P248, T301, P248, Y264, N267, Q269, L162, C270, G271, and Y273 are involved in substrate binding (Amin et al., 2021).
10.2.1 Main Protease
The in silico explorations of potential inhibitors against SARS-CoV-2 Mpro are summarized in Table 5. The first crystal structure of the Mpro with covalent inhibitor N3 was reported in January 2020 (Jin et al., 2020), and the co-crystal data available from many other research groups have provided the basis for fast target-based lead drug development against SARS-CoV-2 Mpro. They were utilized to create a pharmacophore model and perform docking research to identify anti-SARS-CoV-2 inhibitors such as lopinavir, remdesivir, ritonavir, saquinavir, and raltegravir (Daoud et al., 2021). Ritonavir was well occupied in the Mpro active site and interacted with the oxyanion hole residues N142 and G143 (Nutho et al., 2020). Jin et al. (2020) screened >10,000 approved drugs, candidates in clinical trials, and pharmacologically active compounds using combined structure-based virtual and high-throughput screening. The two FDA-approved drugs (disulfiram and carmofur) and four clinical trial compounds (ebselen, tideglusib, shikonin, and PX-12) showed potent SARS-CoV-2 Mpro inhibition with the IC50 range of 0.67–21.4 μM. Using the active site conformations of SARS-CoV-2 Mpro through protease pharmacophore clustering, the resulting anti-HCV drugs boceprevir and telaprevir and the anti-HIV drug nelfinavir from a set of 2,122 drugs exhibited significant Mpro inhibition and antiviral efficacy in the micromolar range (Pathak et al., 2021). From the superDRUG2 database, binifibrate and bamifylline identified by e-pharmacophore modeling using the Mpro structure co-crystalized with imidazole–carboxamide inhibitor can bind tightly at the active site and form hydrogen bonds with G143 and E166 throughout MD simulation (Arun et al., 2021). For the mechanism of action for the drug candidates against SARS-CoV-2 Mpro currently studied in clinical trials, the dynamic behavior of PF-07321332 and PF-00835231 showed hydrogen bond formations with C145, E166, and Q189 residues, while additional hydrogen bonds with G143 and H164 were observed in PF-00835231 binding (Ahmad et al., 2021; Baig et al., 2021).
Some natural products with promising pharmacodynamic and pharmacokinetic characteristics, for example, higenamine hydrochloride, phloretin, daidzin, and naringenin chalcone, were screened from the ZINC database using the receptor-based pharmacophore modeling and molecular docking (Saeed et al., 2021). The receptor-, ligand-, and machine learning-based screening methods elucidated the small-molecule inhibitors of Mpro with IC50 in the micromolar range: rottlerin (37 μM), amentoflavone (143 μM), baicalein (208 μM), and synthetic compounds (e.g., CID 46897844, 31 μM) (Glaab et al., 2021). The crucial residues frequently participating within these potent compounds are E166, T190, Q189, and Q192, while the catalytic residues H41 and C145 are important for amentoflavone and baicalein, respectively. The inhibitors such as N3 and myricetin which covalently bound to C145 could terminate the SARS-CoV-2 Mpro functions (Jin et al., 2020; Glaab et al., 2021). The MD study on Mpro in complex with the four reported peptidomimetic inhibitors N3, 11a, 13b, and 14b indicated that the ligand–protein complexation is mainly driven by vdW and hydrogen bond interactions (Somboon et al., 2021). The polar moieties (e.g., benzamide) and the bulky N-terminal protecting groups (e.g., thiazole) should be introduced to P1’ and P4 sites of 13b structure to increase hydrogen bonds and hydrophobic interactions, respectively.
10.2.2 Papain-Like Protease
The two irreversible inhibitors VIR250 and VIR251 with a significant degree of SARS-CoV-2 PLpro selectivity over other proteases were discovered (Rut et al., 2020), and their crystal structures were widely used for virtual screening. The PLpro inhibitors derived from in silico screening are illustrated in Table 6. The antidiabetic drug phenformin, anti-HIV drug ritonavir, and natural compound quercetin resulted from ∼1,700 clinical FDA-approved drugs showed favorable pharmacokinetics and strong binding interactions with SARS-CoV-2 PLpro (Kandeel et al., 2021). In addition to quercetin, PLpro has also been shown to bind with several compounds from the 26 Chinese herbal medicines such as cryptotanshinone and tanshinone IIa (Zhang et al., 2020b). Some anti-HCV drugs, namely, simeprevir, grazoprevir, and vaniprevir, with PLpro inhibition synergize with remdesivir to reduce SARs-CoV-2 virus replication in Vero and/or human cells (Bafna et al., 2021).
The naphthalene-based derivatives with previously reported SARS-CoV-1 PLpro inhibitory activity could be beneficial for SARS-CoV-2 treatment (IC50 values of 2.4 and 5 μM for GRL-0617 and compound 6, respectively) due to almost identical residues in the PLpro BL2 loop of the two viruses (Amin et al., 2021). The selective compounds from the ENAMINE REAL database using pharmacophore modeling which have IC50 values of 159–505 nM bind to the target protein in a similar manner to the non-covalent SARS-CoV-2 PLpro inhibitor, GRL-0617 (Stasiulewicz et al., 2021). The identified deubiquitinase inhibitors against PLpro, TCID and DUB-IN-3 with IC50 of 6.42 and 12.5 μM, formed hydrogen bonding with the PLpro residues Y264 and R166, respectively (Liu et al., 2021). The in silico molecular interaction-based method was used to elucidate the cyanobacterial metabolites against SARS-CoV-2 PLpro. The deoxycylindrospermopsin binding with the important residues T26, C44, F140, S144, C145, H163, and E166 was identified as the most promising inhibitory candidate (Naidoo et al., 2021). By molecular docking and MD study of 97 antiviral secondary metabolites from fungi, norquinadoline A was found to be the most effective inhibitor of SARS-CoV-2 PLpro with high gastrointestinal absorption, low blood–brain barrier penetrability, and high drug-likeness (Quimque et al., 2021).
10.4 RNA-Dependent RNA Polymerase
RNA-dependent RNA polymerase or RdRp (Nsp12) catalyzes viral RNA synthesis, and as a result, it plays a key role in viral replication and multiplication, alongside cofactors Nsp7 and Nsp8 proteins. Among seven key motifs in RdRp catalytic domain, motifs A–F are highly conserved across all viral RdRps, but motif G is a unique structural characteristic of primer-dependent RdRps in some positive-sense RNA viruses binding with the primer strand at the beginning of RNA synthesis. In Figure 5D, the catalytic residues S759, D760, and D761 with Mg2+ as a catalyst cofactor are located in motif C (Naydenova et al., 2021), while the other crucial residues are D618, C622, and D623 in the active site, S682, T687, A688, and N691 in motif B, and K545, R553, and R555 in motif F (Ghazwani et al., 2021).
Favipiravir is the first antiviral drug authorized for the treatment of SARS-CoV-2 by China’s National Medical Products Administration. Several drugs, that is, sofosbuvir, ribavirin, galidesivir, and remdesivir, are being tested in clinical trials against SARS-CoV-2 RdRp (Elfiky, 2020). In the reported crystal structures, the drugs favipiravir and remdesivir are accommodated in the ATP binding site of RdRp (Yin et al., 2020; Naydenova et al., 2021). In silico drug design and discovery of RdRp inhibitors are given in Table 7. In addition to remdesivir and ribavirin, the molecular docking study of 1,749 antiviral drugs suggested that paritaprevir, glecaprevir, and velpatasvir also showed interesting interactions with RdRp (Singh et al., 2021). The top 50 compounds retrieved from structure-based virtual screening of 15,220 compounds from DrugBank and TargetMol Bioactive compounds Library against SARS-CoV-2 RdRp were evaluated by bio-layer interferometry (BLI) binding followed by cell-based polymerase activity assays (Li et al., 2021). Corilagin showed the highest inhibition SARS-CoV-2 RdRp (KD of 0.54 220 μM) and inhibited viral replication in Vero cells (EC50 of 0.13 μM), by binding at RdRp’s palm domain and thus preventing the conformational changes necessary for nucleotide incorporation. Its binding pocket comprised the conserved residues S759, D760, and D761 in motif C, and the surrounding residues G616, D761, K798, W61, W800, D618, S814, E811, S549, C799, and A550. Relative to remdesivir, the 11 obtained compounds from the virtual screening and MD simulations derived from the ZINC database displayed significant interactions with all RdRp active site residues (Ghazwani et al., 2021). Based on pharmacophore modeling of the remdesivir/RdRp complex (two anionic acceptor, one donor, one acceptor, and one dual donor and acceptor features), the epigallocatechin gallate, kuromanin, procyanidin-b-2, and rutin were the top four hits among 5,836 compounds from the ChEMBL database (Kandwal and Fayne, 2020). Pharmacophore modeling, structure-based virtual screening, and MD simulations of RdRp bound with the known RdRp inhibitors were used to screen the potential agents from the six databases; PubChem-134297651, CHEMBL387201, CHEMBL1196124, PubChem-122704503, ZINC257357489, and ZINC5605331, which were highly interacting with RdRp at the ATP binding pocket (Grzybowski et al., 2002).
11 Pharmacogenomics for COVID-19 Vaccine
At least 13 different vaccines have been administered until now to combat the biggest infectious challenges of the 21st century. Although majority of these vaccines are well-tolerated, they may not be responsive similarly to everyone and may also account for some vaccine-related side/toxic effects. The application of existing pharmacogenetic/pharmacogenomic science to vaccines is termed as “vaccinomics” (Hoffman et al., 1998; Poland et al., 2007, 2021; Omersel and Karas Kuželički, 2020; Soiza et al., 2021). Since the pharmacogenomic association with the safety or efficacy of many clinical important medications, for example, antiepileptics, antiplatelets, cardiovascular drugs, antidepressants, and anticancers, have been well-established and many of these considerations are now implemented in routine clinical practice in some parts of the world, we are expecting that similar approaches in terms of COVID-19 vaccines will start to appear very soon.
Some genetic insights for vaccines applied in other infectious diseases have already been recognized, for example, specific genetic polymorphisms of the TLR3 gene were associated with significantly reduced immune responses to the measles vaccine as reviewed elsewhere (Poland et al., 2018). Vaccinomics provide a promising newly evolving research area through which the safety or efficacy of vaccines may be optimized. A wide range of genotype/phenotype association data are currently being integrated into this newly emerging research field for many live viral vaccines and expecting that similar attributes will begin for COVID-19 vaccines as well. The application of vaccinomics in COVID-19 may allow us to explain the interindividual immune responses’ variability and adverse events, and may also accelerate the development of personalized vaccine (Poland et al., 2021).
Immunogenicity data of COVID-19 vaccination come from the assessment of specific T-cell responses and specific antibody responses (Zhu et al., 2020). Both activation of cytotoxic T cell and antibody production need antigen presentation via HLA class I and II, respectively. We hypothesize that variation of the HLA genotype might affect the immunogenicity of COVID-19 vaccines. Further investigation in association between HLA polymorphism and COVID-19 vaccine immunogenicity is interesting and might help to predict individual COVID-19 vaccine effectiveness.
Within a year, several vaccines have been developed and millions of doses were delivered. The ChAdOx1 nCoV-19 vaccine (AstraZeneca) has recently been reported as an increasing risk of venous thrombosis and thrombocytopenia, called vaccine-induced immune thrombotic thrombocytopenia (VITT), 7–10 days after receiving the first dose (Schultz et al., 2021). Recently, some studies showed that pathogenic antibodies to platelet factor 4 (PF4), which have a major role to develop VITT, can occur after the administration of the ChAdOx1 nCoV-19 vaccine (Scully et al., 2021). This pathogenic PF4-dependent syndrome is unrelated to the use of heparin therapy, called heparin-induced thrombocytopenia (HIT). The higher PF4 level has an association with HLA-DRB1*03:01-DQB1*02:01 haplotype (Zhang et al., 2019). Genetic variants of HLA-DRB1 and HLA-DQB1 were found ∼25% in Thai population (Puangpetch et al., 2014; Satapornpong et al., 2020), indicating that considerable proprotion of Thai population might be at risk of developing VITT associated with these genetic polymorphisms. Clinical validation of the screening of HLA-DRB1*03:01 and HLA-DQB1*02:01 to predict the occurrence of VITT should be investigated, and it might help people to avoid this life-threatening condition. Even though platelet level is very low, VITT should not be treated by platelet transfusion. Ideally, such transfusion should be avoid because it would provide a substrate for further antibody-mediated platelet activation and coagulopathy. Therefore, rapid recognition of VITT is very important.
12 Precision Medicine for COVID-19 Treatment: Key Clinical Considerations
12.1 Drug–Drug Interactions
It is also alarming that many patients are developing serious complications, for example, life-threatening adverse drug reactions. Since the mortality of COVID-19 patients is significantly higher in older patients and also for those having multiple comorbidities (Biswas et al., 2021a), it is likely to expect DDIs and consequently ADRs generated from the risk of polypharmacy, as evidenced in recent observational studies (Ramírez et al., 2020; Falcão et al., 2021). For example, at least 62 life-threatening potential DDIs of LPV/RTV that should be considered in COVID-19 patients if taking these drugs have been identified (Biswas, 2020). A summary of very important clinically significant DDIs of COVID-19 therapies as evidenced or suggested elsewhere is shown in Table 8.
DDIs are likely important in case of assessing pharmacogenomic effects of any particular drug since sometimes the DDI may alter/exacerbate the clinical effects or change phenotypes (phenoconversion) associated with interactions with the, respective, genes (Klomp et al., 2020). A recent systematic review reported a phenoconversion from a higher metabolizer phenotype into a lower metabolizer phenotype by the concurrent use of CYP inhibiting drugs and also by the extrinsic factors such as cancer, inflammation, and older age. By contrast, phenoconversion from a lower metabolizer phenotype into a higher metabolizer phenotype was reported by the concomitant use of CYP inducer drugs and also by smoking. In addition, alcohol, pregnancy, and vitamin D exposure may also contribute to the phenoconversion process (Klomp et al., 2020).
If any patients are taking COVID-19 therapeutics influenced by the CYP metabolism and taking CYP inhibitors, also carrying CYP genetic variants, then the net clinical effects may be further exacerbated profoundly. There is evidence for such phenomenon as reported in a recent meta-analysis showing that for patients carrying CYP2C19 LoF alleles and taking clopidogrel and proton pump inhibitors, the risk of MACE was almost over double compared to the patients taking clopidogrel with or without CYP2C19 LoF alleles (Biswas et al., 2021b). Therefore, DDIs are very important clinical considerations for optimizing safety or effectiveness of COVID-19 medications through assessing pharmacogenomic interventions.
12.2 Patients’ Condition and Underlying Diseases
Systemic vascular inflammation and coagulopathy resulting from cytokine storm contribute to multi-organ failure in patients with severe COVID-19. In addition to the genetic biomarker, that is, HLA genotype, the non-genetic biomarkers associated with the severity and disease progression of COVID-19 can be divided into 1) hematological biomarkers [lymphocyte count, neutrophil count, and neutrophil–lymphocyte ratio (NLR)], 2) inflammatory biomarkers [C-reactive protein (CRP), erythrocyte sedimentation rate (ESR), and procalcitonin (PCT)], 3) immunological biomarkers (IL-6 and IL-10), 4) biochemical biomarkers [D-dimer, troponin, creatine kinase (CK), and aspartate aminotransferase (AST)], and 5) new laboratory biomarkers (homocysteine and angiotensin II) (Ponti et al., 2020a). Almost all these biomarkers are previously used to monitor the critically ill patients with systemic infection/inflammation or multi-organ failure from several causes.
The levels of cytokine storm are associated with COVID-19 severity and severe progression. Among them, IL-6 and IL-10 could be used as biomarkers for fast diagnosis of patients with a higher risk of disease deterioration (Han et al., 2020). The identification of IL-6 might also potentially benefit from anti-IL-6 immunotherapies with tocilizumab (Zhang et al., 2020a).
The level of D-dimer or fibrin degradation products (FDPs) is a strong evidence of thrombosis and thromboembolism (Halaby et al., 2015). Studies have reported an increase in D-dimer and fibrinogen concentrations in the early stages of COVID-19 disease; a 3 to 4-fold rise in D-dimer levels is linked to poor prognosis (Rostami and Mansouritorghabeh, 2020). Monitoring the level of D-dimer might help in determining the prognosis of patients and making the decision of early aggressive treatment.
Plasma levels of homocysteine are associated with vascular inflammation and damage (Balint et al., 2020). Recent data demonstrated a predictive value of homocysteine for the severity of pneumonia from COVID-19 (Ponti et al., 2020b). The high plasma level of Ang II also has been demonstrated in COVID-19 patients with severe lung injury and high viral load (Gheblawi et al., 2020; Liu et al., 2020). When we get further clinical validation, these two new biomarkers would be useful to predict or determine the severity of pneumonia in COVID-19 patients.
12.3 Drug–Herb Interactions
Medicinal plants often serve as a very crucial alternative or adjuvant therapy to synthetic allopathic drugs for combating numerous diseases from the ancient time. Andrographolide isolated form the medicinal plant of Andrographis paniculata has been clinically used for the treatment of inflammatory diseases and viral infections for many years. Recent molecular docking suggests that andrographolide is able to form a covalent bond with the active site of SARS-CoV-2 and may suppress the progression of this pandemic virus. This herbal component may therefore serve as an alternative option for the management of the COVID-19 pandemic (Shi et al., 2020b).
However, interaction of andrographolide with other drugs called drug–herb interactions (DHIs) might be of particular interest in assessing its potency against SARS-CoV-2 infection. An in vitro study found potent inhibitory effects on the activities of CYP3A4 and CYP2C9 enzymes by andrographolide, suggesting that drug–herb interactions (DHIs) of andrographolide would be of particular concern for drugs primarily metabolized by CYP3A4 and CYP2C9 pathways such as warfarin (Pan et al., 2011). This is consistent with the findings of another study suggesting that andrographolide could cause DHIs in humans through interfering of CYP2C9 or CYP3A4 enzyme activities (Pekthong et al., 2008, 2009). DHI between andrographolide and warfarin has already been established in the mouse model where andrographolide was found to increase the systemic exposure of warfarin probably by the inhibition of the CYP3A4- or CYP2C9-mediated warfarin metabolism (Zhang et al., 2018). Another study found a DHI between andrographolide and tolbutamide where andrographolide enhanced the metabolic rate of tolbutamide by increasing the expression and activity of certain CYP enzymes (Chen et al., 2013). Also, andrographolide could also induce CYP1A1 and CYP1A2 expression as found in the mouse model (Jaruchotikamol et al., 2007), potentially interacting with drugs metabolized by CYP1A1 or CYP1A2. Many other herbs may also interact with COVID-19 therapies as shown in Table 9.
In addition of DHIs, we are also concerned about the pharmacogenetics of herbs termed as “herbogenomics,” potentially affecting the safety or efficacy of herbs. For examples, genetic polymorphisms of CYP3A4 or CYP2C9 may modify the clinical effects of andrographolide and should be considered clinically along with DHIs. Although the concept is new, it suggests to considering herbogenomics in future studies for patients taking COVID-19 medications. In addition to andrographolide, many other herbs are using against SARS-CoV-2 infection, as shown in Table 10.
Because of the antiviral activity of curcumin against various viruses, for example, HIV, Zika virus, herpes simplex virus, Chikungunya virus, hepatitis viruses, and adenovirus (Prasad and Tyagi, 2015; Mounce et al., 2017; Praditya et al., 2019), it may be potentially applied in the management of COVID-19 patients (Ho et al., 2021). A recent molecular docking study revealed that curcumin may inhibit the host entry of SARS-CoV-2 by interfering the viral S protein and host ACE2 receptor protein (Das et al., 2021b). Also, the existing antithrombotic, anti-cytokine, and antifibrotic properties of curcumin may assist in quick recovery of severe COVID-19 patients (Lelli et al., 2017; Wichmann, 2020). Future clinical studies are warranted to develop standard dosages of curcumin to assess possible clinical benefits in patients with COVID-19 (Ho et al., 2021). After compelling previous therapeutic evidence of N. sativa and recent molecular docking findings, a recent review suggests that some bioactive compounds of N. sativa, for example, α-hederin, nigellidine, and thymoquinone, could be used as alternative potential herbal drugs to treat COVID-19 (Islam et al., 2021). Another recent review has updated the current status of the naturally occurring compounds such as alkaloids, terpenes, flavonoids, and benzoquinones from different herbs against SARS-CoV-2 infection and suggested that accurate experimental investigation of these compounds may provide insightful information for the potential therapy of COVID-19 patients (Romeo et al., 2021).
13 Genetic Testing for COVID-19 Treatment: Panel of Gene Considerations
Plenty of genes of interest that may either be involved in the severity of COVID-19 progression or may potentially modify the PK/PD profiles of COVID-19 therapeutics, and may therefore potentially affect the safety or effectiveness of these medications have been identified in this review. From reviewing the previous information, we enlisted a panel of genes as two categories: 1) mandate genetic test and 2) recommendations for the genetic test.
13.1 Mandate Genetic Test
13.1.1 Mandate Genetic Test for COVID-19 Severity
As described and found evidence in this review, we strongly mandate the genetic test for HLA, ACE2, and TMPRSS2 genes for assessing the severity of COVID-19 associated with the genetic variants of these genes.
13.1.2 Mandate Theranostics
Some of the drugs used either as repurposely to combat SARS-CoV-2 infection or used as supportive care for alleviating complications associated with COVID-19 have already well-established evidence for considering pharmacogenomics interventions, and different international pharmacogenomic working groups such as Clinical Pharmacogenetics Implementation Consortium (CPIC) have provided pharmacogenomic-based dosing clinical recommendations as shown in Table 11.
At the infancy stage where almost no pharmacogenomics study of COVID-19 therapeutics in this unprecedented health situations, we strongly mandate to undertake at least theranostics for these drug–gene pairs (atazanavir–UGT1A1, ABCB1, SLCO1B1, and APOA5; efavirenz–CYP2B6; nevirapine–HLA, CYP2B6, and ABCB1; lopinavir–SLCO1B3 and ABCC2; ribavirin–SLC28A2; tocilizumab–FCGR3A; ivermectin–ABCB1; oseltamivir–CES1 and ABCB1; clopidogrel–CYP2C19 and ABCB1, warfarin–CYP2C9 and VKORC1; NSAIDs–CYP2C9) in patients with COVID-19 based on the evidence of drug–gene interactions for optimizing the safety or efficacy of COVID-19 therapies.
13.2 Recommendations for Theranostics
After evaluating PK properties and low evidence of pharmacogenomic associations, we recommend these drug–gene pairs (remdesivir–CES1, CYP2C8, CYP3A4, and CYP2D6; azithromycin–ABCB1; losartan–ABCB1 and CYP2C9; lopinavir/ritonavir–ABCB1) for further considerations in clinical studies to establish evidence for genetic associations with the safety or effectiveness of COVID-19 medications.
It is reasonable to understand that at the beginning of emergency pandemic situations, clinicians may not be able to prioritize pharmacogenomics intervention issues of COVID-19 drugs; probably that might be one of the best reasons for higher mortality of COVID-19 patients due to not well clinically managed of these patients. Also, although pharmacogenomics is starting to incorporate into routine clinical practice in some parts of the world, for example, United States, Thailand, United Kingdom, and Netherlands, clinicians still are not well-positioned to consider pharmacogenomics recommendations due to either low understanding of this newly evolving area or may not have adequate training regarding the pharmacogenomic uptake in the clinical practice. However, COVID-19 situations are stabilizing slowly, and this is the high time for clinicians/genomics researchers to investigate pharmacogenomics associations of drugs in this clinical condition.
Since no pharmacogenomic study assessing the associations of COVID-19 therapeutics with the safety or efficacy has not been either undertaken or published yet, we suggest to consider a panel of genes of interest which have already been discussed above in this review to assess the impacts of pharmacogenomics in COVID-19 therapeutics to establish precision medicine of COVID-19, as illustrated in Figure 6.
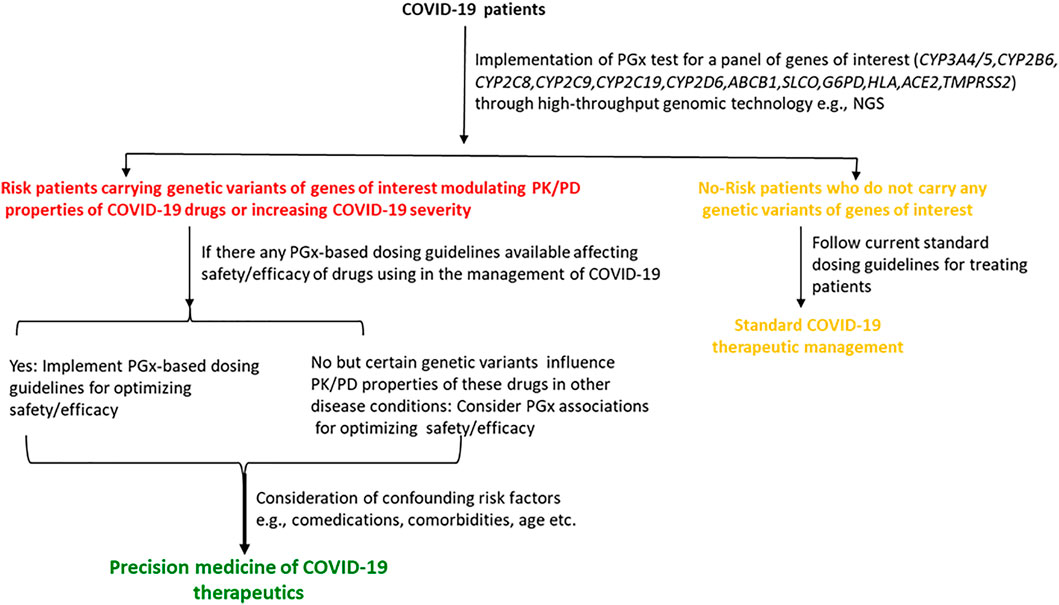
FIGURE 6. Implementation of precision medicine for drugs used in the management of COVID-19. Here, COVID-19, coronavirus disease-2019; NGS, next-generation sequencing; PK, pharmacokinetics; PD, pharmacodynamics.
14 Pharmacogenomics and Precision Medicine for COVID-19 in Thailand
Thai population might be at particular risk for either developing severe COVID-19 due to the HLA genetics or developing toxicities/therapeutic ineffectiveness by COVID-19 drugs. This is partly because Thai population has great diversity of HLA, transporters, and CYP genetic variants. It has been previously reported that ∼25% of the Thai population carried HLA-DQA1/HLA-DQB1 genetic polymorphisms (Puangpetch et al., 2014; Satapornpong et al., 2020), which might render COVID-19 severity. Minor allele frequencies of the CYP2C9*2 and CYP2C9*3 in Thai population were 0.08 and 5.3%, respectively. Minor allele frequencies of the CYP2C19*2, CYP2C19*3, and CYP2C19*17 in Thai population were 25.6, 2.5, and 1.8%, respectively. Approximately 30% of the CYP3A4 variant allele was identified in the Thai population as reported elsewhere (Sukprasong et al., 2021). Over 30% Thai population carried C3435T ABCB1 genetic polymorphisms as revealed in a previous study conducted in Thailand (Sensorn et al., 2013). Overall, it is concluded that considerable proportion of Thai population might be at risk of either severe COVID-19 manifestation or might be at risk of developing toxicities/ineffectiveness of the COVID-19 medications due to carrying these genetic variants. Moreover, the herbs especially andrographolide and the others as described in this review are commonly used in this population; this might also render the risk of toxicities/ineffectiveness of these herbs due to either DHIs or herbogenomics.
15 Clinical Perspective
To our best knowledge, no clinical studies were identified in the literature to date that had assessed either metabolic or transporter genetic variants with the safety or effectiveness of current COVID-19 therapeutics. This is creating evidence impasse and delaying the target for finding appropriate therapeutics to combat COVID-19 successfully. From considering the PK/PD profiles of the current COVID-19 therapeutics under investigation as discussed in this review, it is emerging the needs for assessing genetic associations of the relevant metabolic or transporter genes of interest for optimizing the safety or effectiveness of COVID-19 therapeutics. Future clinical studies or trials are warranted to investigate such genetic associations for the achievement of precision medicine for COVID-19. Since it is well evidenced that the mortality is significantly higher in older people and having comorbidities (Biswas et al., 2021a), DDIs should also be considered in these assessments because of vulnerability to polypharmacy. Ideally, considerations of multifactorial drug–gene interactions (DGIs) of COVID-19 therapeutics may accelerate the development of precision medicine of COVID-19 in the real clinical settings as shown in Figure 7, as established in other therapeutic areas such as antiplatelets (Biswas et al., 2021b).
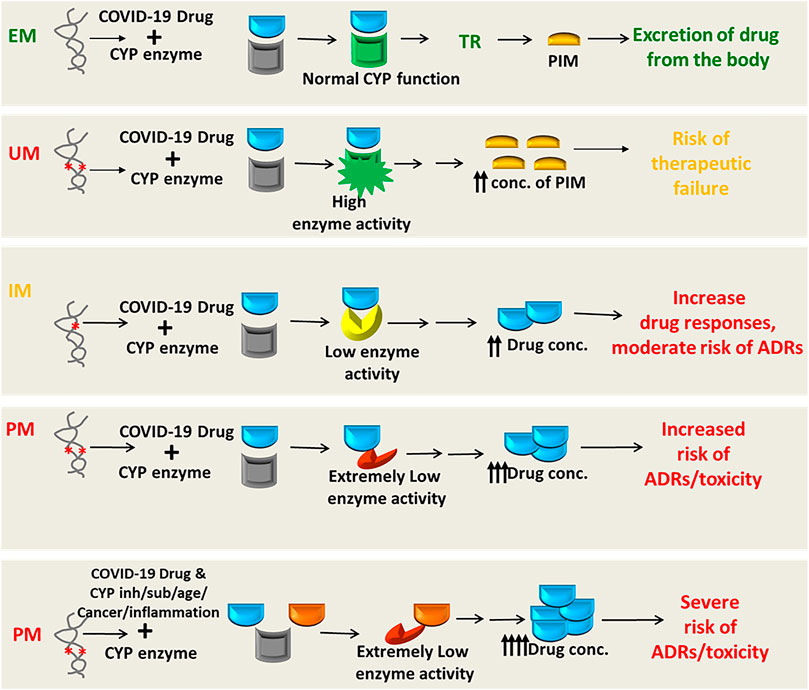
FIGURE 7. Predictive model of multifactorial DGIs for a COVID-19 drug showing possible effects of CYP gene variants representing likely phenotypes associated with the risk of therapeutic failure or ADRs/toxicity. Here, DGIs, drug–gene interactions; COVID-19, coronavirus disease-2019; EM, extensive metabolizer; UM, ultrarapid metabolizer; IM, intermediate metabolizer; PM, poor metabolizer; CYP, cytochrome P450 enzyme; *indicates CYP gene variant; Inh, inhibitor; Subs, substrate; TR, therapeutic response; PIM, pharmacologically inactive metabolite; ADRs, adverse drug reactions; conc., concentration.
It is very important to note here that this predictive model has considered only a pharmacologically active drug involving CYP metabolism; however, in case of a prodrug, the effects will be vice versa, and this model is also applicable to other genes, for example, transporter genes affecting the safety or efficacy of COVID-19 drugs.
16 Conclusion
The global outbreak of SARS-CoV-2 has evolved into an emergent COVID-19 pandemic causing huge morbidity and mortality in the world. Many drugs without establishing clinical effectiveness or tailoring safety are being administered to tackle COVID-19 pandemic situations. A repurposing strategy might be more effective and successful if pharmacogenetic interventions of these drugs are being considered in future clinical studies/trials. Safety and effectiveness of several repurposed drugs currently being used for the management of COVID-19 may be affected by the CYP/transporter genetic variants. From reviewing the current evidence of pharmacogenetic of these drugs in either COVID-19 or other diseases, we strongly mandate to undertake pharmacogenetic assessment for at least these drug–gene pairs (atazanavir–UGT1A1, ABCB1, SLCO1B1, and APOA5; efavirenz–CYP2B6; nevirapine–HLA, CYP2B6, and ABCB1; lopinavir–SLCO1B3 and ABCC2; ribavirin–SLC28A2; tocilizumab–FCGR3A; ivermectin–ABCB1; oseltamivir–CES1 and ABCB1; clopidogrel–CYP2C19 and ABCB1, warfarin–CYP2C9 and VKORC1; NSAIDs–CYP2C9) in patients with COVID-19 for advancing precision COVID-19 therapeutics by optimizing the safety or effectiveness of these drugs.
Although it is very unlikely that there are almost no pharmacogenetic data for COVID-19 drugs, from inferring the PK/PD properties and some pharmacogenetic evidence of these drugs in other diseases/clinical conditions, it is highly likely that pharmacogenetic associations are also feasible in at least some COVID-19 drugs currently being administered as shown in this review and should be considered in future clinical studies/trials. Molecular docking and computational studies are promising to achieve new COVID-19 therapies as shown in this review. Current situation in the discovery of anti-SARS-CoV-2 agents at four important targets from in silico studies has been described and summarized in this review. Although naturally occurring compounds from different herbs against SARS-CoV-2 infection are favorable, accurate experimental investigation of these compounds is warranted to provide insightsful information. Moreover, clinical considerations of DDIs and DHIs of the existing repurposed drugs along with pharmacogenetic (e.g., efavirenz and CYP2B6) and herbogenetic (e.g., andrographolide and CYP2C9) interventions, collectively called multifactorial drug-gene interactions (DGIs), may further accelerate the development of precision COVID-19 therapies in the real-world clinical settings.
Author Contributions
CS contributed to the conception, and designed and reviewed the manuscript. MB, NS, and TR contributed to the writing of the manuscript. NS, KS, MB, and ME drew the figures and summarized the tables. All authors contributed to the article and approved the submitted version.
Funding
This study was supported by grants from the 1) Mahidol University International Postdoctoral Fellowship, Mahidol University; 2) Faculty of Medicine, Ramathibodi Hospital, Mahidol University; 3) the Health System Research Institute under Genomics Thailand Strategic Fund; and 4) the International Research Network-the Thailand Research Fund (No. IRN60W003).
Conflict of Interest
The authors declare that the research was conducted in the absence of any commercial or financial relationships that could be construed as a potential conflict of interest.
Publisher’s Note
All claims expressed in this article are solely those of the authors and do not necessarily represent those of their affiliated organizations, or those of the publisher, the editors, and the reviewers. Any product that may be evaluated in this article, or claim that may be made by its manufacturer, is not guaranteed or endorsed by the publisher.
Acknowledgments
The authors thank the staff of Pharmacogenomic and Personalized Medicine of Ramathibodi Hospital.
References
Ackermann, M., Verleden, S. E., Kuehnel, M., Haverich, A., Welte, T., and Laenger, F. (2020). Pulmonary Vascular Endothelialitis, Thrombosis, and Angiogenesis in Covid-19. N. Engl. J. Med. 383, 120–128. doi:doi:10.1056/NEJMoa2015432
Ackermann, M., Werlein, C., Länger, F., Kühnel, M. P., and Jonigk, D. D. (2021). COVID-19: Effects on the Lungs and Heart. Pathologe 42, 164–171. doi:10.1007/s00292-021-00918-9
Adedeji, A. O., Severson, W., Jonsson, C., Singh, K., Weiss, S. R., and Sarafianos, S. G. (2013). Novel Inhibitors of Severe Acute Respiratory Syndrome Coronavirus Entry that Act by Three Distinct Mechanisms. J. Virol. 87, 8017–8028. doi:10.1128/JVI.00998-13
Agarwal, A., Rochwerg, B., Lamontagne, F., Siemieniuk, R. A., Agoritsas, T., Askie, L., et al. (2020). A Living WHO Guideline on Drugs for Covid-19. BMJ 370, m3379. doi:10.1136/bmj.m3379
Ahmad, B., Batool, M., Ain, Q. U., Kim, M. S., and Choi, S. (2021). Exploring the Binding Mechanism of PF-07321332 SARS-CoV-2 Protease Inhibitor through Molecular Dynamics and Binding Free Energy Simulations. Int. J. Mol. Sci. 22. doi:10.3390/ijms22179124
Ahmed-Hassan, H., Sisson, B., Shukla, R. K., Wijewantha, Y., Funderburg, N. T., Li, Z., et al. (2020). Innate Immune Responses to Highly Pathogenic Coronaviruses and Other Significant Respiratory Viral Infections. Front. Immunol. 11, 1979. doi:10.3389/fimmu.2020.01979
Al-Sanea, M. M., Abelyan, N., Abdelgawad, M. A., Musa, A., Ghoneim, M. M., Al-Warhi, T., et al. (2021). Strawberry and Ginger Silver Nanoparticles as Potential Inhibitors for SARS-CoV-2 Assisted by In Silico Modeling and Metabolic Profiling. Antibiot 10. doi:10.3390/antibiotics10070824
Alavian, G., Kolahdouzan, K., Mortezazadeh, M., and Torabi, Z. S. (2021). Antiretrovirals for Prophylaxis against COVID-19: A Comprehensive Literature Review. J. Clin. Pharmacol. 61, 581–590. doi:10.1002/jcph.1788
Alizadehsani, R., Alizadeh Sani, Z., Behjati, M., Roshanzamir, Z., Hussain, S., Abedini, N., et al. (2021). Risk Factors Prediction, Clinical Outcomes, and Mortality in COVID-19 Patients. J. Med. Virol. 93, 2307–2320. doi:10.1002/jmv.26699
Allegra, S., Cusato, J., De Nicolò, A., Boglione, L., Gatto, A., Cariti, G., et al. (2015). Role of Pharmacogenetic in Ribavirin Outcome Prediction and Pharmacokinetics in an Italian Cohort of HCV-1 and 4 Patients. Biomed. Pharmacother. 69, 47–55. doi:10.1016/j.biopha.2014.10.030
Amanat, F., and Krammer, F. (2020). SARS-CoV-2 Vaccines: Status Report. Immunity 52, 583–589. doi:10.1016/j.immuni.2020.03.007
Ambrocio-Ortiz, E., Pérez-Rubio, G., Del Ángel-Pablo, A. D., Buendía-Roldán, I., Chávez-Galán, L., Hernández-Zenteno, R. de. J., et al. (2021). Angiotensin-Converting Enzyme 2 (ACE2) in the Context of Respiratory Diseases and its Importance in Severe Acute Respiratory Syndrome Coronavirus 2 (SARS-CoV-2) Infection. Pharmaceuticals (Basel) 14. doi:10.3390/ph14080805
Amin, S. A., Banerjee, S., Ghosh, K., Gayen, S., and Jha, T. (2021). Protease Targeted COVID-19 Drug Discovery and its Challenges: Insight into Viral Main Protease (Mpro) and Papain-like Protease (PLpro) Inhibitors. Bioorg. Med. Chem. 29, 115860. doi:10.1016/j.bmc.2020.115860
Amin, S. A., and Jha, T. (2020). Fight against Novel Coronavirus: A Perspective of Medicinal Chemists. Eur. J. Med. Chem. 201, 112559. doi:10.1016/j.ejmech.2020.112559
Angamo, M. T., Mohammed, M. A., and Peterson, G. M. (2021). Efficacy and Safety of Remdesivir in Hospitalised COVID-19 Patients: a Systematic Review and Meta-Analysis. Infection, 1–15. doi:10.1007/s15010-021-01671-0
Arun, K. G., Sharanya, C. S., Abhithaj, J., Francis, D., and Sadasivan, C. (2021). Drug Repurposing against SARS-CoV-2 Using E-Pharmacophore Based Virtual Screening, Molecular Docking and Molecular Dynamics with Main Protease as the Target. J. Biomol. Struct. Dyn. 39, 4647–4658. doi:10.1080/07391102.2020.1779819
Aruna, D., and Naidu, M. U. R. (2007). Pharmacodynamic Interaction Studies of Ginkgo Biloba with Cilostazol and Clopidogrel in Healthy Human Subjects. Br. J. Clin. Pharmacol. 63, 333–338. doi:10.1111/j.1365-2125.2006.02759.x
Asher, G. N., Corbett, A. H., and Hawke, R. L. (2017). Common Herbal Dietary Supplement-Drug Interactions. Am. Fam. Physician 96, 101–107.
Aspiroz, E. L., Cabrera Figueroa, S. E., Cruz, R., Porras Hurtado, G. L., Martín, A. F., Hurlé, A. D.-G., et al. (2014). Toxicogenetics of Lopinavir/ritonavir in HIV-Infected European Patients. Per. Med. 11, 263–272. doi:10.2217/pme.14.7
Atasilp, C., Chansriwong, P., Sirachainan, E., Reungwetwattana, T., Sirilerttrakul, S., Chamnanphon, M., et al. (2020). Effect of Drug Metabolizing Enzymes and Transporters in Thai Colorectal Cancer Patients Treated with Irinotecan-Based Chemotherapy. Sci. Rep. 10, 13486. doi:10.1038/s41598-020-70351-0
Attaway, A. H., Scheraga, R. G., Bhimraj, A., Biehl, M., and Hatipoğlu, U. (2021). Severe Covid-19 Pneumonia: Pathogenesis and Clinical Management. BMJ 372, n436. doi:10.1136/bmj.n436
Badary, O. A. (2021). Pharmacogenomics and COVID-19: Clinical Implications of Human Genome Interactions with Repurposed Drugs. Pharmacogenomics J. 21, 275–284. doi:10.1038/s41397-021-00209-9
Bafna, K., White, K., Harish, B., Rosales, R., Ramelot, T. A., Acton, T. B., et al. (2021). Hepatitis C Virus Drugs that Inhibit SARS-CoV-2 Papain-like Protease Synergize with Remdesivir to Suppress Viral Replication in Cell Culture. Cell Rep 35, 109133. doi:10.1016/j.celrep.2021.109133
Bahadur Gurung, A., Ajmal Ali, M., Lee, J., Abul Farah, M., Mashay Al-Anazi, K., and Al-Hemaid, F. (2021). Identification of SARS-CoV-2 Inhibitors from Extracts of Houttuynia Cordata Thunb. Saudi J. Biol. Sci. doi:10.1016/j.sjbs.2021.08.100
Baig, M. H., Sharma, T., Ahmad, I., Abohashrh, M., Alam, M. M., and Dong, J.-J. (2021). Is PF-00835231 a Pan-SARS-CoV-2 Mpro Inhibitor? A Comparative Study. Molecules 26. doi:10.3390/molecules26061678
Bakhshandeh, B., Sorboni, S. G., Javanmard, A.-R., Mottaghi, S. S., Mehrabi, M.-R., Sorouri, F., et al. (2021). Variants in ACE2; Potential Influences on Virus Infection and COVID-19 Severity. Infect. Genet. Evol. J. Mol. Epidemiol. Evol. Genet. Infect. Dis. 90, 104773. doi:10.1016/j.meegid.2021.104773
Balint, B., Jepchumba, V. K., Guéant, J.-L., and Guéant-Rodriguez, R.-M. (2020). Mechanisms of Homocysteine-Induced Damage to the Endothelial, Medial and Adventitial Layers of the Arterial wall. Biochimie 173, 100–106. doi:10.1016/j.biochi.2020.02.012
Baral, R., White, M., and Vassiliou, V. S. (2020). Effect of Renin-Angiotensin-Aldosterone System Inhibitors in Patients with COVID-19: a Systematic Review and Meta-Analysis of 28,872 Patients. Curr. Atheroscler. Rep. 22, 61. doi:10.1007/s11883-020-00880-6
Barnes, B. J., Adrover, J. M., Baxter-Stoltzfus, A., Borczuk, A., Cools-Lartigue, J., Crawford, J. M., et al. (2020). Targeting Potential Drivers of COVID-19: Neutrophil Extracellular Traps. J. Exp. Med. 217. doi:10.1084/jem.20200652
Baudou, E., Lespine, A., Durrieu, G., André, F., Gandia, P., Durand, C., et al. (2020). Serious Ivermectin Toxicity and Human ABCB1 Nonsense Mutations. N. Engl. J. Med. 383, 787–789. doi:10.1056/NEJMc1917344
Becker, R. C. (2020). Covid-19 Treatment Update: Follow the Scientific Evidence. J. Thromb. Thrombolysis 50, 43–53. doi:10.1007/s11239-020-02120-9
Benetti, E., Tita, R., Spiga, O., Ciolfi, A., Birolo, G., Bruselles, A., et al. (2020). ACE2 Gene Variants May Underlie Interindividual Variability and Susceptibility to COVID-19 in the Italian Population. Eur. J. Hum. Genet. 28, 1602–1614. doi:10.1038/s41431-020-0691-z
Bergmann, C. C., and Silverman, R. H. (2020). COVID-19: Coronavirus Replication, Pathogenesis, and Therapeutic Strategies. Cleve. Clin. J. Med. 87, 321–327. doi:10.3949/ccjm.87a.20047
Bermúdez de León, M., León-Cachón, R. B. R., Silva-Ramírez, B., González-Ríos, R. N., Escobedo-Guajardo, B., Leyva-Parra, R., et al. (2020). Association Study of Genetic Polymorphisms in Proteins Involved in Oseltamivir Transport, Metabolism, and Interactions with Adverse Reactions in Mexican Patients with Acute Respiratory Diseases. Pharmacogenomics J. 20, 613–620. doi:10.1038/s41397-020-0151-8
Berretta, A. A., Silveira, M. A. D., Cóndor Capcha, J. M., and De Jong, D. (2020). Propolis and its Potential against SARS-CoV-2 Infection Mechanisms and COVID-19 Disease: Running Title: Propolis against SARS-CoV-2 Infection and COVID-19. Biomed. Pharmacother. 131, 110622. doi:10.1016/j.biopha.2020.110622
Bikdeli, B., Madhavan, M. V., Jimenez, D., Chuich, T., Dreyfus, I., Driggin, E., et al. (2020). COVID-19 and Thrombotic or Thromboembolic Disease: Implications for Prevention, Antithrombotic Therapy, and Follow-Up: JACC State-Of-The-Art Review. J. Am. Coll. Cardiol. 75, 2950–2973. doi:10.1016/j.jacc.2020.04.031
Biswas, M., Kali, M. S. K., Biswas, T. K., and Ibrahim, B. (2020a). Risk of Major Adverse Cardiovascular Events of CYP2C19 Loss-Of-Function Genotype Guided Prasugrel/ticagrelor vs Clopidogrel Therapy for Acute Coronary Syndrome Patients Undergoing Percutaneous Coronary Intervention: a Meta-Analysis. Platelets. doi:10.1080/09537104.2020.1792871
Biswas, M., Author, F., Sumaiya Khatun Kali, M., Tapash Kumar Biswas, Mp., and Baharudin Ibrahim, M. (2022). Association of CYP2C19 Loss-Of-Function Alleles with Major Adverse Cardiovascular Events of Clopidogrel in Acute Coronary Syndrome Patients Undergoing Percutaneous Coronary Intervention: Meta-Analysis. Pharmacogenomics. doi:10.2217/pgs-2021-0098
Biswas, M. (2021a). Global Distribution of CYP2C19 Risk Phenotypes Affecting Safety and Effectiveness of Medications. Pharmacogenomics J. 21, 190–199. doi:10.1038/s41397-020-00196-3
Biswas, M., and Kali, M. S. K. (2021a). Association of Angiotensin-Converting Enzyme Inhibitors and Angiotensin-Receptor Blockers with Risk of Mortality, Severity or SARS-CoV-2 Test Positivity in COVID-19 Patients: Meta-Analysis. Sci. Rep. 11, 5012. doi:10.1038/s41598-021-84678-9
Biswas, M., and Kali, S. K. (2021b). Association of CYP2C19 Loss-Of-Function Alleles with Major Adverse Cardiovascular Events of Clopidogrel in Stable Coronary Artery Disease Patients Undergoing Percutaneous Coronary Intervention: Meta-Analysis. Cardiovasc. Drugs Ther. doi:10.1007/s10557-021-07142-w
Biswas, M. (2020). Potential Clinically Significant Life-Threatening Drug–Drug Interactions of Lopinavir and Ritonavir Used in the Treatment of COVID-19. Exp. Results 1, e49. doi:10.1017/exp.2020.53
Biswas, M. (2021b). Predictive Association of ABCB1 C3435T Genetic Polymorphism with the Efficacy or Safety of Lopinavir and Ritonavir in COVID-19 Patients. Pharmacogenomics 22, 375–381. doi:10.2217/pgs-2020-0096
Biswas, M. (2021c). Predictive Prevalence of Risk Phenotypes Associated with Rs2285666 Single Nucleotide Polymorphism of ACE2 in the World Population Susceptible to SARS-CoV-2 Infection. J. Viro Res. Rep. 2, 1–4. doi:10.47363/jvrr/2021(2)129
Biswas, M., Rahaman, S., Biswas, T. K., Haque, Z., and Ibrahim, B. (2021a). Association of Sex, Age, and Comorbidities with Mortality in COVID-19 Patients: A Systematic Review and Meta-Analysis. Intervirology 64, 36–47. doi:10.1159/000512592
Biswas, M., Rahaman, S., Biswas, T. K., and Ibrahim, B. (2020b). Effects of the ABCB1 C3435T Single Nucleotide Polymorphism on Major Adverse Cardiovascular Events in Acute Coronary Syndrome or Coronary Artery Disease Patients Undergoing Percutaneous Coronary Intervention and Treated with Clopidogrel: A Systematic Revie. Expert Opin. Drug Saf. 19, 1605–1616. doi:10.1080/14740338.2020.1836152
Biswas, M., Rahaman, S., Biswas, T. K., and Ibrahim, B. (2021b). Risk of Major Adverse Cardiovascular Events for Concomitant Use of Clopidogrel and Proton Pump Inhibitors in Patients Inheriting CYP2C19 Loss-Of-Function Alleles: Meta-Analysis. Int. J. Clin. Pharm. 43, 1360–1369. doi:10.1007/s11096-021-01261-y
Biswas, M., and Roy, D. N. (2021). Potential Clinically Significant Drug-Drug Interactions of Hydroxychloroquine Used in the Treatment of COVID-19. Int. J. Clin. Pract., e14710. doi:10.1111/ijcp.14710
Bonora, S., Rusconi, S., Calcagno, A., Bracchi, M., Viganò, O., Cusato, J., et al. (2015). Successful Pharmacogenetics-Based Optimization of Unboosted Atazanavir Plasma Exposure in HIV-Positive Patients: a Randomized, Controlled, Pilot Study (The REYAGEN Study). J. Antimicrob. Chemother. 70, 3096–3099. doi:10.1093/jac/dkv208
Br, B., Damle, H., Ganju, S., and Damle, L. (2020). In Silico screening of Known Small Molecules to Bind ACE2 Specific RBD on Spike Glycoprotein of SARS-CoV-2 for Repurposing against COVID-19. F1000Research 9, 663. doi:10.12688/f1000research.24143.1
Burrows, F. S., Carlos, L. M., Benzimra, M., Marriott, D. J. E., Havryk, A. P., Plit, M. L., et al. (2015). Oral Ribavirin for Respiratory Syncytial Virus Infection after Lung Transplantation: Efficacy and Cost-Efficiency. J. Hear. Lung Transpl. Off. Publ. Int. Soc. Hear. Transpl. 34, 958–962. doi:10.1016/j.healun.2015.01.009
Cafiero, C., Re, A., Micera, A., Palmirotta, R., Monaco, D., Romano, F., et al. (2020). Pharmacogenomics and Pharmacogenetics: In Silico Prediction of Drug Effects in Treatments for Novel Coronavirus SARS-CoV2 Disease. Pharmgenomics. Pers. Med. 13, 463–484. doi:10.2147/PGPM.S270069
Cao, B., Wang, Y., Wen, D., Liu, W., Wang, J., Fan, G., et al. (2020). A Trial of Lopinavir–Ritonavir in Adults Hospitalized with Severe Covid-19. N. Engl. J. Med. 382, 1787–1799. doi:10.1056/nejmoa2001282
Chen, H.-W., Huang, C.-S., Liu, P.-F., Li, C.-C., Chen, C.-T., Liu, C.-T., et al. (20132013). Andrographis Paniculata Extract and Andrographolide Modulate the Hepatic Drug Metabolism System and Plasma Tolbutamide Concentrations in Rats. Evid. Based. Complement. Alternat. Med., 982689. doi:10.1155/2013/982689
Chen, N., Zhou, M., Dong, X., Qu, J., Gong, F., Han, Y., et al. (2020). Epidemiological and Clinical Characteristics of 99 Cases of 2019 Novel Coronavirus Pneumonia in Wuhan, China: a Descriptive Study. Lancet 395, 507–513. doi:10.1016/S0140-6736(20)30211-7
Chen, W., and Pan, J. Y. (2021). Anatomical and Pathological Observation and Analysis of SARS and COVID-19: Microthrombosis Is the Main Cause of Death. Biol. Proced. Online 23, 4. doi:10.1186/s12575-021-00142-y
Chen, X.-W., Sneed, K. B., Pan, S.-Y., Cao, C., Kanwar, J. R., Chew, H., et al. (2012). Herb-drug Interactions and Mechanistic and Clinical Considerations. Curr. Drug Metab. 13, 640–651. doi:10.2174/1389200211209050640
Cheng, B.-H., Zhou, X., Wang, Y., Chan, J. Y.-W., Lin, H.-Q., Or, P. M. Y., et al. (2015). Herb-drug Interaction between an Anti-HIV Chinese Herbal SH Formula and Atazanavir In Vitro and In Vivo. J. Ethnopharmacol. 162, 369–376. doi:10.1016/j.jep.2015.01.010
Cheng, F.-J., Huynh, T.-K., Yang, C.-S., Hu, D.-W., Shen, Y.-C., Tu, C.-Y., et al. (2021). Hesperidin Is a Potential Inhibitor against SARS-CoV-2 Infection. Nutrients 13. doi:10.3390/nu13082800
Choudhary, S., Sreenivasulu, K., Mitra, P., Misra, S., and Sharma, P. (2021). Role of Genetic Variants and Gene Expression in the Susceptibility and Severity of COVID-19. Ann. Lab. Med. 41, 129–138. doi:10.3343/alm.2021.41.2.129
da Costa, V. G., Moreli, M. L., and Saivish, M. V. (2020). The Emergence of SARS, MERS and Novel SARS-2 Coronaviruses in the 21st century. Arch. Virol. 165, 1517–1526. doi:10.1007/s00705-020-04628-0
da Rocha, I. M., Gasparotto, A. S., Lazzaretti, R. K., Notti, R. K., Sprinz, E., and Mattevi, V. S. (2015). Polymorphisms Associated with Renal Adverse Effects of Antiretroviral Therapy in a Southern Brazilian HIV Cohort. Pharmacogenet. Genomics 25, 541–547. doi:10.1097/FPC.0000000000000169
Daoud, S., Alabed, S. J., and Dahabiyeh, L. A. (2021). Identification of Potential COVID-19 Main Protease Inhibitors Using Structure-Based Pharmacophore Approach, Molecular Docking and Repurposing Studies. Acta Pharm. 71, 163–174. doi:10.2478/acph-2021-0016
Das, S. K., Mahanta, S., Tanti, B., Tag, H., and Hui, P. K. (2021a). Identification of Phytocompounds from Houttuynia Cordata Thunb. As Potential Inhibitors for SARS-CoV-2 Replication Proteins through GC-MS/LC-MS Characterization, Molecular Docking and Molecular Dynamics Simulation. Mol. Divers., 1–24. doi:10.1007/s11030-021-10226-2
Das, S., Sarmah, S., Lyndem, S., and Singha Roy, A. (2021b). An Investigation into the Identification of Potential Inhibitors of SARS-CoV-2 Main Protease Using Molecular Docking Study. J. Biomol. Struct. Dyn. 39, 3347–3357. doi:10.1080/07391102.2020.1763201
David, A. B., Diamant, E., Dor, E., Barnea, A., Natan, N., Levin, L., et al. (2021). Identification of SARS-CoV-2 Receptor Binding Inhibitors by In Vitro Screening of Drug Libraries. Molecules 26. doi:10.3390/molecules26113213
D’Avolio, A., Cusato, J., De Nicolò, A., Allegra, S., and Di Perri, G. (2016). Pharmacogenetics of Ribavirin-Induced Anemia in HCV Patients. Pharmacogenomics 17, 925–941. doi:10.2217/pgs.16.22
de Ligt, M., Hesselink, M. K. C., Jorgensen, J., Hoebers, N., Blaak, E. E., and Goossens, G. H. (2021). Resveratrol Supplementation Reduces ACE2 Expression in Human Adipose Tissue. Adipocyte 10, 408–411. doi:10.1080/21623945.2021.1965315
de Wit, E., van Doremalen, N., Falzarano, D., and Munster, V. J. (2016). SARS and MERS: Recent Insights into Emerging Coronaviruses. Nat. Rev. Microbiol. 14, 523–534. doi:10.1038/nrmicro.2016.81
Deb, S., Reeves, A. A., Hopefl, R., and Bejusca, R. (2021). ADME and Pharmacokinetic Properties of Remdesivir: Its Drug Interaction Potential. Pharmaceuticals (Basel) 14. doi:10.3390/ph14070655
Debnath, P., Bhaumik, S., Sen, D., Muttineni, R. K., and Debnath, S. (2021). Identification of SARS-CoV-2 Main Protease Inhibitors Using Structure Based Virtual Screening and Molecular Dynamics Simulation of DrugBank Database. ChemistrySelect 6, 4991–5013. doi:10.1002/slct.202100854
Deetanya, P., Hengphasatporn, K., Wilasluck, P., Shigeta, Y., Rungrotmongkol, T., and Wangkanont, K. (2021). Interaction of 8-Anilinonaphthalene-1-Sulfonate with SARS-CoV-2 Main Protease and its Application as a Fluorescent Probe for Inhibitor Identification. Comput. Struct. Biotechnol. J. 19, 3364–3371. doi:10.1016/j.csbj.2021.05.053
Deng, P., Zhong, D., Yu, K., Zhang, Y., Wang, T., and Chen, X. (2013). Pharmacokinetics, Metabolism, and Excretion of the Antiviral Drug Arbidol in Humans. Antimicrob. Agents Chemother. 57, 1743–1755. doi:10.1128/AAC.02282-12
Deng, Y., Mo, Y.-F., Chen, X.-M., Zhang, L.-Z., Liao, C.-F., Song, Y., et al. (2016). Effect of Ginkgo Biloba Extract on the Pharmacokinetics and Metabolism of Clopidogrel in Rats. Phytother. Res. 30, 1886–1892. doi:10.1002/ptr.5691
Desta, Z., Gammal, R. S., Gong, L., Whirl-Carrillo, M., Gaur, A. H., Sukasem, C., et al. (2019). Clinical Pharmacogenetics Implementation Consortium (CPIC) Guideline for CYP2B6 and Efavirenz-Containing Antiretroviral Therapy. Clin. Pharmacol. Ther. 106, 726–733. doi:10.1002/cpt.1477
Diomede, L., Beeg, M., Gamba, A., Fumagalli, O., Gobbi, M., and Salmona, M. (2021). Can Antiviral Activity of Licorice Help Fight COVID-19 Infection? Biomolecules 11. doi:10.3390/biom11060855
Donma, M. M., and Donma, O. (2020). The Effects of Allium Sativum on Immunity within the Scope of COVID-19 Infection. Med. Hypotheses 144, 109934. doi:10.1016/j.mehy.2020.109934
Du, L., He, Y., Zhou, Y., Liu, S., Zheng, B.-J., and Jiang, S. (2009). The Spike Protein of SARS-CoV-Aa Target for Vaccine and Therapeutic Development. Nat. Rev. Microbiol. 7, 226–236. doi:10.1038/nrmicro2090
Du, P., Wang, A., Ma, Y., and Li, X. (2019). Association between the UGT1A1*28 Allele and Hyperbilirubinemia in HIV-Positive Patients Receiving Atazanavir: a Meta-Analysis. Biosci. Rep. 39. doi:10.1042/BSR20182105
Du, Y. X., and Chen, X. P. (2020). Favipiravir: Pharmacokinetics and Concerns about Clinical Trials for 2019-nCoV Infection. Clin. Pharmacol. Ther. doi:10.1002/cpt.1844
Eastman, R. T., Roth, J. S., Brimacombe, K. R., Simeonov, A., Shen, M., Patnaik, S., et al. (2020). Remdesivir: A Review of its Discovery and Development Leading to Emergency Use Authorization for Treatment of COVID-19. ACS Cent. Sci. doi:10.1021/acscentsci.0c00489
Ehteshami, M., Tao, S., Zandi, K., Hsiao, H.-M., Jiang, Y., Hammond, E., et al. (2017). Characterization of β-d-N(4)-Hydroxycytidine as a Novel Inhibitor of Chikungunya Virus. Antimicrob. Agents Chemother. 61. doi:10.1128/AAC.02395-16
El Hassab, M. A., Shoun, A. A., Al-Rashood, S. T., Al-Warhi, T., and Eldehna, W. M. (2020). Identification of a New Potential SARS-COV-2 RNA-dependent RNA Polymerase Inhibitor via Combining Fragment-Based Drug Design, Docking, Molecular Dynamics, and MM-PBSA Calculations. Front. Chem. 8, 584894. doi:10.3389/fchem.2020.584894
Elfiky, A. A. (2020). Anti-HCV, Nucleotide Inhibitors, Repurposing against COVID-19. Life Sci. 248, 117477. doi:10.1016/j.lfs.2020.117477
Elsawah, H. K., Elsokary, M. A., Abdallah, M. S., and ElShafie, A. H. (2021). Efficacy and Safety of Remdesivir in Hospitalized Covid-19 Patients: Systematic Review and Meta-Analysis Including Network Meta-Analysis. Rev. Med. Virol. 31, e2187. doi:10.1002/rmv.2187
European Medicines Agency (2020). Summary on Compassionate Use: Remdesivir Gilead. Available at: https://www.ema.europa.eu/en/documents/other/summary-compassionate-use-remdesivir-gilead_en.pdf (Accessed May 10, 2020).
Fakhar, Z., Faramarzi, B., Pacifico, S., and Faramarzi, S. (2021). Anthocyanin Derivatives as Potent Inhibitors of SARS-CoV-2 Main Protease: An In-Silico Perspective of Therapeutic Targets against COVID-19 Pandemic. J. Biomol. Struct. Dyn. 39, 6171–6183. doi:10.1080/07391102.2020.1801510
Falcão, F., Viegas, E., Carmo, I., Soares, J., Falcao, M., Solano, M., et al. (2021). A Prospective, Observational Study to Evaluate Adverse Drug Reactions in Patients with COVID-19 Treated with Remdesivir or Hydroxychloroquine: a Preliminary Report. Eur. J. Hosp. Pharm. Sci. Pract. 28, 248–253. doi:10.1136/ejhpharm-2020-002613
Fantini, J., Di Scala, C., Chahinian, H., and Yahi, N. (2020). Structural and Molecular Modelling Studies Reveal a New Mechanism of Action of Chloroquine and Hydroxychloroquine against SARS-CoV-2 Infection. Int. J. Antimicrob. Agents 55, 105960. doi:10.1016/j.ijantimicag.2020.105960
Farouk, A.-E., Baig, M. H., Khan, M. I., Park, T., Alotaibi, S. S., and Dong, J.-J. (2021). Screening of Inhibitors against SARS-CoV-2 Spike Protein and Their Capability to Block the Viral Entry Mechanism: A Viroinformatics Study. Saudi J. Biol. Sci. 28, 3262–3269. doi:10.1016/j.sjbs.2021.02.066
Fayyazi, N., Mostashari-Rad, T., Ghasemi, J. B., Ardakani, M. M., and Kobarfard, F. (2021). Molecular Dynamics Simulation, 3D-Pharmacophore and Scaffold Hopping Analysis in the Design of Multi-Target Drugs to Inhibit Potential Targets of COVID-19. J. Biomol. Struct. Dyn., 1–22. doi:10.1080/07391102.2021.1965914
Fda, (2020). Remdesivir EUA Letter of Authorization. Available at: https://www.fda.gov/media/137564/download (Accessed May 1, 2020).
Fernández-Cruz, A., Ruiz-Antorán, B., Muñoz-Gómez, A., Sancho-López, A., Mills-Sánchez, P., Centeno-Soto, G. A., et al. (2020). A Retrospective Controlled Cohort Study of the Impact of Glucocorticoid Treatment in SARS-CoV-2 Infection Mortality. Antimicrob. Agents Chemother. 64. doi:10.1128/AAC.01168-20
Filardo, S., Di Pietro, M., Mastromarino, P., and Sessa, R. (2020). Therapeutic Potential of Resveratrol against Emerging Respiratory Viral Infections. Pharmacol. Ther. 214, 107613. doi:10.1016/j.pharmthera.2020.107613
Fintelman-Rodrigues, N., Sacramento, C. Q., Ribeiro Lima, C., Souza da Silva, F., Ferreira, A. C., Mattos, M., et al. (2020). Atazanavir, Alone or in Combination with Ritonavir, Inhibits SARS-CoV-2 Replication and Proinflammatory Cytokine Production. Antimicrob. Agents Chemother. 64. doi:10.1128/AAC.00825-20
Fiorillo, M. T., Paladini, F., Tedeschi, V., and Sorrentino, R. (2017). HLA Class I or Class II and Disease Association: Catch the Difference if You Can. Front. Immunol. 8, 1475. doi:10.3389/fimmu.2017.01475
Freitas, B. T., Durie, I. A., Murray, J., Longo, J. E., Miller, H. C., Crich, D., et al. (2020). Characterization and Noncovalent Inhibition of the Deubiquitinase and deISGylase Activity of SARS-CoV-2 Papain-like Protease. ACS Infect. Dis. 6, 2099–2109. doi:10.1021/acsinfecdis.0c00168
Fricke-Galindo, I., and Falfán-Valencia, R. (2021). Pharmacogenetics Approach for the Improvement of COVID-19 Treatment. Viruses 13. doi:10.3390/v13030413
Gammal, R. S., Court, M. H., Haidar, C. E., Iwuchukwu, O. F., Gaur, A. H., Alvarellos, M., et al. (2016). Clinical Pharmacogenetics Implementation Consortium (CPIC) Guideline for UGT1A1 and Atazanavir Prescribing. Clin. Pharmacol. Ther. 99. doi:10.1002/cpt.269
Gavriatopoulou, M., Ntanasis-Stathopoulos, I., Korompoki, E., Fotiou, D., Migkou, M., Tzanninis, I.-G., et al. (2021). Emerging Treatment Strategies for COVID-19 Infection. Clin. Exp. Med. 21, 167–179. doi:10.1007/s10238-020-00671-y
Gemmati, D., and Tisato, V. (2020). Genetic Hypothesis and Pharmacogenetics Side of Renin-Angiotensin-System in COVID-19. Genes (Basel) 11. doi:10.3390/genes11091044
Ghazwani, M. Y., Bakheit, A. H., Hakami, A. R., and Alkahtani, H. M. (2021). Virtual Screening and Molecular Docking Studies for Discovery of Potential RNA-dependent RNA Polymerase Inhibitors. Crystals 11, 471. doi:10.3390/cryst11050471
Gheblawi, M., Wang, K., Viveiros, A., Nguyen, Q., Zhong, J.-C., Turner, A. J., et al. (2020). Angiotensin-Converting Enzyme 2: SARS-CoV-2 Receptor and Regulator of the Renin-Angiotensin System: Celebrating the 20th Anniversary of the Discovery of ACE2. Circ. Res. 126, 1456–1474. doi:10.1161/CIRCRESAHA.120.317015
Glaab, E., Manoharan, G. B., and Abankwa, D. (2021). Pharmacophore Model for SARS-CoV-2 3CLpro Small-Molecule Inhibitors and In Vitro Experimental Validation of Computationally Screened Inhibitors. J. Chem. Inf. Model. 61, 4082–4096. doi:10.1021/acs.jcim.1c00258
Göktaş, M. T., Pepedil, F., Karaca, Ö., Kalkışım, S., Cevik, L., Gumus, E., et al. (2016). Relationship between Genetic Polymorphisms of Drug Efflux Transporter MDR1 (ABCB1) and Response to Losartan in Hypertension Patients. Eur. Rev. Med. Pharmacol. Sci. 20, 2460–2467.
González Canga, A., Sahagún Prieto, A. M., Diez Liébana, M. J., Fernández Martínez, N., Sierra Vega, M., and García Vieitez, J. J. (2008). The Pharmacokinetics and Interactions of Ivermectin in Humans-Aa Mini-Review. AAPS J. 10, 42–46. doi:10.1208/s12248-007-9000-9
Gordon, C. J., Tchesnokov, E. P., Schinazi, R. F., and Götte, M. (2021). Molnupiravir Promotes SARS-CoV-2 Mutagenesis via the RNA Template. J. Biol. Chem. 297, 100770. doi:10.1016/j.jbc.2021.100770
Gowrishankar, S., Muthumanickam, S., Kamaladevi, A., Karthika, C., Jothi, R., Boomi, P., et al. (2021). Promising Phytochemicals of Traditional Indian Herbal Steam Inhalation Therapy to Combat COVID-19 - an In Silico Study. Food Chem. Toxicol. Int. J. Publ. Br. Ind. Biol. Res. Assoc. 148, 111966. doi:10.1016/j.fct.2020.111966
Goyal, B., and Goyal, D. (2020). Targeting the Dimerization of the Main Protease of Coronaviruses: A Potential Broad-Spectrum Therapeutic Strategy. ACS Comb. Sci. 22, 297–305. doi:10.1021/acscombsci.0c00058
Grzybowski, B. A., Ishchenko, A. V., Shimada, J., and Shakhnovich, E. I. (2002). From Knowledge-Based Potentials to Combinatorial lead Design In Silico. Acc. Chem. Res. 35, 261–269. doi:10.1021/ar970146b
Gunst, J. D., Staerke, N. B., Pahus, M. H., Kristensen, L. H., Bodilsen, J., Lohse, N., et al. (2021). Efficacy of the TMPRSS2 Inhibitor Camostat Mesilate in Patients Hospitalized with Covid-19-A Double-Blind Randomized Controlled Trial. EClinicalMedicine 35, 100849. doi:10.1016/j.eclinm.2021.100849
Halaby, R., Popma, C. J., Cohen, A., Chi, G., Zacarkim, M. R., Romero, G., et al. (2015). D-dimer Elevation and Adverse Outcomes. J. Thromb. Thrombolysis 39, 55–59. doi:10.1007/s11239-014-1101-6
Han, H., Ma, Q., Li, C., Liu, R., Zhao, L., Wang, W., et al. (2020). Profiling Serum Cytokines in COVID-19 Patients Reveals IL-6 and IL-10 Are Disease Severity Predictors. Emerg. Microbes Infect. 9, 1123–1130. doi:10.1080/22221751.2020.1770129
Han, Y., Guo, D., Chen, Y., Chen, Y., Tan, Z.-R., and Zhou, H.-H. (2009). Effect of Silymarin on the Pharmacokinetics of Losartan and its Active Metabolite E-3174 in Healthy Chinese Volunteers. Eur. J. Clin. Pharmacol. 65, 585–591. doi:10.1007/s00228-009-0624-9
He, X.-J., Zhao, L.-M., Qiu, F., Sun, Y.-X., and Li-Ling, J. (2009). Influence of ABCB1 Gene Polymorphisms on the Pharmacokinetics of Azithromycin Among Healthy Chinese Han Ethnic Subjects. Pharmacol. Rep. 61, 843–850. doi:10.1016/s1734-1140(09)70140-9
Ho, P., Zheng, J.-Q., Wu, C.-C., Hou, Y.-C., Liu, W.-C., Lu, C.-L., et al. (2021). Perspective Adjunctive Therapies for COVID-19: Beyond Antiviral Therapy. Int. J. Med. Sci. 18, 314–324. doi:10.7150/ijms.51935
Hodgson, S. H., Mansatta, K., Mallett, G., Harris, V., Emary, K. R. W., and Pollard, A. J. (2021). What Defines an Efficacious COVID-19 Vaccine? A Review of the Challenges Assessing the Clinical Efficacy of Vaccines against SARS-CoV-2. Lancet Infect. Dis. 21, e26–e35. doi:10.1016/S1473-3099(20)30773-8
Hoffman, S. L., Rogers, W. O., Carucci, D. J., and Venter, J. C. (1998). From Genomics to Vaccines: Malaria as a Model System. Nat. Med. 4, 1351–1353. doi:10.1038/3934
Holzinger, E. R., Grady, B., Ritchie, M. D., Ribaudo, H. J., Acosta, E. P., Morse, G. D., et al. (2012). Genome-wide Association Study of Plasma Efavirenz Pharmacokinetics in AIDS Clinical Trials Group Protocols Implicates Several CYP2B6 Variants. Pharmacogenet. Genomics 22, 858–867. doi:10.1097/FPC.0b013e32835a450b
Horby, P., Lim, W. S., Emberson, J. R., Mafham, M., Bell, J. L., Linsell, L., et al. (2021). Dexamethasone in Hospitalized Patients with Covid-19. N. Engl. J. Med. 384, 693–704. doi:10.1056/NEJMoa2021436
Hou, Y., Zhao, J., Martin, W., Kallianpur, A., Chung, M. K., Jehi, L., et al. (2020). New Insights into Genetic Susceptibility of COVID-19: an ACE2 and TMPRSS2 Polymorphism Analysis. BMC Med. 18, 216. doi:10.1186/s12916-020-01673-z
Hu, B., Guo, H., Zhou, P., and Shi, Z.-L. (2021a). Characteristics of SARS-CoV-2 and COVID-19. Nat. Rev. Microbiol. 19, 141–154. doi:10.1038/s41579-020-00459-7
Hu, K., Guan, W.-J., Bi, Y., Zhang, W., Li, L., Zhang, B., et al. (2021b). Efficacy and Safety of Lianhuaqingwen Capsules, a Repurposed Chinese Herb, in Patients with Coronavirus Disease 2019: A Multicenter, Prospective, Randomized Controlled Trial. Phytomedicine 85, 153242. doi:10.1016/j.phymed.2020.153242
Huang, C., Wang, Y., Li, X., Ren, L., Zhao, J., Hu, Y., et al. (2020). Clinical Features of Patients Infected with 2019 Novel Coronavirus in Wuhan, China. Lancet 395, 497–506. doi:10.1016/S0140-6736(20)30183-5
Huang, H., Sikora, M. J., Islam, S., Chowdhury, R. R., Chien, Y.-H., Scriba, T. J., et al. (2019). Select Sequencing of Clonally Expanded CD8(+) T Cells Reveals Limits to Clonal Expansion. Proc. Natl. Acad. Sci. U. S. A. 116, 8995–9001. doi:10.1073/pnas.1902649116
Huang, S.-T., Chen, Y., Chang, W.-C., Chen, H.-F., Lai, H.-C., Lin, Y.-C., et al. (2021). Scutellaria Barbata D. Don Inhibits the Main Proteases (M(pro) and TMPRSS2) of Severe Acute Respiratory Syndrome Coronavirus 2 (SARS-CoV-2) Infection. Viruses 13. doi:10.3390/v13050826
Islam, M. N., Hossain, K. S., Sarker, P. P., Ferdous, J., Hannan, M. A., Rahman, M. M., et al. (2021). Revisiting Pharmacological Potentials of Nigella Sativa Seed: A Promising Option for COVID-19 Prevention and Cure. Phytother. Res. 35, 1329–1344. doi:10.1002/ptr.6895
Iwamura, A., Fukami, T., Hosomi, H., Nakajima, M., and Yokoi, T. (2011). CYP2C9-mediated Metabolic Activation of Losartan Detected by a Highly Sensitive Cell-Based Screening Assay. Drug Metab. Dispos. 39, 838–846. doi:10.1124/dmd.110.037259
Jafrin, S., Naznin, N. E., Reza, M. S., Aziz, M. A., and Islam, M. S. (2021). Risk of Stroke in CYP2C19 LoF Polymorphism Carrier Coronary Artery Disease Patients Undergoing Clopidogrel Therapy: An Ethnicity-Based Updated Meta-Analysis. Eur. J. Intern. Med. 90, 49–65. doi:10.1016/j.ejim.2021.05.022
Jantararoungtong, T., Tempark, T., Koomdee, N., Medhasi, S., and Sukasem, C. (2021a). Genotyping HLA Alleles to Predict the Development of Severe Cutaneous Adverse Drug Reactions (SCARs): State-Of-The-Art. Expert Opin. Drug Metab. Toxicol., 1–16. doi:10.1080/17425255.2021.1946514
Jantararoungtong, T., Wiwattanakul, S., Tiyasirichokchai, R., Prommas, S., Sukprasong, R., Koomdee, N., et al. (2021b). TPMT*3C as a Predictor of 6-Mercaptopurine-Induced Myelotoxicity in Thai Children with Acute Lymphoblastic Leukemia. J. Pers. Med. 11. doi:10.3390/jpm11080783
Jaruchotikamol, A., Jarukamjorn, K., Sirisangtrakul, W., Sakuma, T., Kawasaki, Y., and Nemoto, N. (2007). Strong Synergistic Induction of CYP1A1 Expression by Andrographolide Plus Typical CYP1A Inducers in Mouse Hepatocytes. Toxicol. Appl. Pharmacol. 224, 156–162. doi:10.1016/j.taap.2007.07.008
Jaruthamsophon, K., Tipmanee, V., Sangiemchoey, A., Sukasem, C., and Limprasert, P. (2017). HLA-B*15:21 and Carbamazepine-Induced Stevens-Johnson Syndrome: Pooled-Data and In Silico Analysis. Sci. Rep. 7, 45553. doi:10.1038/srep45553
Jayk Bernal, A., Gomes da Silva, M. M., Musungaie, D. B., Kovalchuk, E., Gonzalez, A., Delos Reyes, V., et al. (2021). Molnupiravir for Oral Treatment of Covid-19 in Nonhospitalized Patients. N. Engl. J. Med. doi:10.1056/NEJMoa2116044
Jeon, S., Ko, M., Lee, J., Choi, I., Byun, S. Y., Park, S., et al. (2020). Identification of Antiviral Drug Candidates against SARS-CoV-2 from FDA-Approved Drugs. Antimicrob. Agents Chemother. 64. doi:10.1128/AAC.00819-20
Jiang, F., Deng, L., Zhang, L., Cai, Y., Cheung, C. W., and Xia, Z. (2020). Review of the Clinical Characteristics of Coronavirus Disease 2019 (COVID-19). J. Gen. Intern. Med., 1–5. doi:10.1007/s11606-020-05762-w
Jiménez Morales, A., Maldonado-Montoro, M., Martínez de la Plata, J. E., Pérez Ramírez, C., Daddaoua, A., Alarcón Payer, C., et al. (2019). FCGR2A/FCGR3A Gene Polymorphisms and Clinical Variables as Predictors of Response to Tocilizumab and Rituximab in Patients with Rheumatoid Arthritis. J. Clin. Pharmacol. 59, 517–531. doi:10.1002/jcph.1341
Jin, Z., Du, X., Xu, Y., Deng, Y., Liu, M., Zhao, Y., et al. (2020). Structure of M(pro) from SARS-CoV-2 and Discovery of its Inhibitors. Nature 582, 289–293. doi:10.1038/s41586-020-2223-y
Johnson, J. A., Caudle, K. E., Gong, L., Whirl-Carrillo, M., Stein, C. M., Scott, S. A., et al. (2017). Clinical Pharmacogenetics Implementation Consortium (CPIC) Guideline for Pharmacogenetics-Guided Warfarin Dosing: 2017 Update. Clin. Pharmacol. Ther. 102, 397–404. doi:10.1002/cpt.668
Jorgensen, A. L., FitzGerald, R. J., Oyee, J., Pirmohamed, M., and Williamson, P. R. (2012). Influence of CYP2C9 and VKORC1 on Patient Response to Warfarin: a Systematic Review and Meta-Analysis. PLoS One 7, e44064. doi:10.1371/journal.pone.0044064
Joshi, R. S., Jagdale, S. S., Bansode, S. B., Shankar, S. S., Tellis, M. B., Pandya, V. K., et al. (2021). Discovery of Potential Multi-Target-Directed Ligands by Targeting Host-specific SARS-CoV-2 Structurally Conserved Main Protease. J. Biomol. Struct. Dyn. 39, 3099–3114. doi:10.1080/07391102.2020.1760137
Kabinger, F., Stiller, C., Schmitzová, J., Dienemann, C., Kokic, G., Hillen, H. S., et al. (2021). Mechanism of Molnupiravir-Induced SARS-CoV-2 Mutagenesis. Nat. Struct. Mol. Biol. 28, 740–746. doi:10.1038/s41594-021-00651-0
Kandeel, M., Abdelrahman, A. H. M., Oh-Hashi, K., Ibrahim, A., Venugopala, K. N., Morsy, M. A., et al. (2021). Repurposing of FDA-Approved Antivirals, Antibiotics, Anthelmintics, Antioxidants, and Cell Protectives against SARS-CoV-2 Papain-like Protease. J. Biomol. Struct. Dyn. 39, 5129–5136. doi:10.1080/07391102.2020.1784291
Kandwal, S., and Fayne, D. (2020). Repurposing Drugs for Treatment of SARS-CoV-2 Infection: Computational Design Insights into Mechanisms of Action. J. Biomol. Struct. Dyn., 1–15. doi:10.1080/07391102.2020.1825232
Kanuri, S. H., and Kreutz, R. P. (2019). Pharmacogenomics of Novel Direct Oral Anticoagulants: Newly Identified Genes and Genetic Variants. J. Pers. Med. 9. doi:10.3390/jpm9010007
Kar, P., Kumar, V., Vellingiri, B., Sen, A., Jaishee, N., Anandraj, A., et al. (2020). Anisotine and Amarogentin as Promising Inhibitory Candidates against SARS-CoV-2 Proteins: a Computational Investigation. J. Biomol. Struct. Dyn., 1–11. doi:10.1080/07391102.2020.1860133
Khater, S., Kumar, P., Dasgupta, N., Das, G., Ray, S., and Prakash, A. (2021). Combining SARS-CoV-2 Proofreading Exonuclease and RNA-dependent RNA Polymerase Inhibitors as a Strategy to Combat COVID-19: A High-Throughput In Silico Screening. Front. Microbiol. 12, 647693. doi:10.3389/fmicb.2021.647693
Kim, Y. S., Chung, H.-S., Noh, S. G., Lee, B., Chung, H. Y., and Choi, J.-G. (2021). Geraniin Inhibits the Entry of SARS-CoV-2 by Blocking the Interaction between Spike Protein RBD and Human ACE2 Receptor. Int. J. Mol. Sci. 22. doi:10.3390/ijms22168604
Klemm, T., Ebert, G., Calleja, D. J., Allison, C. C., Richardson, L. W., Bernardini, J. P., et al. (2020). Mechanism and Inhibition of the Papain-like Protease, PLpro, of SARS-CoV-2. EMBO J. 39, e106275. doi:10.15252/embj.2020106275
Klok, F. A., Kruip, M. J. H. A., van der Meer, N. J. M., Arbous, M. S., Gommers, D. A. M. P. J., Kant, K. M., et al. (2020). Incidence of Thrombotic Complications in Critically Ill ICU Patients with COVID-19. Thromb. Res. 191, 145–147. doi:10.1016/j.thromres.2020.04.013
Klomp, S. D., Manson, M. L., Guchelaar, H.-J., and Swen, J. J. (2020). Phenoconversion of Cytochrome P450 Metabolism: A Systematic Review. J. Clin. Med. 9. doi:10.3390/jcm9092890
Kodchakorn, K., Poovorawan, Y., Suwannakarn, K., and Kongtawelert, P. (2020). Molecular Modelling Investigation for Drugs and Nutraceuticals against Protease of SARS-CoV-2. J. Mol. Graph. Model. 101, 107717. doi:10.1016/j.jmgm.2020.107717
Koomdee, N., Pratoomwun, J., Jantararoungtong, T., Theeramoke, V., Tassaneeyakul, W., Klaewsongkram, J., et al. (2017). Association of HLA-A and HLA-B Alleles with Lamotrigine-Induced Cutaneous Adverse Drug Reactions in the Thai Population. Front. Pharmacol. 8, 879. doi:10.3389/fphar.2017.00879
Kowalec, K., Wright, G. E. B., Drögemöller, B. I., Aminkeng, F., Bhavsar, A. P., Kingwell, E., et al. (2018). Common Variation Near IRF6 Is Associated with IFN-β-Induced Liver Injury in Multiple Sclerosis. Nat. Genet. 50, 1081–1085. doi:10.1038/s41588-018-0168-y
Kuhn, J. H., Li, W., Choe, H., and Farzan, M. (2004). Angiotensin-converting Enzyme 2: a Functional Receptor for SARS Coronavirus. Cell. Mol. Life Sci. 61, 2738–2743. doi:10.1007/s00018-004-4242-5
Lan, J., Ge, J., Yu, J., Shan, S., Zhou, H., Fan, S., et al. (2020). Structure of the SARS-CoV-2 Spike Receptor-Binding Domain Bound to the ACE2 Receptor. Nature 581, 215–220. doi:10.1038/s41586-020-2180-5
Langton, D. J., Bourke, S. C., Lie, B. A., Reiff, G., Natu, S., Darlay, R., et al. (2021). The Influence of HLA Genotype on the Severity of COVID-19 Infection. HLA 98, 14–22. doi:10.1111/tan.14284
Le Bert, N., Tan, A. T., Kunasegaran, K., Tham, C. Y. L., Hafezi, M., Chia, A., et al. (2020). SARS-CoV-2-specific T Cell Immunity in Cases of COVID-19 and SARS, and Uninfected Controls. Nature 584, 457–462. doi:10.1038/s41586-020-2550-z
Leal, C. M., Leitão, S. G., Sausset, R., Mendonça, S. C., Nascimento, P. H. A., de Araujo R Cheohen, C. F., et al. (2021). Flavonoids from Siparuna Cristata as Potential Inhibitors of SARS-CoV-2 Replication. Rev. Bras. Farmacogn. Orgao da Soc. Bras. Farmacogn., 1–9. doi:10.1007/s43450-021-00162-5
Lee, W. S., Wheatley, A. K., Kent, S. J., and DeKosky, B. J. (2020). Antibody-dependent Enhancement and SARS-CoV-2 Vaccines and Therapies. Nat. Microbiol. 5, 1185–1191. doi:10.1038/s41564-020-00789-5
Lelli, D., Sahebkar, A., Johnston, T. P., and Pedone, C. (2017). Curcumin Use in Pulmonary Diseases: State of the Art and Future Perspectives. Pharmacol. Res. 115, 133–148. doi:10.1016/j.phrs.2016.11.017
Lemaitre, F., Solas, C., Grégoire, M., Lagarce, L., Elens, L., Polard, E., et al. (2020). Potential Drug-Drug Interactions Associated with Drugs Currently Proposed for COVID-19 Treatment in Patients Receiving Other Treatments. Fundam. Clin. Pharmacol. 34, 530–547. doi:10.1111/fcp.12586
Lespine, A., Dupuy, J., Orlowski, S., Nagy, T., Glavinas, H., Krajcsi, P., et al. (2006). Interaction of Ivermectin with Multidrug Resistance Proteins (MRP1, 2 and 3). Chem. Biol. Interact. 159, 169–179. doi:10.1016/j.cbi.2005.11.002
Li, F., Li, W., Farzan, M., and Harrison, S. C. (2005). Structure of SARS Coronavirus Spike Receptor-Binding Domain Complexed with Receptor. Science 309, 1864–1868. doi:10.1126/science.1116480
Li, Q., Yi, D., Lei, X., Zhao, J., Zhang, Y., Cui, X., et al. (2021). Corilagin Inhibits SARS-CoV-2 Replication by Targeting Viral RNA-dependent RNA Polymerase. Acta Pharm. Sin. B 11, 1555–1567. doi:10.1016/j.apsb.2021.02.011
Lim, Y. X., Ng, Y. L., Tam, J. P., and Liu, D. X. (2016). Human Coronaviruses: A Review of Virus-Host Interactions. Dis. (Basel, Switzerland) 4. doi:10.3390/diseases4030026
Littera, R., Campagna, M., Deidda, S., Angioni, G., Cipri, S., Melis, M., et al. (2020). Human Leukocyte Antigen Complex and Other Immunogenetic and Clinical Factors Influence Susceptibility or Protection to SARS-CoV-2 Infection and Severity of the Disease Course. The Sardinian Experience. Front. Immunol. 11, 605688. doi:10.3389/fimmu.2020.605688
Liu, W., Cho, C.-C., Li, S. G., Lalonde, T. J., Yang, K. S., Yu, G., et al. (2021). Drug Repurposing for the SARS-CoV-2 Papain-like Protease. ChemMedChem. doi:10.1002/cmdc.202100455
Liu, Y., Yang, Y., Zhang, C., Huang, F., Wang, F., Yuan, J., et al. (2020). Clinical and Biochemical Indexes from 2019-nCoV Infected Patients Linked to Viral Loads and Lung Injury. Sci. China Life Sci. 63, 364–374. doi:10.1007/s11427-020-1643-8
Lorente, L., Martín, M. M., Franco, A., Barrios, Y., Cáceres, J. J., Solé-Violán, J., et al. (2021). HLA Genetic Polymorphisms and Prognosis of Patients with COVID-19. Med. Intensiva 45, 96–103. doi:10.1016/j.medin.2020.08.004
Lubomirov, R., di Iulio, J., Fayet, A., Colombo, S., Martinez, R., Marzolini, C., et al. (2010). ADME Pharmacogenetics: Investigation of the Pharmacokinetics of the Antiretroviral Agent Lopinavir Coformulated with Ritonavir. Pharmacogenet. Genomics 20, 217–230. doi:10.1097/FPC.0b013e328336eee4
Macías, Y., Gómez Tabales, J., García-Martín, E., and Agúndez, J. A. G. (2020). An Update on the Pharmacogenomics of NSAID Metabolism and the Risk of Gastrointestinal Bleeding. Expert Opin. Drug Metab. Toxicol. 16, 319–332. doi:10.1080/17425255.2020.1744563
Mahdi, M., Mótyán, J. A., Szojka, Z. I., Golda, M., Miczi, M., and Tőzsér, J. (2020). Analysis of the Efficacy of HIV Protease Inhibitors against SARS-CoV-2’s Main Protease. Virol. J. 17, 190. doi:10.1186/s12985-020-01457-0
Maideen, N. M. P. (2020). Prophetic Medicine-Nigella Sativa (Black Cumin Seeds) - Potential Herb for COVID-19? J. Pharmacopuncture 23, 62–70. doi:10.3831/KPI.2020.23.010
Maldonado-Montoro, M., Cañadas-Garre, M., González-Utrilla, A., and Ángel Calleja-Hernández, M. (2018). Influence of IL6R Gene Polymorphisms in the Effectiveness to Treatment with Tocilizumab in Rheumatoid Arthritis. Pharmacogenomics J. 18, 167–172. doi:10.1038/tpj.2016.88
Maldonado-Montoro, M., Cañadas-Garre, M., González-Utrilla, A., Plaza-Plaza, J. C., and Calleja-Hernández, M. Ÿ. (2016). Genetic and Clinical Biomarkers of Tocilizumab Response in Patients with Rheumatoid Arthritis. Pharmacol. Res. 111, 264–271. doi:10.1016/j.phrs.2016.06.016
Manjaly Thomas, Z.-R., Leuppi-Taegtmeyer, A., Jamiolkowski, D., Steveling-Klein, E., Bellutti-Enders, F., Scherer Hofmeier, K., et al. (2020). Emerging Treatments in COVID-19: Adverse Drug Reactions Including Drug Hypersensitivities. J. Allergy Clin. Immunol. 146, 786–789. doi:10.1016/j.jaci.2020.07.008
Manosuthi, W., Sukasem, C., Thongyen, S., Nilkamhang, S., Manosuthi, S., and Sungkanuparph, S. (2014). CYP2B6 18492T->C Polymorphism Compromises Efavirenz Concentration in Coinfected HIV and Tuberculosis Patients Carrying CYP2B6 Haplotype *1/*1. Antimicrob. Agents Chemother. 58, 2268–2273. doi:10.1128/AAC.02384-13
Maria, K., Costanzi, E., Reinshagen, J., Esposito, F., Vangeel, L., Wolf, M., et al. (2021). Identification of Inhibitors of SARS-CoV-2 3CL-Pro Enzymatic Activity Using a Small Molecule In Vitro Repurposing Screen. ACS Pharmacol. Transl Sci. 4, 1096–1110. doi:10.1021/acsptsci.0c00216
Martin, A. M., Nolan, D., James, I., Cameron, P., Keller, J., Moore, C., et al. (2005). Predisposition to Nevirapine Hypersensitivity Associated with HLA-Drb1*0101 and Abrogated by Low CD4 T-Cell Counts. AIDS 19, 97–99. doi:10.1097/00002030-200501030-00014
Martinez, M. A. (2020). Compounds with Therapeutic Potential against Novel Respiratory 2019 Coronavirus. Antimicrob. Agents Chemother. 64. doi:10.1128/AAC.00399-20
Mathpal, S., Sharma, P., Joshi, T., Joshi, T., Pande, V., and Chandra, S. (2021). Screening of Potential Bio-Molecules from Moringa Olifera against SARS-CoV-2 Main Protease Using Computational Approaches. J. Biomol. Struct. Dyn., 1–16. doi:10.1080/07391102.2021.1936183
Mattos-Silva, P., Felix, N. S., Silva, P. L., Robba, C., Battaglini, D., Pelosi, P., et al. (2020). Pros and Cons of Corticosteroid Therapy for COVID-19 Patients. Respir. Physiol. Neurobiol. 280, 103492. doi:10.1016/j.resp.2020.103492
McCreary, E. K., and Pogue, J. M. (2020). Coronavirus Disease 2019 Treatment: A Review of Early and Emerging Options. Open Forum Infect. Dis. 7. doi:10.1093/ofid/ofaa105
McDonagh, E. M., Lau, J. L., Alvarellos, M. L., Altman, R. B., and Klein, T. E. (2015). PharmGKB Summary: Efavirenz Pathway, Pharmacokinetics. Pharmacogenet. Genomics 25, 363–376. doi:10.1097/FPC.0000000000000145
Medhasi, S., Pasomsub, E., Vanwong, N., Ngamsamut, N., Puangpetch, A., Chamnanphon, M., et al. (2016). Clinically Relevant Genetic Variants of Drug-Metabolizing Enzyme and Transporter Genes Detected in Thai Children and Adolescents with Autism Spectrum Disorder. Neuropsychiatr. Dis. Treat. 12, 843–851. doi:10.2147/NDT.S101580
Melo, J. R. R., Duarte, E. C., Moraes, M. V., Fleck, K., Silvado, A. S. N. E., and Arrais, P. S. D. (2021). Adverse Drug Reactions in Patients with COVID-19 in Brazil: Analysis of Spontaneous Notifications of the Brazilian Pharmacovigilance System. Cad. Saude Publica 37, e00245820. doi:10.1590/0102-311X00245820
Moltó, J., Valle, M., Miranda, C., Cedeño, S., Negredo, E., Barbanoj, M. J., et al. (2011). Herb-drug Interaction between Echinacea Purpurea and Darunavir-Ritonavir in HIV-Infected Patients. Antimicrob. Agents Chemother. 55, 326–330. doi:10.1128/AAC.01082-10
Moltó, J., Xinarianos, G., Miranda, C., Pushpakom, S., Cedeño, S., Clotet, B., et al. (2013). Simultaneous Pharmacogenetics-Based Population Pharmacokinetic Analysis of Darunavir and Ritonavir in HIV-Infected Patients. Clin. Pharmacokinet. 52, 543–553. doi:10.1007/s40262-013-0057-6
Mounce, B. C., Cesaro, T., Carrau, L., Vallet, T., and Vignuzzi, M. (2017). Curcumin Inhibits Zika and Chikungunya Virus Infection by Inhibiting Cell Binding. Antivir. Res 142, 148–157. doi:10.1016/j.antiviral.2017.03.014
Mozzini, C., and Girelli, D. (2020). The Role of Neutrophil Extracellular Traps in Covid-19: Only an Hypothesis or a Potential New Field of Research? Thromb. Res. 191, 26–27. doi:10.1016/j.thromres.2020.04.031
Mukonzo, J. K., Röshammar, D., Waako, P., Andersson, M., Fukasawa, T., Milani, L., et al. (2009). A Novel Polymorphism in ABCB1 Gene, CYP2B6*6 and Sex Predict Single-Dose Efavirenz Population Pharmacokinetics in Ugandans. Br. J. Clin. Pharmacol. 68, 690–699. doi:10.1111/j.1365-2125.2009.03516.x
Naemi, F. M. A., Al-Adwani, S., Al-Khatabi, H., and Al-Nazawi, A. (2021). Association between the HLA Genotype and the Severity of COVID-19 Infection Among South Asians. J. Med. Virol. 93, 4430–4437. doi:10.1002/jmv.27003
Naidoo, D., Roy, A., Kar, P., Mutanda, T., and Anandraj, A. (2021). Cyanobacterial Metabolites as Promising Drug Leads against the M(pro) and PL(pro) of SARS-CoV-2: an In Silico Analysis. J. Biomol. Struct. Dyn. 39, 6218–6230. doi:10.1080/07391102.2020.1794972
Narkhede, R. R., Pise, A. V., Cheke, R. S., and Shinde, S. D. (2020). Recognition of Natural Products as Potential Inhibitors of COVID-19 Main Protease (Mpro): In-Silico Evidences. Nat. Prod. Bioprospect. 10, 297–306. doi:10.1007/s13659-020-00253-1
Nastri, B. M., Zannella, C., Folliero, V., Rinaldi, L., Restivo, L., Stelitano, D., et al. (2020). Role of Highly Active Antiretroviral Therapy (HAART) for the COVID-19 Treatment. Eur. Rev. Med. Pharmacol. Sci. 24, 11982–11984. doi:10.26355/eurrev_202011_23861
Nathan, R., Shawa, I., De La Torre, I., Pustizzi, J. M., Haustrup, N., Patel, D. R., et al. (2021). A Narrative Review of the Clinical Practicalities of Bamlanivimab and Etesevimab Antibody Therapies for SARS-CoV-2. Infect. Dis. Ther. 10, 1933–1947. doi:10.1007/s40121-021-00515-6
Nawrot-Hadzik, I., Zmudzinski, M., Matkowski, A., Preissner, R., Kęsik-Brodacka, M., Hadzik, J., et al. (2021). Reynoutria Rhizomes as a Natural Source of SARS-CoV-2 Mpro Inhibitors-Molecular Docking and In Vitro Study. Pharmaceuticals (Basel) 14. doi:10.3390/ph14080742
Naydenova, K., Muir, K. W., Wu, L.-F., Zhang, Z., Coscia, F., Peet, M. J., et al. (2021). Structure of the SARS-CoV-2 RNA-dependent RNA Polymerase in the Presence of Favipiravir-RTP. Proc. Natl. Acad. Sci. U. S. A. 118. doi:10.1073/pnas.2021946118
Ng, S. L., Khaw, K.-Y., Ong, Y. S., Goh, H. P., Kifli, N., Teh, S. P., et al. (2021). Licorice: A Potential Herb in Overcoming SARS-CoV-2 Infections. J. Evidence-based Integr. Med. 26, 2515690X21996662. doi:10.1177/2515690X21996662
Noris, M., Benigni, A., and Remuzzi, G. (2020). The Case of Complement Activation in COVID-19 Multiorgan Impact. Kidney Int. 98, 314–322. doi:10.1016/j.kint.2020.05.013
Novelli, A., Andreani, M., Biancolella, M., Liberatoscioli, L., Passarelli, C., Colona, V. L., et al. (2020). HLA Allele Frequencies and Susceptibility to COVID-19 in a Group of 99 Italian Patients. HLA 96, 610–614. doi:10.1111/tan.14047
Nutho, B., Mahalapbutr, P., Hengphasatporn, K., Pattaranggoon, N. C., Simanon, N., Shigeta, Y., et al. (2020). Why Are Lopinavir and Ritonavir Effective against the Newly Emerged Coronavirus 2019? Atomistic Insights into the Inhibitory Mechanisms. Biochemistry 59, 1769–1779. doi:10.1021/acs.biochem.0c00160
Omersel, J., and Karas Kuželički, N. (2020). Vaccinomics and Adversomics in the Era of Precision Medicine: A Review Based on HBV, MMR, HPV, and COVID-19 Vaccines. J. Clin. Med. 9. doi:10.3390/jcm9113561
Pan, Y., Abd-Rashid, B. A., Ismail, Z., Ismail, R., Mak, J. W., Pook, P. C. K., et al. (2011). In Vitro determination of the Effect of Andrographis Paniculata Extracts and Andrographolide on Human Hepatic Cytochrome P450 Activities. J. Nat. Med. 65, 440–447. doi:10.1007/s11418-011-0516-z
Panikar, S., Shoba, G., Arun, M., Sahayarayan, J. J., Usha Raja Nanthini, A., Chinnathambi, A., et al. (2021). Essential Oils as an Effective Alternative for the Treatment of COVID-19: Molecular Interaction Analysis of Protease (M(pro)) with Pharmacokinetics and Toxicological Properties. J. Infect. Public Health 14, 601–610. doi:10.1016/j.jiph.2020.12.037
Pathak, N., Chen, Y.-T., Hsu, Y.-C., Hsu, N.-Y., Kuo, C.-J., Tsai, H. P., et al. (2021). Uncovering Flexible Active Site Conformations of SARS-CoV-2 3CL Proteases through Protease Pharmacophore Clusters and COVID-19 Drug Repurposing. ACS Nano 15, 857–872. doi:10.1021/acsnano.0c07383
Peele, K. A., Potla Durthi, C., Srihansa, T., Krupanidhi, S., Ayyagari, V. S., Babu, D. J., et al. (2020). Molecular Docking and Dynamic Simulations for Antiviral Compounds against SARS-CoV-2: A Computational Study. Inform. Med. unlocked 19, 100345. doi:10.1016/j.imu.2020.100345
Pekthong, D., Blanchard, N., Abadie, C., Bonet, A., Heyd, B., Mantion, G., et al. (2009). Effects of Andrographis Paniculata Extract and Andrographolide on Hepatic Cytochrome P450 mRNA Expression and Monooxygenase Activities after In Vivo Administration to Rats and In Vitro in Rat and Human Hepatocyte Cultures. Chem. Biol. Interact. 179, 247–255. doi:10.1016/j.cbi.2008.10.054
Pekthong, D., Martin, H., Abadie, C., Bonet, A., Heyd, B., Mantion, G., et al. (2008). Differential Inhibition of Rat and Human Hepatic Cytochrome P450 by Andrographis Paniculata Extract and Andrographolide. J. Ethnopharmacol. 115, 432–440. doi:10.1016/j.jep.2007.10.013
Penzak, S. R., Robertson, S. M., Hunt, J. D., Chairez, C., Malati, C. Y., Alfaro, R. M., et al. (2010). Echinacea Purpurea Significantly Induces Cytochrome P450 3A Activity but Does Not Alter Lopinavir-Ritonavir Exposure in Healthy Subjects. Pharmacotherapy 30, 797–805. doi:10.1592/phco.30.8.797
Pineda-Tenor, D., García-Álvarez, M., Jiménez-Sousa, M. A., Vázquez-Morón, S., and Resino, S. (2015). Relationship between ITPA Polymorphisms and Hemolytic Anemia in HCV-Infected Patients after Ribavirin-Based Therapy: a Meta-Analysis. J. Transl. Med. 13, 320. doi:10.1186/s12967-015-0682-y
Poland, G. A., Ovsyannikova, I. G., Jacobson, R. M., and Smith, D. I. (2007). Heterogeneity in Vaccine Immune Response: the Role of Immunogenetics and the Emerging Field of Vaccinomics. Clin. Pharmacol. Ther. 82, 653–664. doi:10.1038/sj.clpt.6100415
Poland, G. A., Ovsyannikova, I. G., and Kennedy, R. B. (2018). Personalized Vaccinology: A Review. Vaccine 36, 5350–5357. doi:10.1016/j.vaccine.2017.07.062
Poland, G. A., Ovsyannikova, I. G., and Kennedy, R. B. (2021). Pharmacogenomics and Vaccine Development. Clin. Pharmacol. Ther. 110, 546–548. doi:10.1002/cpt.2288
Ponti, G., Maccaferri, M., Ruini, C., Tomasi, A., and Ozben, T. (2020a). Biomarkers Associated with COVID-19 Disease Progression. Crit. Rev. Clin. Lab. Sci. 57, 389–399. doi:10.1080/10408363.2020.1770685
Ponti, G., Ruini, C., and Tomasi, A. (2020b). Homocysteine as a Potential Predictor of Cardiovascular Risk in Patients with COVID-19. Med. Hypotheses 143, 109859. doi:10.1016/j.mehy.2020.109859
Poustforoosh, A., Hashemipour, H., Tüzün, B., Pardakhty, A., Mehrabani, M., and Nematollahi, M. H. (2021). Evaluation of Potential Anti-RNA-dependent RNA Polymerase (RdRP) Drugs against the Newly Emerged Model of COVID-19 RdRP Using Computational Methods. Biophys. Chem. 272, 106564. doi:10.1016/j.bpc.2021.106564
Praditya, D., Kirchhoff, L., Brüning, J., Rachmawati, H., Steinmann, J., and Steinmann, E. (2019). Anti-infective Properties of the Golden Spice Curcumin. Front. Microbiol. 10, 912. doi:10.3389/fmicb.2019.00912
Prasad, S., and Tyagi, A. K. (2015). Curcumin and its Analogues: a Potential Natural Compound against HIV Infection and AIDS. Food Funct. 6, 3412–3419. doi:10.1039/c5fo00485c
Puangpetch, A., Koomdee, N., Chamnanphol, M., Jantararoungtong, T., Santon, S., Prommas, S., et al. (2014). HLA-B Allele and Haplotype Diversity Among Thai Patients Identified by PCR-SSOP: Evidence for High Risk of Drug-Induced Hypersensitivity. Front. Genet. 5, 478. doi:10.3389/fgene.2014.00478
Puangpetch, A., Suwannarat, P., Chamnanphol, M., Koomdee, N., Ngamsamut, N., Limsila, P., et al. (2015). Significant Association of HLA-B Alleles and Genotypes in Thai Children with Autism Spectrum Disorders: A Case-Control Study. Dis. Markers 2015, 724935. doi:10.1155/2015/724935
Puttaswamy, H., Gowtham, H. G., Ojha, M. D., Yadav, A., Choudhir, G., Raguraman, V., et al. (2020). In Silico studies Evidenced the Role of Structurally Diverse Plant Secondary Metabolites in Reducing SARS-CoV-2 Pathogenesis. Sci. Rep. 10, 20584. doi:10.1038/s41598-020-77602-0
Quimque, M. T. J., Notarte, K. I. R., Fernandez, R. A. T., Mendoza, M. A. O., Liman, R. A. D., Lim, J. A. K., et al. (2021). Virtual Screening-Driven Drug Discovery of SARS-CoV2 Enzyme Inhibitors Targeting Viral Attachment, Replication, post-translational Modification and Host Immunity Evasion Infection Mechanisms. J. Biomol. Struct. Dyn. 39, 4316–4333. doi:10.1080/07391102.2020.1776639
Rahimi, R., and Abdollahi, M. (2012). An Update on the Ability of St. John’s Wort to Affect the Metabolism of Other Drugs. Expert Opin. Drug Metab. Toxicol. 8, 691–708. doi:10.1517/17425255.2012.680886
Rahman, N., Basharat, Z., Yousuf, M., Castaldo, G., Rastrelli, L., and Khan, H. (2020). Virtual Screening of Natural Products against Type II Transmembrane Serine Protease (TMPRSS2), the Priming Agent of Coronavirus 2 (SARS-CoV-2). Molecules 25. doi:10.3390/molecules25102271
Rainsford, K. D., Parke, A. L., Clifford-Rashotte, M., and Kean, W. F. (2015). Therapy and Pharmacological Properties of Hydroxychloroquine and Chloroquine in Treatment of Systemic Lupus Erythematosus, Rheumatoid Arthritis and Related Diseases. Inflammopharmacology 23, 231–269. doi:10.1007/s10787-015-0239-y
Rakhmanina, N. Y., Neely, M. N., Van Schaik, R. H. N., Gordish-Dressman, H. A., Williams, K. D., Soldin, S. J., et al. (2011). CYP3A5, ABCB1, and SLCO1B1 Polymorphisms and Pharmacokinetics and Virologic Outcome of Lopinavir/ritonavir in HIV-Infected Children. Ther. Drug Monit. 33, 417–424. doi:10.1097/FTD.0b013e318225384f
Ramakrishnan, S., Nicolau, D. V. J., Langford, B., Mahdi, M., Jeffers, H., Mwasuku, C., et al. (2021). Inhaled Budesonide in the Treatment of Early COVID-19 (STOIC): a Phase 2, Open-Label, Randomised Controlled Trial. Lancet Respir. Med. 9, 763–772. doi:10.1016/S2213-2600(21)00160-0
Ramírez, E., Urroz, M., Rodríguez, A., González-Muñoz, M., Martín-Vega, A., Villán, Y., et al. (2020). Incidence of Suspected Serious Adverse Drug Reactions in Corona Virus Disease-19 Patients Detected by a Pharmacovigilance Program by Laboratory Signals in a Tertiary Hospital in Spain: Cautionary Data. Front. Pharmacol. 11, 602841. doi:10.3389/fphar.2020.602841
Ranasinghe, S., Lamothe, P. A., Soghoian, D. Z., Kazer, S. W., Cole, M. B., Shalek, A. K., et al. (2016). Antiviral CD8(+) T Cells Restricted by Human Leukocyte Antigen Class II Exist during Natural HIV Infection and Exhibit Clonal Expansion. Immunity 45, 917–930. doi:10.1016/j.immuni.2016.09.015
Rezaee, H., Pourkarim, F., Pourtaghi-Anvarian, S., Entezari-Maleki, T., Asvadi-Kermani, T., and Nouri-Vaskeh, M. (2021). Drug-drug Interactions with Candidate Medications Used for COVID-19 Treatment: An Overview. Pharmacol. Res. Perspect. 9, e00705. doi:10.1002/prp2.705
Robb, C. T., Goepp, M., Rossi, A. G., and Yao, C. (2020). Non-steroidal Anti-inflammatory Drugs, Prostaglandins, and COVID-19. Br. J. Pharmacol. 177, 4899–4920. doi:10.1111/bph.15206
Robertson, S. M., Davey, R. T., Voell, J., Formentini, E., Alfaro, R. M., and Penzak, S. R. (2008). Effect of Ginkgo Biloba Extract on Lopinavir, Midazolam and Fexofenadine Pharmacokinetics in Healthy Subjects. Curr. Med. Res. Opin. 24, 591–599. doi:10.1185/030079908x260871
Rodríguez-Nóvoa, S., Martín-Carbonero, L., Barreiro, P., González-Pardo, G., Jiménez-Nácher, I., González-Lahoz, J., et al. (2007). Genetic Factors Influencing Atazanavir Plasma Concentrations and the Risk of Severe Hyperbilirubinemia. AIDS 21, 41–46. doi:10.1097/QAD.0b013e328011d7c1
Rombolà, L., Scuteri, D., Marilisa, S., Watanabe, C., Morrone, L. A., Bagetta, G., et al. (2020). Pharmacokinetic Interactions between Herbal Medicines and Drugs: Their Mechanisms and Clinical Relevance. Life (Basel, Switzerland) 10. doi:10.3390/life10070106
Romeo, I., Mesiti, F., Lupia, A., and Alcaro, S. (2021). Current Updates on Naturally Occurring Compounds Recognizing Sars-Cov-2 Druggable Targets. Molecules 26. doi:10.3390/molecules26030632
Rostami, M., and Mansouritorghabeh, H. (2020). D-dimer Level in COVID-19 Infection: a Systematic Review. Expert Rev. Hematol. 13, 1265–1275. doi:10.1080/17474086.2020.1831383
Rouf, R., Uddin, S. J., Sarker, D. K., Islam, M. T., Ali, E. S., Shilpi, J. A., et al. (2020). Antiviral Potential of Garlic (Allium Sativum) and its Organosulfur Compounds: A Systematic Update of Pre-clinical and Clinical Data. Trends Food Sci. Technol. 104, 219–234. doi:10.1016/j.tifs.2020.08.006
Runfeng, L., Yunlong, H., Jicheng, H., Weiqi, P., Qinhai, M., Yongxia, S., et al. (2020). Lianhuaqingwen Exerts Anti-viral and Anti-inflammatory Activity against Novel Coronavirus (SARS-CoV-2). Pharmacol. Res. 156, 104761. doi:10.1016/j.phrs.2020.104761
Rut, W., Lv, Z., Zmudzinski, M., Patchett, S., Nayak, D., Snipas, S. J., et al. (2020). Activity Profiling and crystal Structures of Inhibitor-Bound SARS-CoV-2 Papain-like Protease: A Framework for Anti-COVID-19 Drug Design. Sci. Adv. 6. doi:10.1126/sciadv.abd4596
Saeed, M., Saeed, A., Alam, M. J., and Alreshidi, M. (2021). Receptor-Based Pharmacophore Modeling in the Search for Natural Products for COVID-19 M(pro). Molecules 26. doi:10.3390/molecules26061549
Salama, C., Han, J., Yau, L., Reiss, W. G., Kramer, B., Neidhart, J. D., et al. (2021). Tocilizumab in Patients Hospitalized with Covid-19 Pneumonia. N. Engl. J. Med. 384, 20–30. doi:10.1056/NEJMoa2030340
Sanders, J. M., Monogue, M. L., Jodlowski, T. Z., and Cutrell, J. B. (2020). Pharmacologic Treatments for Coronavirus Disease 2019 (COVID-19): A Review. JAMA - J. Am. Med. Assoc. 323, 1824–1836. doi:10.1001/jama.2020.6019
Satapornpong, P., Jinda, P., Jantararoungtong, T., Koomdee, N., Chaichan, C., Pratoomwun, J., et al. (2020). Genetic Diversity of HLA Class I and Class II Alleles in Thai Populations: Contribution to Genotype-Guided Therapeutics. Front. Pharmacol. 11, 78. doi:10.3389/fphar.2020.00078
Scherrmann, J. M. (2020). Intracellular ABCB1 as a Possible Mechanism to Explain the Synergistic Effect of Hydroxychloroquine-Azithromycin Combination in COVID-19 Therapy. AAPS J. 22, 86. doi:10.1208/s12248-020-00465-w
Schrezenmeier, E., and Dörner, T. (2020). Mechanisms of Action of Hydroxychloroquine and Chloroquine: Implications for Rheumatology. Nat. Rev. Rheumatol. 16, 155–166. doi:10.1038/s41584-020-0372-x
Schultz, N. H., Sørvoll, I. H., Michelsen, A. E., Munthe, L. A., Lund-Johansen, F., Ahlen, M. T., et al. (2021). Thrombosis and Thrombocytopenia after ChAdOx1 nCoV-19 Vaccination. N. Engl. J. Med. 384, 2124–2130. doi:10.1056/NEJMoa2104882
Scott, S. A., Sangkuhl, K., Stein, C. M., Hulot, J. S., Mega, J. L., Roden, D. M., et al. (2013). Clinical Pharmacogenetics Implementation Consortium Guidelines for CYP2C19 Genotype and Clopidogrel Therapy: 2013 Update. Clin. Pharmacol. Ther. 94, 317–323. doi:10.1038/clpt.2013.105
Scully, M., Singh, D., Lown, R., Poles, A., Solomon, T., Levi, M., et al. (2021). Pathologic Antibodies to Platelet Factor 4 after ChAdOx1 nCoV-19 Vaccination. N. Engl. J. Med. 384, 2202–2211. doi:10.1056/NEJMoa2105385
Sen, D., Bhaumik, S., Debnath, P., and Debnath, S. (2021). Potentiality of Moringa Oleifera against SARS-CoV-2: Identified by a Rational Computer Aided Drug Design Method. J. Biomol. Struct. Dyn., 1–18. doi:10.1080/07391102.2021.1898475
Sensorn, I., Sirachainan, E., Chamnanphon, M., Pasomsub, E., Trachu, N., Supavilai, P., et al. (2013). Association of CYP3A4/5, ABCB1 and ABCC2 Polymorphisms and Clinical Outcomes of Thai Breast Cancer Patients Treated with Tamoxifen. Pharmgenomics. Pers. Med. 6, 93–98. doi:10.2147/PGPM.S44006
Sensorn, I., Sukasem, C., Sirachainan, E., Chamnanphon, M., Pasomsub, E., Trachu, N., et al. (2016). ABCB1 and ABCC2 and the Risk of Distant Metastasis in Thai Breast Cancer Patients Treated with Tamoxifen. Onco. Targets Ther. 9, 2121–2129. doi:10.2147/OTT.S100905
Sheahan, T. P., Sims, A. C., Graham, R. L., Menachery, V. D., Gralinski, L. E., Case, J. B., et al. (2017). Broad-spectrum Antiviral GS-5734 Inhibits Both Epidemic and Zoonotic Coronaviruses. Sci. Transl. Med. 9. doi:10.1126/scitranslmed.aal3653
Shi, D., Yang, J., Yang, D., LeCluyse, E. L., Black, C., You, L., et al. (2006). Anti-influenza Prodrug Oseltamivir Is Activated by Carboxylesterase Human Carboxylesterase 1, and the Activation Is Inhibited by Antiplatelet Agent Clopidogrel. J. Pharmacol. Exp. Ther. 319, 1477–1484. doi:10.1124/jpet.106.111807
Shi, R., Shan, C., Duan, X., Chen, Z., Liu, P., Song, J., et al. (2020a). A Human Neutralizing Antibody Targets the Receptor-Binding Site of SARS-CoV-2. Nature 584, 120–124. doi:10.1038/s41586-020-2381-y
Shi, T.-H., Huang, Y.-L., Chen, C.-C., Pi, W.-C., Hsu, Y.-L., Lo, L.-C., et al. (2020b). Andrographolide and its Fluorescent Derivative Inhibit the Main Proteases of 2019-nCoV and SARS-CoV through Covalent Linkage. Biochem. Biophys. Res. Commun. 533, 467–473. doi:10.1016/j.bbrc.2020.08.086
Shin, H.-B., Jung, E. H., Kang, P., Lim, C. W., Oh, K.-Y., Cho, C.-K., et al. (2020). ABCB1 c.2677G>T/c.3435C>T Diplotype Increases the Early-phase Oral Absorption of Losartan. Arch. Pharm. Res. 43, 1187–1196. doi:10.1007/s12272-020-01294-3
Shippey, E. A., Wagler, V. D., and Collamer, A. N. (2018). Hydroxychloroquine: An Old Drug with New Relevance. Cleve. Clin. J. Med. 85, 459–467. doi:10.3949/ccjm.85a.17034
Shree, P., Mishra, P., Selvaraj, C., Singh, S. K., Chaube, R., Garg, N., et al. (2020). Targeting COVID-19 (SARS-CoV-2) Main Protease through Active Phytochemicals of Ayurvedic Medicinal Plants - Withania Somnifera (Ashwagandha), Tinospora Cordifolia (Giloy) and Ocimum Sanctum (Tulsi) - a Molecular Docking Study. J. Biomol. Struct. Dyn., 1–14. doi:10.1080/07391102.2020.1810778
Siepmann, T., Sedghi, A., Simon, E., Winzer, S., Barlinn, J., de With, K., et al. (2021). Increased Risk of Acute Stroke Among Patients with Severe COVID-19: a Multicenter Study and Meta-Analysis. Eur. J. Neurol. 28, 238–247. doi:10.1111/ene.14535
Singh, S. K., Upadhyay, A. K., and Reddy, M. S. (2021). Screening of Potent Drug Inhibitors against SARS-CoV-2 RNA Polymerase: an In Silico Approach. 3 Biotech. 11, 93. doi:10.1007/s13205-020-02610-w
Sivaloganathan, H., Ladikou, E. E., and Chevassut, T. (2020). COVID-19 Mortality in Patients on Anticoagulants and Antiplatelet Agents. Br. J. Haematol. 190, e192–e195. doi:10.1111/bjh.16968
Soiza, R. L., Scicluna, C., and Thomson, E. C. (2021). Efficacy and Safety of COVID-19 Vaccines in Older People. Age Ageing 50, 279–283. doi:10.1093/ageing/afaa274
Somboon, T., Mahalapbutr, P., Sanachai, K., Maitarad, P., Lee, V. S., Hannongbua, S., et al. (2021). Computational Study on Peptidomimetic Inhibitors against SARS-CoV-2 Main Protease. J. Mol. Liq. 322, 114999. doi:10.1016/j.molliq.2020.114999
Song, Q.-Q., Xie, W.-Y., Tang, Y.-J., Zhang, J., and Liu, J. (2017). Genetic Variation in the Glucocorticoid Pathway Involved in Interindividual Differences in the Glucocorticoid Treatment. Pharmacogenomics 18, 293–316. doi:10.2217/pgs-2016-0151
Song, W., Gui, M., Wang, X., and Xiang, Y. (2018). Cryo-EM Structure of the SARS Coronavirus Spike Glycoprotein in Complex with its Host Cell Receptor ACE2. Plos Pathog. 14, e1007236. doi:10.1371/journal.ppat.1007236
Song, Y., Zhang, M., Yin, L., Wang, K., Zhou, Y., Zhou, M., et al. (2020). COVID-19 Treatment: Close to a Cure? A Rapid Review of Pharmacotherapies for the Novel Coronavirus (SARS-CoV-2). Int. J. Antimicrob. Agents 56, 106080. doi:10.1016/j.ijantimicag.2020.106080
Sriram, K., and Insel, P. A. (2020). Risks of ACE Inhibitor and ARB Usage in COVID-19: Evaluating the Evidence. Clin. Pharmacol. Ther. 108, 236–241. doi:10.1002/cpt.1863
Stalin, A., Lin, D., Senthamarai Kannan, B., Feng, Y., Wang, Y., Zhao, W., et al. (2021). An In-Silico Approach to Identify the Potential Hot Spots in SARS-CoV-2 Spike RBD to Block the Interaction with ACE2 Receptor. J. Biomol. Struct. Dyn., 1–16. doi:10.1080/07391102.2021.1897682
Stasi, C., Fallani, S., Voller, F., and Silvestri, C. (2020). Treatment for COVID-19: An Overview. Eur. J. Pharmacol. 889, 173644. doi:10.1016/j.ejphar.2020.173644
Stasiulewicz, A., Maksymiuk, A. W., Nguyen, M. L., Bełza, B., and Sulkowska, J. I. (2021). SARS-CoV-2 Papain-like Protease Potential Inhibitors-In Silico Quantitative Assessment. Int. J. Mol. Sci. 22. doi:10.3390/ijms22083957
Sukardiman, M. S., Ervina, M., Fadhil Pratama, M. R., Poerwono, H., and Siswodihardjo, S. (2020). The Coronavirus Disease 2019 Main Protease Inhibitor from Andrographis Paniculata. J. Adv. Pharm. Technol. Res. 11, 157–162. doi:10.4103/japtr.JAPTR_84_20
Sukasem, C., Atasilp, C., Chansriwong, P., Chamnanphon, M., Puangpetch, A., and Sirachainan, E. (2016a). Development of Pyrosequencing Method for Detection of UGT1A1 Polymorphisms in Thai Colorectal Cancers. J. Clin. Lab. Anal. 30, 84–89. doi:10.1002/jcla.21820
Sukasem, C., Chaichan, C., Nakkrut, T., Satapornpong, P., Jaruthamsophon, K., Jantararoungtong, T., et al. (2018a). Association between HLA-B Alleles and Carbamazepine-Induced Maculopapular Exanthema and Severe Cutaneous Reactions in Thai Patients. J. Immunol. Res. 2018, 2780272. doi:10.1155/2018/2780272
Sukasem, C., Chamnanphon, M., Koomdee, N., Santon, S., Jantararoungtong, T., Prommas, S., et al. (2014a). Pharmacogenetics and Clinical Biomarkers for Subtherapeutic Plasma Efavirenz Concentration in HIV-1 Infected Thai Adults. Drug Metab. Pharmacokinet. 29, 289–295. doi:10.2133/dmpk.dmpk-13-rg-077
Sukasem, C., Cressey, T. R., Prapaithong, P., Tawon, Y., Pasomsub, E., Srichunrusami, C., et al. (2012). Pharmacogenetic Markers of CYP2B6 Associated with Efavirenz Plasma Concentrations in HIV-1 Infected Thai Adults. Br. J. Clin. Pharmacol. 74, 1005–1012. doi:10.1111/j.1365-2125.2012.04288.x
Sukasem, C., Jantararoungtong, T., and Koomdee, N. (2021a). Pharmacogenomics Research and its Clinical Implementation in Thailand: Lessons Learned from the Resource-Limited Settings. Drug Metab. Pharmacokinet. 39, 100399. doi:10.1016/j.dmpk.2021.100399
Sukasem, C., Jantararoungtong, T., Kuntawong, P., Puangpetch, A., Koomdee, N., Satapornpong, P., et al. (2016b). HLA-B (*) 58:01 for Allopurinol-Induced Cutaneous Adverse Drug Reactions: Implication for Clinical Interpretation in Thailand. Front. Pharmacol. 7, 186. doi:10.3389/fphar.2016.00186
Sukasem, C., Katsila, T., Tempark, T., Patrinos, G. P., and Chantratita, W. (2018b). Drug-Induced Stevens-Johnson Syndrome and Toxic Epidermal Necrolysis Call for Optimum Patient Stratification and Theranostics via Pharmacogenomics. Annu. Rev. Genomics Hum. Genet. 19, 329–353. doi:10.1146/annurev-genom-083115-022324
Sukasem, C., Pratoomwun, J., Satapornpong, P., Klaewsongkram, J., Rerkpattanapipat, T., Rerknimitr, P., et al. (2020a). Genetic Association of Co-trimoxazole-induced Severe Cutaneous Adverse Reactions Is Phenotype-specific: HLA Class I Genotypes and Haplotypes. Clin. Pharmacol. Ther. 108, 1078–1089. doi:10.1002/cpt.1915
Sukasem, C., Puangpetch, A., Medhasi, S., and Tassaneeyakul, W. (2014b). Pharmacogenomics of Drug-Induced Hypersensitivity Reactions: Challenges, Opportunities and Clinical Implementation. Asian Pac. J. Allergy Immunol. 32, 111–123.
Sukasem, C., Sririttha, S., Chaichan, C., Nakkrut, T., Satapornpong, P., Jaruthamsophon, K., et al. (2021b). Spectrum of Cutaneous Adverse Reactions to Aromatic Antiepileptic Drugs and Human Leukocyte Antigen Genotypes in Thai Patients and Meta-Analysis. Pharmacogenomics J. doi:10.1038/s41397-021-00247-3
Sukasem, C., Sririttha, S., Tempark, T., Klaewsongkram, J., Rerkpattanapipat, T., Puangpetch, A., et al. (2020b). Genetic and Clinical Risk Factors Associated with Phenytoin-Induced Cutaneous Adverse Drug Reactions in Thai Population. Pharmacoepidemiol. Drug Saf. 29, 565–574. doi:10.1002/pds.4979
Sukasem, C., Tunthong, R., Chamnanphon, M., Santon, S., Jantararoungtong, T., Koomdee, N., et al. (2013). CYP2C19 Polymorphisms in the Thai Population and the Clinical Response to Clopidogrel in Patients with Atherothrombotic-Risk Factors. Pharmgenomics. Pers. Med. 6, 85–91. doi:10.2147/PGPM.S42332
Sukprasong, R., Chuwongwattana, S., Koomdee, N., Jantararoungtong, T., Prommas, S., Jinda, P., et al. (2021). Allele Frequencies of Single Nucleotide Polymorphisms of Clinically Important Drug-Metabolizing Enzymes CYP2C9, CYP2C19, and CYP3A4 in a Thai Population. Sci. Rep. 11, 12343. doi:10.1038/s41598-021-90969-y
Sun, J., Deng, X., Chen, X., Huang, J., Huang, S., Li, Y., et al. (2020). Incidence of Adverse Drug Reactions in COVID-19 Patients in China: An Active Monitoring Study by Hospital Pharmacovigilance System. Clin. Pharmacol. Ther. 108, 791–797. doi:10.1002/cpt.1866
Suwalak, T., Srisawasdi, P., Puangpetch, A., Santon, S., Koomdee, N., Chamnanphon, M., et al. (2015). Polymorphisms of the ApoE (Apolipoprotein E) Gene and Their Influence on Dyslipidemia in HIV-1-Infected Individuals. Jpn. J. Infect. Dis. 68, 5–12. doi:10.7883/yoken.JJID.2013.190
T, M. K., K, R., James, N., V, S., and K, R. (2021). Discovery of Potent Covid-19 Main Protease Inhibitors Using Integrated Drug-Repurposing Strategy. Biotechnol. Appl. Biochem. 68, 712–725. doi:10.1002/bab.2159
Tahir Ul Qamar, M., Alqahtani, S. M., Alamri, M. A., and Chen, L.-L. (2020). Structural Basis of SARS-CoV-2 3CL(pro) and Anti-COVID-19 Drug Discovery from Medicinal Plants. J. Pharm. Anal. 10, 313–319. doi:10.1016/j.jpha.2020.03.009
Takahashi, T., Luzum, J. A., Nicol, M. R., and Jacobson, P. A. (2020). Pharmacogenomics of COVID-19 Therapies. NPJ Genomic Med. 5, 35. doi:10.1038/s41525-020-00143-y
Takeuchi, M., Kobayashi, T., Biss, T., Kamali, F., Vear, S. I., Ho, R. H., et al. (2020). CYP2C9, VKORC1, and CYP4F2 Polymorphisms and Pediatric Warfarin Maintenance Dose: a Systematic Review and Meta-Analysis. Pharmacogenomics J. 20, 306–319. doi:10.1038/s41397-019-0117-x
Tanaka, Y., Kurosaki, M., Nishida, N., Sugiyama, M., Matsuura, K., Sakamoto, N., et al. (2011). Genome-wide Association Study Identified ITPA/DDRGK1 Variants Reflecting Thrombocytopenia in Pegylated Interferon and Ribavirin Therapy for Chronic Hepatitis C. Hum. Mol. Genet. 20, 3507–3516. doi:10.1093/hmg/ddr249
Tao, J., Aristotelidis, R., Zanowick-Marr, A., Chambers, L. C., McDonald, J., Mylonakis, E. E., et al. (2021). Evaluation of the Treatment Efficacy and Safety of Remdesivir for COVID-19: a Meta-Analysis. SN Compr. Clin. Med., 1–12. doi:10.1007/s42399-021-01014-y
Tarkiainen, E. K., Backman, J. T., Neuvonen, M., Neuvonen, P. J., Schwab, M., and Niemi, M. (2012). Carboxylesterase 1 Polymorphism Impairs Oseltamivir Bioactivation in Humans. Clin. Pharmacol. Ther. 92, 68–71. doi:10.1038/clpt.2012.13
Taylor, P. C., Adams, A. C., Hufford, M. M., de la Torre, I., Winthrop, K., and Gottlieb, R. L. (2021). Neutralizing Monoclonal Antibodies for Treatment of COVID-19. Nat. Rev. Immunol. 21, 382–393. doi:10.1038/s41577-021-00542-x
Telbisz, Á., Ambrus, C., Mózner, O., Szabó, E., Várady, G., Bakos, É., et al. (2021). Interactions of Potential Anti-COVID-19 Compounds with Multispecific ABC and OATP Drug Transporters. Pharmaceutics 13. doi:10.3390/pharmaceutics13010081
Teli, D. M., Shah, M. B., and Chhabria, M. T. (2020). In Silico Screening of Natural Compounds as Potential Inhibitors of SARS-CoV-2 Main Protease and Spike RBD: Targets for COVID-19. Front. Mol. Biosci. 7, 599079. doi:10.3389/fmolb.2020.599079
Tempark, T., Satapornpong, P., Rerknimitr, P., Nakkam, N., Saksit, N., Wattanakrai, P., et al. (2017). Dapsone-induced Severe Cutaneous Adverse Drug Reactions Are Strongly Linked with HLA-B*13: 01 Allele in the Thai Population. Pharmacogenet. Genomics 27, 429–437. doi:10.1097/FPC.0000000000000306
Theken, K. N., Lee, C. R., Gong, L., Caudle, K. E., Formea, C. M., Gaedigk, A., et al. (2020). Clinical Pharmacogenetics Implementation Consortium Guideline (CPIC) for CYP2C9 and Nonsteroidal Anti-inflammatory Drugs. Clin. Pharmacol. Ther. 108, 191–200. doi:10.1002/cpt.1830
Thomford, N. E., Awortwe, C., Dzobo, K., Adu, F., Chopera, D., Wonkam, A., et al. (2016). Inhibition of CYP2B6 by Medicinal Plant Extracts: Implication for Use of Efavirenz and Nevirapine-Based Highly Active Anti-retroviral Therapy (HAART) in Resource-Limited Settings. Molecules 21. doi:10.3390/molecules21020211
Tsai, K.-C., Chen, S.-Y., Liang, P.-H., Lu, I.-L., Mahindroo, N., Hsieh, H.-P., et al. (2006). Discovery of a Novel Family of SARS-CoV Protease Inhibitors by Virtual Screening and 3D-QSAR Studies. J. Med. Chem. 49, 3485–3495. doi:10.1021/jm050852f
Umehara, K., Huth, F., Jin, Y., Schiller, H., Aslanis, V., Heimbach, T., et al. (2019). Drug-drug Interaction (DDI) Assessments of Ruxolitinib, a Dual Substrate of CYP3A4 and CYP2C9, Using a Verified Physiologically Based Pharmacokinetic (PBPK) Model to Support Regulatory Submissions. Drug Metab. Pers. Ther. 34. doi:10.1515/dmpt-2018-0042
Varga, Z., Flammer, A. J., Steiger, P., Haberecker, M., Andermatt, R., Zinkernagel, A. S., et al. (2020). Endothelial Cell Infection and Endotheliitis in COVID-19. Lancet (London, England) 395, 1417–1418. doi:10.1016/S0140-6736(20)30937-5
Veeravalli, V., Dash, R. P., Thomas, J. A., Babu, R. J., Madgula, L. M. V., and Srinivas, N. R. (2020). Critical Assessment of Pharmacokinetic Drug-Drug Interaction Potential of Tofacitinib, Baricitinib and Upadacitinib, the Three Approved Janus Kinase Inhibitors for Rheumatoid Arthritis Treatment. Drug Saf. 43, 711–725. doi:10.1007/s40264-020-00938-z
Vincent, M. J., Bergeron, E., Benjannet, S., Erickson, B. R., Rollin, P. E., Ksiazek, T. G., et al. (2005). Chloroquine Is a Potent Inhibitor of SARS Coronavirus Infection and Spread. Virol. J. 2, 69. doi:10.1186/1743-422X-2-69
Vitezica, Z. G., Milpied, B., Lonjou, C., Borot, N., Ledger, T. N., Lefebvre, A., et al. (2008). HLA-DRB1*01 Associated with Cutaneous Hypersensitivity Induced by Nevirapine and Efavirenz. AIDS 22, 540–541. doi:10.1097/QAD.0b013e3282f37812
Vohra, M., Sharma, A. R., Satyamoorthy, K., and Rai, P. S. (2021). Pharmacogenomic Considerations for Repurposing of Dexamethasone as a Potential Drug against SARS-CoV-2 Infection. Per. Med. 18, 389–398. doi:10.2217/pme-2020-0183
Wang, M., Cao, R., Zhang, L., Yang, X., Liu, J., Xu, M., et al. (2020a). Remdesivir and Chloroquine Effectively Inhibit the Recently Emerged Novel Coronavirus (2019-nCoV) In Vitro. Cell Res 30, 269–271. doi:10.1038/s41422-020-0282-0
Wang, Q., Zhang, Y., Wu, L., Niu, S., Song, C., Zhang, Z., et al. (2020b). Structural and Functional Basis of SARS-CoV-2 Entry by Using Human ACE2. Cell 181, 894–904. e9. doi:10.1016/j.cell.2020.03.045
Wang, R., Xiao, H., Guo, R., Li, Y., and Shen, B. (2015). The Role of C5a in Acute Lung Injury Induced by Highly Pathogenic Viral Infections. Emerg. Microbes Infect. 4, e28. doi:10.1038/emi.2015.28
Wang, Y., Chen, B., Li, Y., Zhang, L., Wang, Y., Yang, S., et al. (2021). The Use of Renin-Angiotensin-Aldosterone System (RAAS) Inhibitors Is Associated with a Lower Risk of Mortality in Hypertensive COVID-19 Patients: A Systematic Review and Meta-Analysis. J. Med. Virol. 93, 1370–1377. doi:10.1002/jmv.26625
Who, (2021). Coronavirus Disease (COVID-2019) Situation Reports. Available at: https://www.who.int/emergencies/diseases/novel-coronavirus-2019/situation-reports (Accessed September 10, 2021).
Wichmann, D. (2020). Autopsy Findings and Venous Thromboembolism in Patients with COVID-19. Ann. Intern. Med. 173, 1030. doi:10.7326/L20-120610.7326/M20-2003
Wu, C., Chen, X., Cai, Y., Xia, J., Zhou, X., Xu, S., et al. (2020). Risk Factors Associated with Acute Respiratory Distress Syndrome and Death in Patients with Coronavirus Disease 2019 Pneumonia in Wuhan, China. JAMA Intern. Med. 180, 934–943. doi:10.1001/jamainternmed.2020.0994
Xie, Q., Xiang, Q., Mu, G., Ma, L., Chen, S., Zhou, S., et al. (2018). Effect of ABCB1 Genotypes on the Pharmacokinetics and Clinical Outcomes of New Oral Anticoagulants: A Systematic Review and Meta-Analysis. Curr. Pharm. Des. 24, 3558–3565. doi:10.2174/1381612824666181018153641
Xiu, S., Dick, A., Ju, H., Mirzaie, S., Abdi, F., Cocklin, S., et al. (2020). Inhibitors of SARS-CoV-2 Entry: Current and Future Opportunities. J. Med. Chem. 63, 12256–12274. doi:10.1021/acs.jmedchem.0c00502
Yamasaki, S., Tanimoto, K., Kohno, K., Kadowaki, M., Takase, K., Kondo, S., et al. (2015). UGT1A1 *6 Polymorphism Predicts Outcome in Elderly Patients with Relapsed or Refractory Diffuse Large B-Cell Lymphoma Treated with Carboplatin, Dexamethasone, Etoposide and Irinotecan. Ann. Hematol. 94, 65–69. doi:10.1007/s00277-014-2170-5
Yañez, O., Osorio, M. I., Areche, C., Vasquez-Espinal, A., Bravo, J., Sandoval-Aldana, A., et al. (2021). Theobroma Cacao L. Compounds: Theoretical Study and Molecular Modeling as Inhibitors of Main SARS-CoV-2 Protease. Biomed. Pharmacother. 140, 111764. doi:10.1016/j.biopha.2021.111764
Yang, J., Petitjean, S. J. L., Koehler, M., Zhang, Q., Dumitru, A. C., Chen, W., et al. (2020). Molecular Interaction and Inhibition of SARS-CoV-2 Binding to the ACE2 Receptor. Nat. Commun. 11, 4541. doi:10.1038/s41467-020-18319-6
Yang, K. (2020). What Do We Know about Remdesivir Drug Interactions? Clin. Transl. Sci. doi:10.1111/cts.12815
Yepes-Perez, A. F., Herrera-Calderón, O., Oliveros, C. A., Flórez-Álvarez, L., Zapata-Cardona, M. I., Yepes, L., et al. (2021). The Hydroalcoholic Extract of Uncaria Tomentosa (Cat’s Claw) Inhibits the Infection of Severe Acute Respiratory Syndrome Coronavirus 2 (SARS-CoV-2) In Vitro. Evid. Based. Complement. Alternat. Med. 2021, 6679761. doi:10.1155/2021/6679761
Yepes-Pérez, A. F., Herrera-Calderon, O., Sánchez-Aparicio, J.-E., Tiessler-Sala, L., Maréchal, J.-D., and Cardona-G, , W. (2020). Investigating Potential Inhibitory Effect of Uncaria Tomentosa (Cat’s Claw) against the Main Protease 3CL(pro) of SARS-CoV-2 by Molecular Modeling. Evid. Based. Complement. Alternat. Med. 2020, 4932572. doi:10.1155/2020/4932572
Yin, W., Mao, C., Luan, X., Shen, D.-D., Shen, Q., Su, H., et al. (2020). Structural Basis for Inhibition of the RNA-dependent RNA Polymerase from SARS-CoV-2 by Remdesivir. Science 368, 1499–1504. doi:10.1126/science.abc1560
Yoon, J.-J., Toots, M., Lee, S., Lee, M.-E., Ludeke, B., Luczo, J. M., et al. (2018). Orally Efficacious Broad-Spectrum Ribonucleoside Analog Inhibitor of Influenza and Respiratory Syncytial Viruses. Antimicrob. Agents Chemother. 62. doi:10.1128/AAC.00766-18
Yousefi, H., Mashouri, L., Okpechi, S. C., Alahari, N., and Alahari, S. K. (2021). Repurposing Existing Drugs for the Treatment of COVID-19/sars-CoV-2 Infection: A Review Describing Drug Mechanisms of Action. Biochem. Pharmacol. 183, 114296. doi:10.1016/j.bcp.2020.114296
Zandi, K., Musall, K., Oo, A., Cao, D., Liang, B., Hassandarvish, P., et al. (2021). Baicalein and Baicalin Inhibit SARS-CoV-2 RNA-Dependent-RNA Polymerase. Microorganisms 9. doi:10.3390/microorganisms9050893
Zanone Poma, B., Riva, A., Nasi, M., Cicconi, P., Broggini, V., Lepri, A. C., et al. (2008). Genetic Polymorphisms Differently Influencing the Emergence of Atrophy and Fat Accumulation in HIV-Related Lipodystrophy. AIDS 22, 1769–1778. doi:10.1097/QAD.0b013e32830b3a96
Zeng, Z., Andrew, N. W., Arison, B. H., Luffer-Atlas, D., and Wang, R. W. (1998). Identification of Cytochrome P4503A4 as the Major Enzyme Responsible for the Metabolism of Ivermectin by Human Liver Microsomes. Xenobiotica 28, 313–321. doi:10.1080/004982598239597
Zhang, C., Wu, Z., Li, J.-W., Zhao, H., and Wang, G.-Q. (2020a). Cytokine Release Syndrome in Severe COVID-19: Interleukin-6 Receptor Antagonist Tocilizumab May Be the Key to Reduce Mortality. Int. J. Antimicrob. Agents 55, 105954. doi:10.1016/j.ijantimicag.2020.105954
Zhang, D.-H., Wu, K.-L., Zhang, X., Deng, S.-Q., and Peng, B. (2020b). In Silico screening of Chinese Herbal Medicines with the Potential to Directly Inhibit 2019 Novel Coronavirus. J. Integr. Med. 18, 152–158. doi:10.1016/j.joim.2020.02.005
Zhang, F., Huang, J., Liu, W., Wang, C.-R., Liu, Y.-F., Tu, D.-Z., et al. (2021a). Inhibition of Drug-Metabolizing Enzymes by Qingfei Paidu Decoction: Implication of Herb-Drug Interactions in COVID-19 Pharmacotherapy. Food Chem. Toxicol. Int. J. Publ. Br. Ind. Biol. Res. Assoc. 149, 111998. doi:10.1016/j.fct.2021.111998
Zhang, F., Liu, W., Huang, J., Chen, Q.-L., Wang, D.-D., Zou, L.-W., et al. (2021b). Inhibition of Drug-Metabolizing Enzymes by Jingyin Granules: Implications of Herb-Drug Interactions in Antiviral Therapy. Acta Pharmacol. Sin., 1–10. doi:10.1038/s41401-021-00697-2
Zhang, L., Lin, D., Sun, X., Curth, U., Drosten, C., Sauerhering, L., et al. (2020c). Crystal Structure of SARS-CoV-2 Main Protease Provides a Basis for Design of Improved α-ketoamide Inhibitors. Science 368, 409–412. doi:10.1126/science.abb3405
Zhang, L., Poorbaugh, J., Dougan, M., Chen, P., Gottlieb, R. L., Huhn, G., et al. (2021c). Endogenous Antibody Responses to SARS-CoV-2 in Patients with Mild or Moderate COVID-19 Who Received Bamlanivimab Alone or Bamlanivimab and Etesevimab Together. Front. Immunol. 12, 790469. doi:10.3389/fimmu.2021.790469
Zhang, R., Duffy, B. F., Lange, V., Eby, C. S., and Liu, C. (2019). Association between the HLA-Drb1*03:01-Dqb1*02:01 Haplotype and PF4/heparin Antibodies. Blood Adv. 3, 3136–3142. doi:10.1182/bloodadvances.2019000311
Zhang, X., Zhang, X., Wang, X., and Zhao, M. (2018). Influence of Andrographolide on the Pharmacokinetics of Warfarin in Rats. Pharm. Biol. 56, 351–356. doi:10.1080/13880209.2018.1478431
Zhao, Y.-H., Zhao, L., Yang, X.-C., and Wang, P. (2021). Cardiovascular Complications of SARS-CoV-2 Infection (COVID-19): a Systematic Review and Meta-Analysis. Rev. Cardiovasc. Med. 22, 159–165. doi:10.31083/j.rcm.2021.01.238
Zhou, Y., Ingelman-Sundberg, M., and Lauschke, V. M. (2017). Worldwide Distribution of Cytochrome P450 Alleles: A Meta-Analysis of Population-Scale Sequencing Projects. Clin. Pharmacol. Ther. 102, 688–700. doi:10.1002/cpt.690
Zhu, F.-C., Guan, X.-H., Li, Y.-H., Huang, J.-Y., Jiang, T., Hou, L.-H., et al. (2020). Immunogenicity and Safety of a Recombinant Adenovirus Type-5-Vectored COVID-19 Vaccine in Healthy Adults Aged 18 Years or Older: a Randomised, Double-Blind, Placebo-Controlled, Phase 2 Trial. Lancet (London, England) 396, 479–488. doi:10.1016/S0140-6736(20)31605-6
Zhu, W., He, X., Cheng, K., Zhang, L., Chen, D., Wang, X., et al. (2019). Ankylosing Spondylitis: Etiology, Pathogenesis, and Treatments. Bone Res. 7, 22. doi:10.1038/s41413-019-0057-8
Keywords: COVID-19, pathogenesis and severity, repurposed drugs, pharmacogenetics, molecular docking, drug-drug interactions, drug-herb interactions, precision medicine
Citation: Biswas M, Sawajan N, Rungrotmongkol T, Sanachai K, Ershadian M and Sukasem C (2022) Pharmacogenetics and Precision Medicine Approaches for the Improvement of COVID-19 Therapies. Front. Pharmacol. 13:835136. doi: 10.3389/fphar.2022.835136
Received: 15 December 2021; Accepted: 24 January 2022;
Published: 18 February 2022.
Edited by:
Giosuè Costa, University of Catanzaro, ItalyReviewed by:
Dmitry A. Sychev, Russian Medical Academy of Continuous Professional Education, RussiaIsabella Romeo, University of Catanzaro, Italy
Copyright © 2022 Biswas, Sawajan, Rungrotmongkol, Sanachai, Ershadian and Sukasem. This is an open-access article distributed under the terms of the Creative Commons Attribution License (CC BY). The use, distribution or reproduction in other forums is permitted, provided the original author(s) and the copyright owner(s) are credited and that the original publication in this journal is cited, in accordance with accepted academic practice. No use, distribution or reproduction is permitted which does not comply with these terms.
*Correspondence: Chonlaphat Sukasem, chonlaphat.suk@mahidol.ac.th