- 1Biotechnology Division, CSIR-Institute of Himalayan Bioresource Technology, Palampur, India
- 2Academy of Scientific and Innovative Research (AcSIR), Ghaziabad, India
Sinopodophyllum hexandrum is an endangered medicinal herb known for its bioactive lignan podophyllotoxin (PTOX), which is used for the preparation of anticancer drugs. In its natural habitat, S. hexandrum is exposed to a multitude of adversities, such as fluctuating temperatures, water deficit, and high UV radiations. Transcriptional regulation of genes, which is regulated by the condition-specific binding of transcriptional factors to precise motifs in the promoter region, underlines responses to an environmental cue. Therefore, analysis of promoter sequences could ascertain the spatio-temporal expression of genes and overall stress responses. Unavailability of genomic information does not permit such analysis in S. hexandrum, especially on regulation of PTOX pathway. Accordingly, this study describes isolation and in silico analysis of 5′-upstream regions of ShPLR (PINORESINOL-LARICIRESINOL REDUCTASE) and ShSLD (SECOISOLARICIRESINOL DEHYDROGENASE), the two key genes of the PTOX biosynthetic pathway. Data showed a range of motifs related to basal transcription, stress-responsive elements, such as those for drought, low temperature, and light, suggesting that the expression of these genes and resulting PTOX accumulation would be affected by, at least, these environmental cues. While the impact of temperature and light on PTOX accumulation is well studied, the effect of water deficit on the physiology of S. hexandrum and PTOX accumulation remains obscure. Given the presence of drought-responsive elements in the promoters of the key genes, the impact of water deficit on growth and development and PTOX accumulation was studied. The results showed decline in relative water content and net photosynthetic rate, and increase in relative electrolyte leakage with stress progression. Plants under stress exhibited a reduction in transpiration rate and chlorophyll content, with a gradual increase in osmoprotectant content. Besides, stressed plants showed an increase in the expression of genes involved in the phenylpropanoid pathway and PTOX biosynthesis, and an increase in PTOX accumulation. Upon re-watering, non-irrigated plants showed a significant improvement in biochemical and physiological parameters. Summarily, our results demonstrated the importance of osmoprotectants during water deficit and the revival capacity of the species from water deficit, wherein PTOX synthesis was also modulated. Moreover, isolated promoter sequences could be employed in genetic transformation to mediate the expression of stress-induced genes in other plant systems.
Introduction
Sinopodophyllum hexandrum (Royle) is a well-known endangered medicinal herb that contains a medicinally important lignan, podophyllotoxin (PTOX), in its underground parts, rhizomes, and roots. PTOX is used as a precursor for anticancer drugs, such as etoposide and teniposide (Wang et al., 2013; Rather and Amin, 2016; Kumari et al., 2017). As a bioactive compound, PTOX also provides the substrate for the synthesis of botanical pesticides (Lv et al., 2020). S. hexandrum can propagate through seeds and rhizomes; however, due to the poor and erratic seed germination (Kharkwal et al., 2008; Sreenivasulu et al., 2009; Dogra et al., 2013), the rhizome is the prime method of reproduction. Because of the presence of PTOX in underground parts, the whole plant is uprooted, thus, resulting in the rapid extinction of the species from its natural habitat. As an outcome, S. hexandrum is listed as an endangered species in the Indian Himalayas (Kala, 2000; Shah, 2006).
Besides human interference, environmental adversities in the natural habitat also influence the survival and propagation of several high-altitude plant species such as S. hexandrum. These adversities include fluctuating temperature (warmer days and cooler nights), high-intensity radiations such as UV-B, low partial pressure of gases, and inadequate availability of water, which at times can create drought-like conditions (Tewari et al., 2017). Despite such unfavorable conditions, S. hexandrum can sustain and complete its life cycle (Kumari et al., 2014). Deciphering the mechanism(s) by which S. hexandrum deals with these cues is essential to understand the adaptability of this high-altitude extremophile.
Although the complete pathway of PTOX biosynthesis remains to be elucidated, a putative pathway has been derived from studies on S. hexandrum, Anthriscus, Linum, and Dysosma (Kumari et al., 2017). The suggested pathway involves a general phenylpropanoid pathway that diverges after the formation of feruloyl-CoA (Supplementary Figure 1). Briefly, the phenylpropanoid pathway initiates with the conversion of phenylalanine to cinnamic acid by PHENYLALANINE AMMONIA LYASE (PAL). Cinnamic acid is converted to p-coumarate by CINNAMATE-4-HYDROXYLASE (C4H), which is further converted to p-coumaroyl CoA by 4-COUMAROYL CoA LIGASE (4CL) (Vogt, 2010). p-Coumaroyl CoA is converted into p-Coumaroyl shikimate by HYDROXYCINNAMOYL COA:SHIKIMATE HYDROXYCINNAMOYL TRANSFERASE (HCT). COUMARATE 3-HYDROXYLASE (C3H) catalyzes the hydroxylation of p-Coumaroyl shikimate to caffeoyl shikimate that, in turn, is converted to caffeoyl-CoA by HCT. Caffeoyl-CoA is methylated to feruloyl-CoA by CAFFEOYL-CoA O-METHYLTRANSFERASE (CCoAOMT). Caffeoyl-CoA can also be converted to caffeic acid by 4-COUMAROYL CoA LIGASE (4CL). Caffeic acid is methylated to ferulic acid by CAFFEIC ACID 3-O-METHYLTRANSFERASE (COMT). Ferulic acid then acts as a substrate for the synthesis of sinapic acid and lignin. In addition, ferulic acid can also be converted to feruloyl-CoA by 4CL. The conversion of feruloyl-CoA to coniferaldehyde is catalyzed by CINNAMOYL-CoA REDUCTASE (CCR). CINNAMYL ALCOHOL DEHYDROGENASE (CAD) coverts coniferaldehyde to coniferyl alcohol, which then undergoes a dimerization reaction by DIRIGENT PROTEIN OXIDASE (DPO/DIR) to form pinoresinol. Pinoresinol acts as a branching point for the generation of a diversity of lignans. For PTOX biosynthesis, in an enantiospecific manner, PINORESINOL-LARICIRESINOL REDUCTASE (PLR) reduces pinoresinol in two steps to secoisolariciresinol, which is dehydrogenated to matairesinol by SECOISOLARICIRESINOL DEHYDROGENASE (SLD/SDH). Matairesinol is then converted to deoxypodophyllotoxin in five steps catalyzed by unknown enzymes. Deoxypodophyllotoxin is the precursor of PTOX and is proposed to be converted by DEOXYPODOPHYLLOTOXIN 7-HYDROXYLASE (DOP7H) (Supplementary Figure 1).
The sessile nature of plants necessitates rapid alteration of physiology and metabolic activities to endure adverse conditions in their habitat. Such alteration is governed by transcriptional regulation, which is mainly achieved by the binding of specific transcriptional factors to respective promoters. Transcriptional reprogramming then activates an array of signal transduction processes required to limit damage (Jin et al., 2019). Promoters are generally 5′-upstream DNA sequences governing the transcription of genes and are crucial targets to understand the molecular mechanism of various pathways. The binding between transcription factors (TFs) and cis-regulatory DNA sequences in the 5′-upstream of genes either activates or represses gene expression (Hernandez-Garcia and Finer, 2014; Biłas et al., 2016). In general, genes with related expression patterns contain common regulatory motifs in their promoter region and are likely to be regulated by common TFs (Klok et al., 2002). Characterization of genes and promoters of the PTOX biosynthesis pathway would answer the long-standing questions on: (i) response of PTOX biosynthesis to environmental variation(s) and (ii) regulation of the PTOX biosynthesis pathway. However, lack of genomic information in S. hexandrum limits answering these questions. As a first step, the issue can be dealt by cloning and characterization of full-length genes and cognate promoter sequences of the PTOX pathway (Kumari et al., 2014).
Given the natural habitat of S. hexandrum in the Himalayas at elevations ranging from 2,400 to 4,500 m above sea level (Kumari et al., 2014; Liu et al., 2014), fluctuating water availability and increased water deficit resulting from freezing and higher light intensity are anticipated to induce drought-like conditions. In addition, the changing climate leading to an increase in carbon dioxide levels and temperature (NOAA, 2020a,b) is also expected to increase drought or water deficit frequency in high altitude ecosystems (Cotado et al., 2020). Among various cues encountered in the natural habitat, the impact of temperature and light on growth and PTOX biosynthesis has been studied in S. hexandrum (Singh and Purohit, 1998; Kumari et al., 2014; Li et al., 2020; Lv et al., 2020). A previous study reported the impact of altitudinal gradient in Zanskar valley, a semi-cold desert region in India with limited water availability, on lignan content in S. hexandrum (Kitchlu et al., 2011), wherein the highest content of PTOX was recorded in plants collected from Tangoli (3,000 m asl) and Padam (3,800 m asl) as compared to those collected from Panikhar (2,800 m asl). The study discussed the positive impact of stress on the enhancement of PTOX content. However, this was a preliminary report and warranted an in-depth analysis.
In general, water deficit leads to both morphological and molecular alterations that are manifested in all phases of plant growth and development (Xu C. et al., 2019). Reduced water availability results in loss of turgor and impairment of growth, primarily because of reduction in gas exchange, photosynthetic efficiency, and nutrient uptake, and it assimilates partitioning (Farooq et al., 2009). The response towards water deficit is determined essentially by the severity and duration of water unavailability (Osmolovskaya et al., 2018). The adaptation of plants to endure water deficit involves the instigation of various physiological, biochemical, and molecular responses. These changes include osmotic adjustments, accumulation of antioxidants and secondary metabolites, and reduced assembly of the photosynthetic complex to prevent the generation of excess reactive oxygen species (ROS) (Laxa et al., 2019). Most of these changes are directed by the transcriptional expression of cognate genes that can be activated either by stress directly or via secondary signaling molecules (Janiak et al., 2016). Water deficit was also shown to reconfigure the phytoconstituent compositions in several plant species cultivated under semi-arid conditions (Farahani et al., 2009). Since water deficit profoundly affects physiology and metabolism (both primary and secondary) in plants (Osmolovskaya et al., 2018), it would be interesting to study how it affects the biosynthesis of PTOX in S. hexandrum.
With this background, this study describes isolation and analysis of promoter region of two genes of the PTOX biosynthesis pathway, namely, ShPLR and ShSLD. The upstream regions of both genes contain specific abiotic stress- and drought-responsive elements in addition to low temperature and high light-responsive elements, which suggested transcriptional reprogramming of these genes under respective environmental cues. Interestingly, S. hexandrum plants exhibited better water retention and accumulated osmoprotectants in response to drought, which perhaps protected the plants. Also, the genes involved in the phenylpropanoid and PTOX biosynthesis pathways were upregulated, leading to increased accumulation of PTOX under reduced water availability.
Materials and Methods
Plant Material and Growth Conditions
Plants of S. hexandrum, collected from the natural habitat (Parashar lake, Mandi; 2,730 m asl; 30°12′ N 77°47′ E, India), and maintained at CSIR-Institute of Himalayan Bioresource Technology, Palampur (1,300 m asl; 32°06′ N, 76°33′ E, India) were used for the experimental purpose. To avoid the impact of age/rhizome size, morphologically similar plants having comparable leaf and root size without any rhizome formation were selected for this study. The Biological Diversity Act 2002 of India permits bonafide Indians to collect plant samples from native habitats for scientific investigations (Venkataraman, 2009).
The plants were grown in potting mix (constituted of soil, sand, and farmyard manure in a ratio of 2:1:1) under a long day (16 h) photoperiod in a plant growth chamber (Percival Scientific, Perry, IA, United States) set at 25 ± 2°C with (photon flux density of 200 μmol m–2s–1 and relative humidity of 70 ± 10%), as described earlier (Kumari et al., 2014).
Isolation of 5′-Upstream Sequences and in silico Analysis
Two genes, namely ShPLR and ShSLD, involved in dedicated steps of PTOX biosynthesis pathway in S. hexandrum were selected for cloning of 5′-upstream sequences. Full-length coding sequences (CDS) of these genes were submitted at NCBI by our group. The accession numbers were EU240218 and GU324975 for ShPLR and ShSLD, respectively. The 5′-upstream sequences were cloned using Genome-Walker™ kit (Clontech, Mountain View, CA, United States). Briefly, genomic DNA was extracted from the leaf tissue using cetyltrimethylammonium bromide (CTAB) method (Doyle and Doyle, 1987). Four DNA libraries (DLs 1-4) were prepared by digesting the DNA separately with blunt-end digestive enzymes, namely, DraI (for DL1), EcoRV (for DL2), PuvII (for DL3), and StuI (for DL4). The digested products were purified and ligated to the genome walker adaptors. PCR-based DNA walking was performed with gene-specific primers (GSPs; designed from a region close to the 5′-end of the target genes; Supplementary Table 1) and adaptor primers AP1 and AP2 (provided in the GenomeWalker kit) using four libraries as templates. The PCR products were electrophoresed, gel-eluted, and ligated into a pGEM-T-Easy (Promega Corporation, Fitchburg, WI, United States) vector system. The ligated products were transformed into Escherichia coli DH5α competent cells, and the positive clones were screened by blue/white selection and colony PCR. Plasmids were isolated from the positive colonies and sequenced using a BigDye™ Terminator Cycle Sequencing kit (Applied Biosystems, Beverly, MA, United States) with an automated DNA sequencer, ABI 3130xl Genetic Analyzer (Applied Biosystems, Beverly, MA, United States). The primer sequences used for the cloning upstream sequences are listed in Supplementary Table 1. The upstream sequences were aligned using the BLASTN tool. In silico analyses of the retrieved promoter sequences were performed using online tools, PLACE1 and PlantCARE2 to identify putative cis-regulatory elements.
Water Deficit Treatment
The plants in the plant growth chamber were exposed to progressive water deficit by withholding water as follows: one set of plants watered every alternate day with 150 ml of water is considered as irrigated. The other set was subjected to water deficit by withholding water for 5, 10, and 15 days (until the plants showed wilting and chlorosis) and was considered as non-irrigated. After 15 days of withholding water, the non-irrigated plants were re-watered every alternate day with 150 ml of water for additional 15 days until the plants exhibited a phenotype comparable to that of the irrigated ones. Leaf and root tissues were collected from the irrigated and non-irrigated plants for further analyses. All the experiments were performed using three independent biological replicates.
Measurement of Soil Moisture Content, Relative Water Content, and Net Photosynthetic Rate
Soil moisture content (SMC) was measured using WET Sensor Moisture Meter (Delta-T Devices Ltd., Cambridge, United Kingdom) according to the manufacturer’s instructions. Relative water content (RWC) of the leaves was measured as described by Barrs and Weatherley (1962). Briefly, three leaf disks (10 mm diameter, average weight 45 ± 5 mg) were cut using a cork borer. Fresh weight (FW) was recorded, and the tissues were rehydrated with de-ionized (DI) water at 4°C for 24 h to record the turgid weight (TW). Rehydrated tissues were oven-dried at 70°C for 48 h, and the dry weight (DW) of the tissues was recorded. RWC was determined using the following formula: RWC (%) = [(FW – DW)/(TW – DW)] × 100.
A portable gas exchange measuring system, LI 6400 (IRGA; LI-COR Biosciences, Lincoln, United States), was used to measure net photosynthetic rate (PN). The measurements were made between 9 AM and 12 noon using 400 μmol mol–1 of CO2 and a photosynthetic photon flux density of 1,000 μmol m–2 s–1. Data on fully expanded leaves were recorded in three separate biological replicates.
Determination of Relative Electrolyte Leakage and Chlorophyll Content
Relative electrolyte leakage (REL) was determined in the leaf and root tissues according to the method described by Blum and Ebercon (1981), with slight modifications. Briefly, leaf disks (three in number, average weight 45 ± 5 mg/disk; cut using a cork borer of 10 mm diameter) were collected from the irrigated and non-irrigated plants and incubated for 24 h at 25°C in a glass vial containing 12 ml of deionized (DI) water. Electrical conductivity (EC1) was measured using a CyberScan PC 510 (Eutech Instruments Ltd., Singapore) conductivity meter. The tissues in the DI water were autoclaved, cooled, and final electrical conductivity (EC2) was measured. REL was calculated using the following formula: REL (%) = (EC1/EC2) × 100.
Total chlorophyll content was calculated using the method described by Arnon (1949). Briefly, 100 mg of leaf tissue was homogenized in liquid nitrogen followed by extraction with 10 ml of 80% acetone. The homogenate was centrifuged at 3,000 rpm for 10 min at 4°C in 3K30 refrigerated centrifuge (Sigma, Germany) to collect the supernatant. The absorbance of the supernatant was recorded at 645 and 663 nm using a SPECORD 200 (Analytik Jena, Jena, Germany) UV/visible spectrophotometer against a blank solvent (80% acetone). Chlorophyll content was calculated using the equation as follows: Chl (mg/l) = 20.2 Abs645 + 8.02 Abs663 and normalized on per g of fresh weight.
Measurement of Proline Content
Proline content was analyzed using the method of Bates et al. (1973), which is based on the formation of a toluene-soluble, brick-red-colored formazan by proline with ninhydrin in an acidic medium. Briefly, 300 mg of leaf tissue was ground to a fine powder in liquid nitrogen followed by extraction with 2 ml of 3% sulfosalicylic acid. The supernatant was collected through centrifugation at 13,000 rpm for 10 min at room temperature. The supernatant (600 μl) was collected and reacted with glacial acetic acid (600 μl) and an acidic ninhydrin reagent (600 μl, prepared by warming 1.25 g ninhydrin in 30 ml glacial acetic acid and 20 ml 6 M phosphoric acid) for 1 h at 100°C in a dry bath with moderate shaking. The reaction was terminated by cooling the tube on an ice bath; 1 ml of toluene was added to the reaction mixture, which was vortexed briefly to mix. Chromophore-containing toluene was extracted, and absorbance was recorded at 520 nm using a SPECORD 200 (Analytik Jena, Jena, Germany) UV/visible spectrophotometer and calculated using the standard curve of proline prepared using L-proline (cat no. P0380; Sigma-Aldrich, St. Louis, MO, United States).
Quantification of Soluble Sugars and Starch
For analysis of extractable soluble sugar, 100 mg of tissue was ground in liquid nitrogen followed by three times extraction with 5 ml of 80% ethanol (Chow and Landhäusser, 2004). After each extraction, tubes were centrifuged at 2,500 rpm for 10 min at 4°C; the supernatant was collected, pooled, and freeze-dried in a lyophilizer (Heto Maxi Dry Lyo, Germany). The residue was dissolved in 20 ml of 100 mM NaOH, and 10 ml of aliquot was used for the quantification of sugars. For starch analysis, the leftover pellet, after soluble sugar extraction, was treated with 50 ml of 35% perchloric acid at 90°C for 1 h. This was followed by filtration using a Whatman filter, and 10 ml aliquot was also used for starch determination. Soluble sugars (inositol, fructose, glucose, and sucrose) and starch content were quantified by ion chromatography (IC) using a Metrohm IC (Metrohm, Herisau, Switzerland) system equipped with an autosampler (45 IC Autosampler plus 1) and a pulsed amperometric detector (PAD) (945 Professional IC Vario). IC separation was performed on a Hamilton RCX-30 column (7 μm, 4.6 mm × 250 mm) using an isocratic mobile phase consisting of 100 mM NaOH. The injection volume was 20 μl, and an isocratic elution was carried out at a flow rate of 1 ml/min and 10.6 MPa of pressure. Analysis settings for sugars are provided by the company on its website3. Quantification was performed using external sugar standards.
Extraction and Estimation of Podophyllotoxin Content
Podophyllotoxin (PTOX) was extracted and estimated in root tissues as described previously (Kumari et al., 2014); PTOX content was not detectable in leaf tissue. Briefly, 100 mg root tissue was ground to a fine powder in liquid nitrogen, followed by intermittent grinding in 1 ml of 70% methanol. The resulting extract was transferred to a centrifuge tube, vortexed for 5 min, sonicated (Ultrasonic Cleaner, MC-109-MP; Oscar Ultrasonics Pvt. Ltd., Mumbai, India) for 10 min at 25°C, and centrifuged at 14,000 rpm for 5 min. The supernatant was collected and filtered through 0.22-μm filter (Millipore, Burlington, MA, United States). PTOX content was estimated using Acquity UPLC (Waters, Millford, MA, United States) equipped with a bridged ethyl hybrid workflow shield C18 (1.7-μm particles, 2.1 mm × 100 mm) analytical column (Waters Corp., Manchester, United Kingdom). Injection volume was 5 μl, and the mobile phase consisted of methanol:water (60:40); isocratic elution was carried out at a flow rate of 0.25 ml min–1. PTOX was monitored at 240 nm and quantified using standard PTOX (Sigma, United States). Three independent biological replicates were used for PTOX estimation.
Expression Analysis of Genes Involved in Podophyllotoxin Biosynthetic Pathway
Total RNA was isolated from the root tissues, as described earlier (Ghawana et al., 2011). RNA was reverse-transcribed using Superscript III (Invitrogen, Waltham, MA, United States), and the resulting cDNA was used for quantitative reverse transcription-PCR (qRT-PCR). Reactions were performed with three independent biological replicates using S. hexandrum ACTIN as an internal standard. Relative expression profile was generated using the Relative Expression Software Tool REST-2009 (Pfaffl, 2012). The primers used for qRT-PCR are listed in Supplementary Table 2.
Statistical Analysis
For each experiment, three independent biological replicates were used. The statistical significance of the data was established by one-way ANOVA with Duncan’s Multiple Range Test (P < 0.05) using Statistica version 7.0 (StatSoft Inc., United States).
Results and Discussion
Isolation and Identification of cis-Regulatory Elements in ShPLR and ShSLD
Deoxyribonucleic acid (DNA) sequences, generally the 5′-upstream of a gene, contain regulatory regions, namely, enhancers, silencers, and cis-acting motifs for the regulation of gene expression. Among these, cis-acting motifs provide sites for the binding of specific transcription factors, which can either enhance or repress the transcription. Therefore, analysis and functional validation of these cis-acting motifs in the promoter region holds high significance in understanding the transcriptional regulation of genes under different cues. For instance, the identification and characterization of stress-inducible promoters and cis-acting elements could be exploited for genetic transformation to generate stress-tolerant transgenic plants (Hernandez-Garcia and Finer, 2014). Lack of genomic information in S. hexandrum limits molecular exploration, such as the understanding of the molecular regulation of PTOX biosynthetic pathway. To deal with this limitation, we cloned 5′-upstream sequences of two genes for which the full-length CDSs were deduced earlier in our laboratory (ShPLR; EU240218, and ShSLD; GU324975). The Genome Walk PCR, using the four libraries (DLs 1-4), obtained multiple products of various sizes in both primary and secondary PCRs (Supplementary Figure 2). Among these products, fragments of ∼1 kb in DL1 in ShPLR and ∼700 bp in DL3 in ShSLD could be successfully cloned and sequenced (Supplementary Figure 2). The Genome Walking approach, thus, obtained a 1,034-bp 5′-upstream region of ShPLR (Figure 1 and Supplementary Figure 2), and a 785-bp region for ShSLD (Figure 2 and Supplementary Figure 2). Our previous experience in characterizing promoters of similar lengths in other plant species suggested that the present length of promoters was large enough to provide useful regulatory sequences controlling gene expression (Kawoosa et al., 2010, 2014; Bhuria et al., 2016). The promoter sequences were submitted to NCBI with accession numbers such as OK574340 for ShPLR and OK574341 for ShSLD.
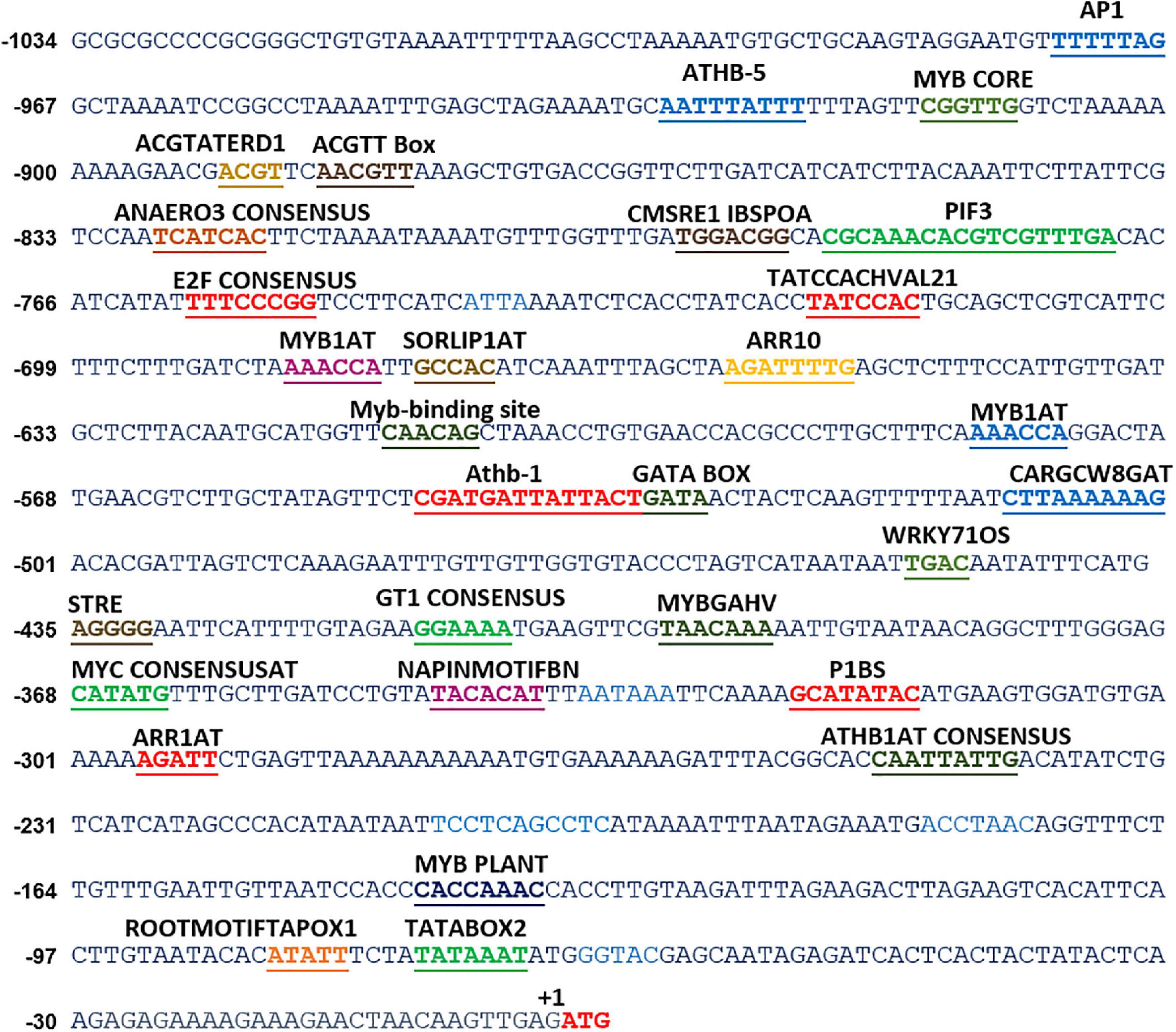
Figure 1. In silico analyses of various cis-acting elements in ShPLR. The analysis indicated the presence of various stress-responsive elements, such as those responding to light, temperature, and dehydration cues. Putative cis-acting regulatory elements are underlined. +1 indicates the translation start site.
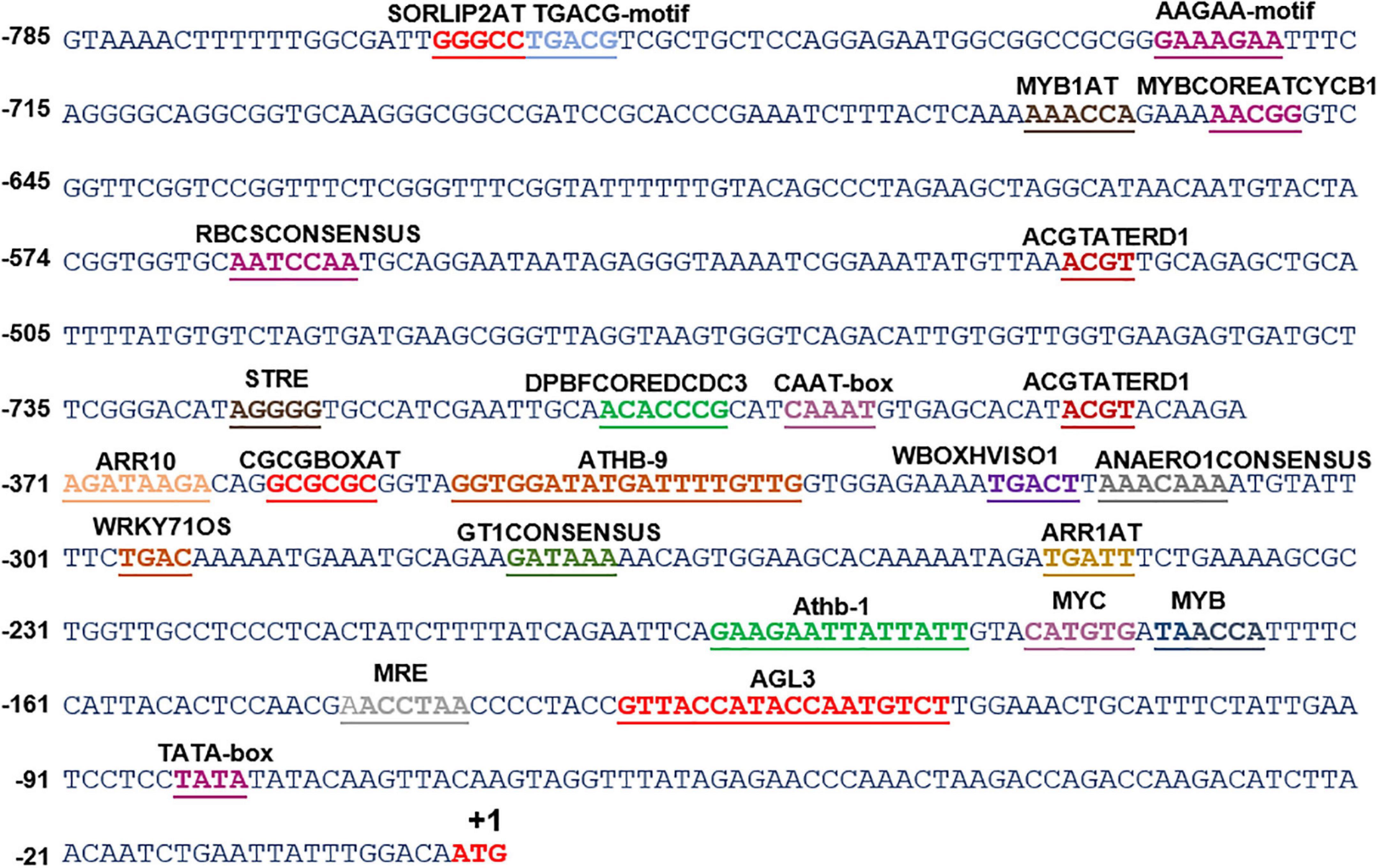
Figure 2. In silico analysis of various cis-acting elements in ShSLD. The analysis indicated the presence of various stress-responsive elements, such as those responding to light, temperature, and dehydration cues. Putative cis-acting regulatory elements are named and underlined. +1 indicates the translation start site.
In silico analysis of these putative promoter sequences revealed that the core promoter regions contain conserved TATA and CAAT boxes. In addition to core motifs, several regulatory motifs known to bind to light-, low- temperature-, drought- and hormone-responsive elements were identified in the upstream region of both genes. These motifs include those related to low temperature, light, ABA, drought/dehydration, gibberellins, cytokinin, auxin, signal transduction, leaf/plastid specificity, cell division, and biotic factors (Figures 1, 2 and Tables 1, 2).
Upstream region of ShPLR contains several drought-responsive cis-acting elements such as MYB transcription factor-binding sites, also called MYB-recognizing elements (MREs), which include MYBPLANT, MYB4, MYB1AT, MYBCORE, ACGTATERD1 (Figure 1 and Table 1). MREs regulate phenylpropanoid metabolism in plants, where binding of MYB transcription factors drives the expression of associated genes (Liu et al., 2015). With drought or ABA stress, MYBs bind key MREs to drive the expression of phenylpropanoid biosynthetic and stress-responsive genes (Xu Z. et al., 2019). Among the MYB binding sites, the MYBPLANT motif is reported to be involved in regulating phenylpropanoid and lignin biosynthesis in transgenic tobacco (Tamagnone et al., 1998), whereas MYBCORE, MYCCONSENSUSAT, MYB1AT, and ACGTATERD1 are recognized as dehydration-responsive elements (Abe et al., 2003). Similar to that of ShPLR, the upstream region of ShSLD also contains drought-responsive cis-acting elements, such as MRE, MYB, ACGTATERD1, and MYBCOREATCYCB1 (Figure 2 and Table 2). The MYC-like sequence (CATGTG) is known to be crucial for dehydration-induced expression of Early response to Dehydration 1 (ERD1) (Simpson et al., 2003). The ARR1 and ARR10 elements are also found in the upstream region of ShSLD. These are important signaling components involved in abiotic stress response (Nguyen et al., 2016). Light responsive elements like SORLIP2AT and GT1CONSENSUS, and motifs such as ARE and ANAERO1CONSENSUS, were also found in the 5′-upstream region of ShSLD. Other cis-elements, such as CGCGBOXAT, a Ca2+/calmodulin regulatory element, ACGTATERD1, a specific drought-responsive element (Simpson et al., 2003), and DPBFCOREDCDC3, an ABA-responsive element, were also present.
Collectively, the upstream region of both ShPLR and ShSLD genes comprises several cis-regulatory elements responding to light, low temperature, and dehydration stress. Interestingly, both of the genes contain cis-elements that are recognized by MYB and MYC family transcription factors, indicating their involvement in stress responses, such as drought stress (Singh and Laxmi, 2015). The in silico analysis reinforced that S. hexandrum encounters various environmental variations, such as drought or drought-like water deficit conditions. It has been reported that, like other high-altitude plants, S. hexandrum also experiences incidence of inadequate water availability and drought-like conditions (Kitchlu et al., 2011). However, the impact of such stress conditions on the physiology and PTOX biosynthesis remains uninvestigated. Therefore, to further understand the possible role of drought-responsive elements in the promoters of PTOX biosynthetic pathway genes, the consequences of water deficit and subsequent rehydration on the physiological and molecular responses of S. hexandrum were studied.
Reduction in Soil Moisture Content Affected Plant Phenotype, Relative Water Content, Photosynthetic Rate, and Relative Electrolyte Leakage in S. hexandrum
Drought or water deficit is a global stress that rigorously impacts plant productivity. While enormous literature exists on the impact of water deficit on crops and model plants, only a few studies have been carried out on high-altitude extremophile plants. Given the economic and medicinal importance of S. hexandrum, this study was performed to assess the impact of water deficit, which is prevalent at high altitudes.
The plants were exposed to drought-like conditions by withholding water. The non-irrigated plants showed a drought-like phenotype, where wilting and chlorosis of the leaves were observed (Figure 3A and Supplementary Figure 3). During the experiment, the non-irrigated plants showed a decline in SMC as compared with the irrigated plants. The SMC of the irrigated plants was 83.1 ± 1.9%, whereas the non-irrigated plants showed a significant and gradual decrease in SMC by 40.8 ± 7.4% on day 5, 15 ± 1.6% on day 10, and 6.6 ± 3.4% on day 15 of water withholding (Figure 3B). Re-watering recovered the SMC to 77 ± 1.09%, which was similar to that in irrigated plants (Figure 3B). Non-availability of water and reduction of SMC influence plant water status (Furlan et al., 2012). To understand the impact of reduced SMC on plants per se, RWC in the plants was measured. On day 0, RWC in the leaf tissue was 82.8 ± 1.7%. With the onset of water deprivation, the RWC of the non-irrigated plants decreased and showed a gradual decline with time as compared with that of the irrigated plants (Figure 3C). Irrigated plants could hold up to 83.3 ± 1.1% of RWC throughout the experiment, whereas the non-irrigated plants showed a significant decrease, with an RWC of 78.5 ± 5.4% on day 5, 70.1 ± 2.6% on day 10, and 62.6 ± 3.4% on day 15 of stress (Figure 3C). After re-watering, the RWC of the non-irrigated plants were recovered to the levels of the RWC of the irrigated ones. Water withholding significantly reduced the SMC (declined by up to 92.29%) (Figure 3B). However, the decrease in the RWC of the plants appeared less drastic (reduced by 24.4%) (Figure 3C), suggesting a better water holding capacity of S. hexandrum.
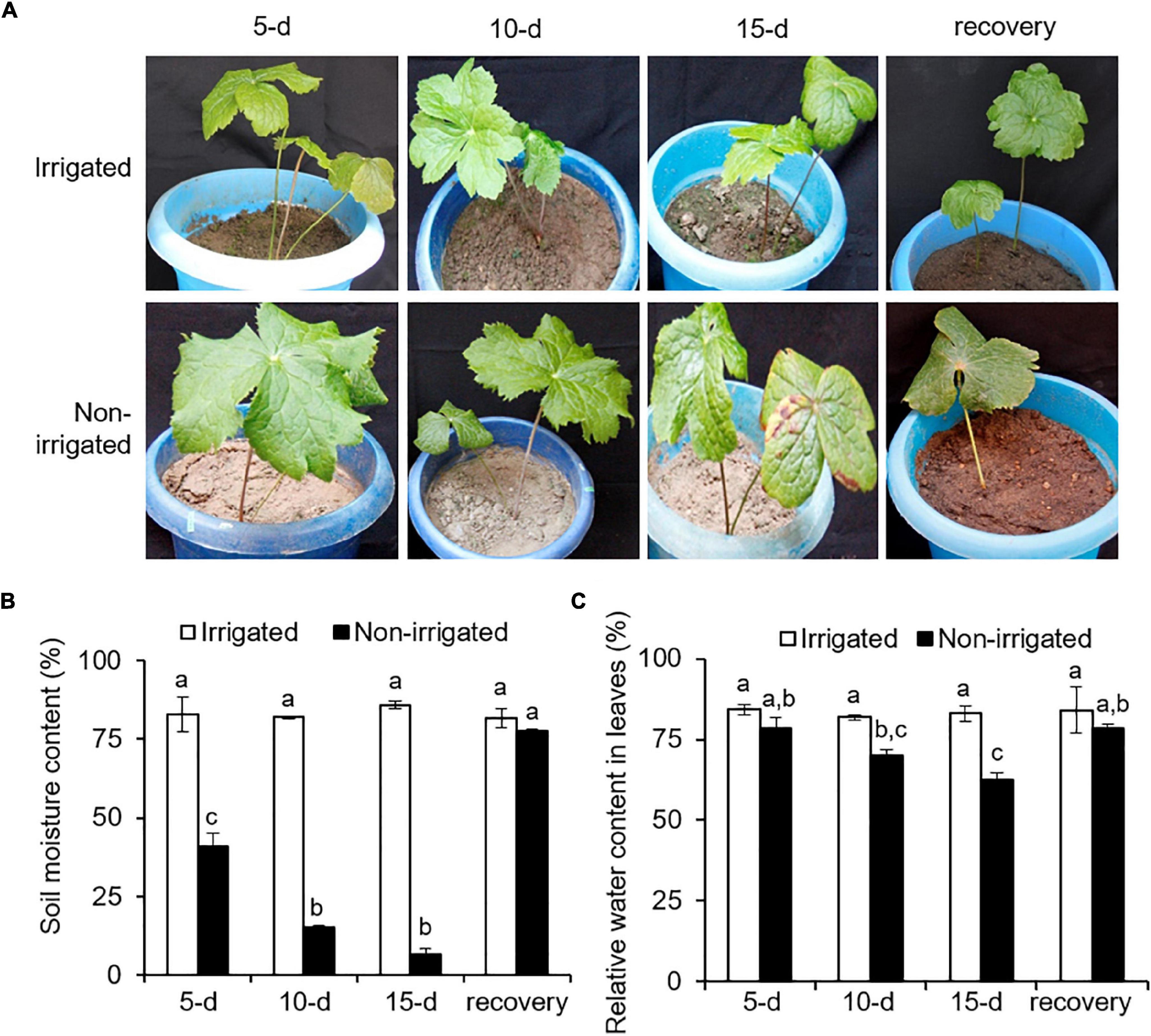
Figure 3. Sinopodophyllum hexandrum plants subjected to water deprivation showed a water deficit phenotype. (A) Plant phenotype. (B) Soil moisture content. (C) Relative water content in leaves. Watering of the plants was stopped, and plant phenotype and soil moisture content were recorded for 15 days with a 5-day interval, followed by re-watering for 15 days (recovery). Plants irrigated frequently served as control. In panels (B,C), data values show mean ± SE of three independent biological replicates. Lowercase letters represent statistical significance of differences between the mean values (P < 0.05, Duncan’s multiple comparison test).
Decline in the SMC and RWC of plants are the signature phenotype of water deficit (Keyvan, 2010; Soltys-Kalina et al., 2016). However, maintenance of RWC is implicated with water deficit tolerance, as reported on several plant species, such as tomato (Rao et al., 2000), sunflower (Jamaux et al., 1997), and barley (Altinkut et al., 2001). Higher water content is concomitant with osmotic adjustment and elasticity of the cell wall of tissues (Morgan, 1984; Ritchie et al., 1990). Since water deficit is inevitable for S. hexandrum in its niche because of seasonal availability of water, maintenance of RWC could be one of the inherent approaches of S. hexandrum to cope with stress.
Under water deficit conditions, plants tend to maintain RWC by preventing transpirational water loss, which is achieved by closing the stomata (Brodribb and Holbrook, 2003). However, this also represses gas exchange, leading to reduced availability of CO2 for the Calvin cycle and affects the overall photosynthetic rate (Chaves et al., 2009). As anticipated, the non-irrigated S. hexandrum plants exhibited a reduction in transpiration rate, consequently repressing photosynthesis rate upon water deprivation (Figures 4A,B). On day 15 of the water deficit, transpiration rate was reduced by 38.9 ± 17.5%, and the rate of photosynthesis declined by 66.7 ± 16.7% (Figures 4A,B). The rate of transpiration and net photosynthesis was recovered upon re-watering, although they remained significantly lower than that of the irrigated plants (Figures 4A,B).
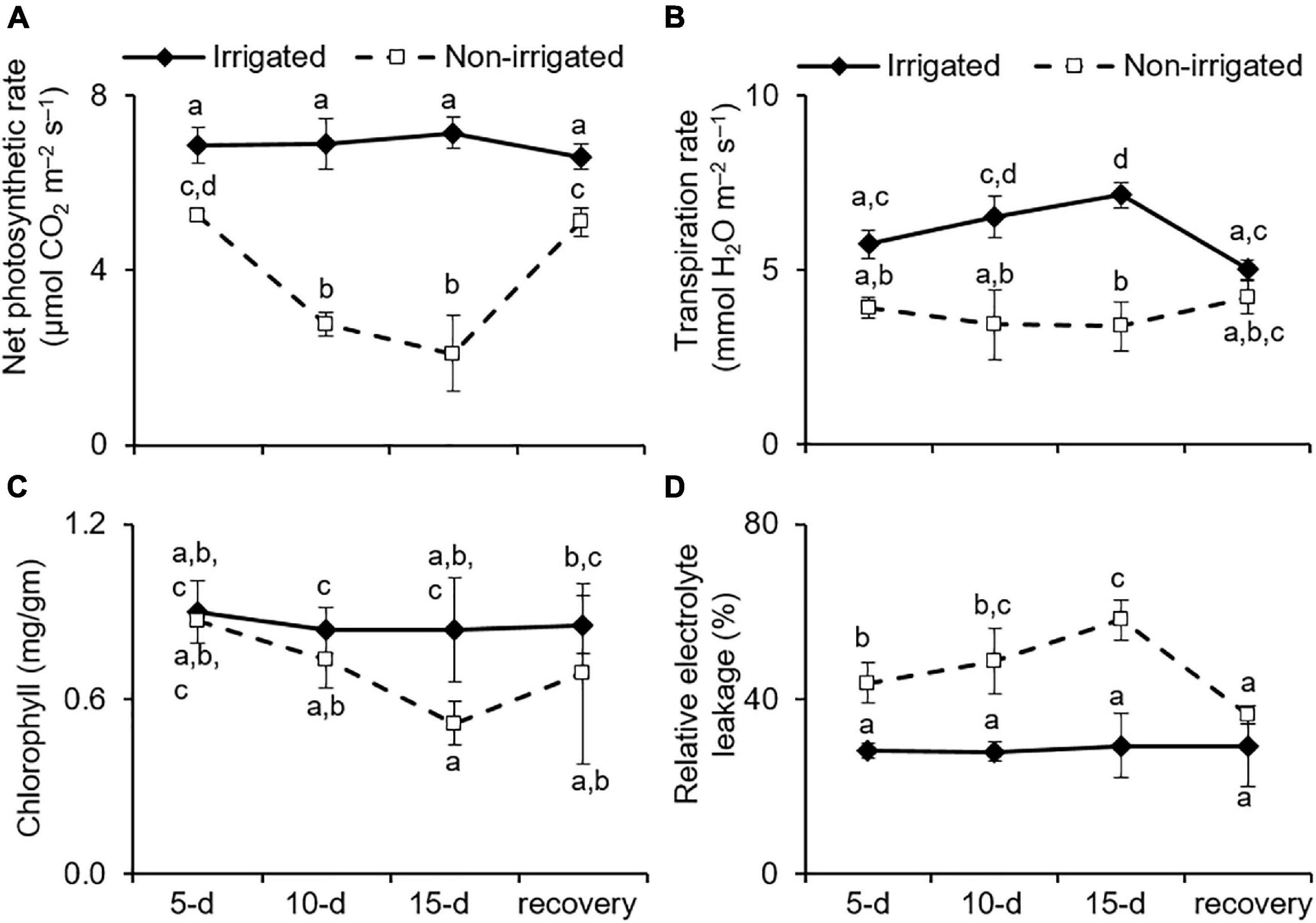
Figure 4. Water deficit negatively impacted the physiology of S. hexandrum. (A) Net photosynthetic rate. (B) Transpiration rate. (C) Chlorophyll content. (D) Relative electrolyte leakage showing membrane damage. The data values show mean ± SE of three independent biological replicates. Lowercase letters represent statistical significance of differences between the mean values (P < 0.05, Duncan’s multiple comparison test).
Given the appearance of lesions, such as wilting and chlorosis of the leaves of water-deprived plants (Figure 3A), the impact of water deficit on chlorophyll content and membrane damage was analyzed. Both chlorophyll content and membrane damage have an impact on photosynthetic efficiency and are implicated in stress tolerance of the plants (Sengupta et al., 2019; Wungrampha et al., 2019; Joshi et al., 2020). The data showed that chlorophyll content was significantly reduced with stress (declined by 32.4 ± 8.7% on day 15 of stress) and recovered by 24.7% after re-watering (Figure 4C). On the contrary, REL, which reflects membrane damage, was significantly higher in the non-irrigated plants as compared to the irrigated plants. The REL showed a dose-dependent increase with stress and could largely recover after re-watering (Figure 4D). The REL of the leaf tissue of irrigated plants did not show any significant change (28.7 ± 0.67%) during the course of the experiment, whereas in the non-irrigated plants, the REL increased to 43.7 ± 4.7 (day 5), 48.7 ± 7.4 (day 10), and 58.1 ± 2.69% (day 15) (Figure 4D). Upon re-watering, the REL of the non-irrigated plants exhibited reduction to 36.47 ± 2.08%, which accounted for 37% recovery (Figure 4D).
Sinopodophyllum hexandrum exhibited a typical phenotype of water deficit upon water deprivation, showing reduced photosynthesis and transpiration rate coupled with reduction in chlorophyll content and increased cell death; however, the impact was not that severe. Moreover, the plants could ably recover once they were watered again. The stress tolerance and recovery capability of the S. hexandrum species could be due to the maintenance of high relative water content and osmotic adjustments. Such an ability to efficiently recover is a desirable trait for the survival of the species where water deficit is inevitable.
Increased Proline and Total Soluble Sugar Contents Appear to Protect S. hexandrum From Water Deprivation
Proline is known to be an osmoprotectant, reactive oxygen species scavenger, and molecular chaperone that protects cells from stress-induced damage (Szabados and Savoure, 2010). Plants combat water deficit via osmotic adjustment (Morgan, 1984), which is achieved by accumulating secondary metabolites and proline (McCue and Hanson, 1990; Hare and Cress, 1997). Similarly, S. hexandrum tended to accumulate proline under water deprivation conditions. In the irrigated plants, proline content was almost constant. However, in the non-irrigated plants, it increased by 24.5 ± 7.9% on day 5, 62.1 ± 8.6% on day 10, and 75.1 ± 2.9% on day 15 of stress, and compared to the irrigated plants, proline content was almost fivefold higher in the leaf tissue on day 15 of stress (Figure 5A). After re-watering for 15 days, proline content declined to 26.3 ± 5.8%, accounting for almost 66% recovery in the leaves of the non-irrigated plants.
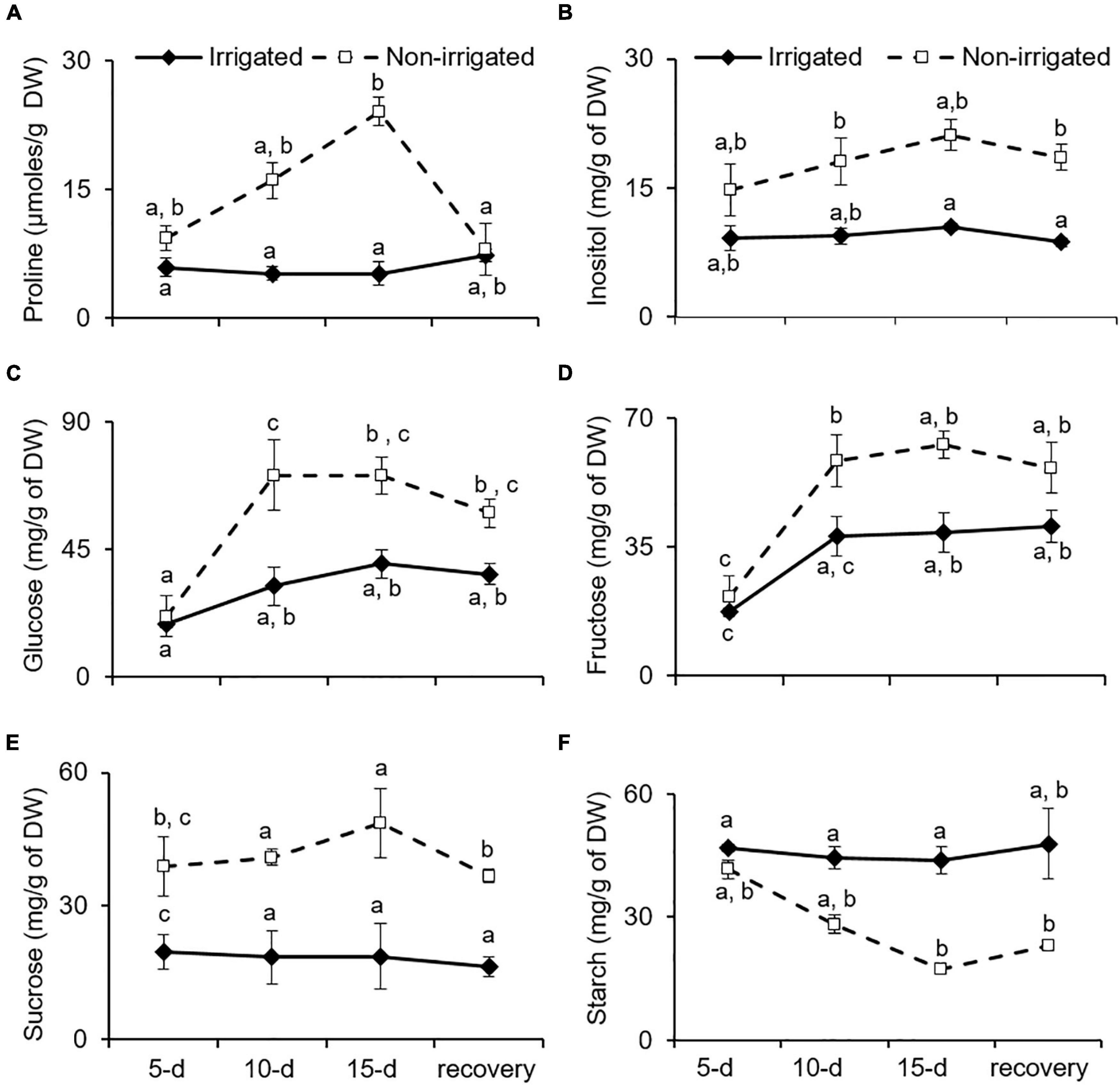
Figure 5. Osmoprotectants tend to accumulate with water deficit in S. hexandrum. (A) Proline. (B) Insolitol. (C) Glucose. (D) Fructose. (E) Sucrose. (F) Starch. The data values show mean ± SE of three independent biological replicates. Lowercase letters represent statistical significance of differences between the mean values (P < 0.05, Duncan’s multiple comparison test).
Accumulation of sugars also aids in osmotic adjustments and is implicated in recovery from water deficit (Sheffer et al., 1979; Busso et al., 1990; McCue and Hanson, 1990). Given the importance of sugars in stress management, the content of various sugars was analyzed under water deficit conditions in S. hexandrum (Figures 5B–E). In the irrigated plants, glucose and fructose exhibited increase with age of the plants, whereas inositol and sucrose remained almost constant. On the contrary, all the four soluble sugars increased with stress in the non-irrigated plants (Figures 5B–E). Upon re-watering, the sugars showed a slight decline in the non-irrigated plants but remained much higher than in the irrigated ones (Figures 5B–E). Inositol levels recovered by 12.2 ± 3.9%, glucose by 18.5 ± 12.4%, fructose by 20 ± 5.9%, and sucrose by 24.4 ± 5.5%. Interestingly, in comparison to the soluble sugars, starch showed a negative trend where it remained almost similar in the irrigated plants, whereas it declined with stress in the non-irrigated plants and exhibited a 2.5-fold decrease on day 15 of stress. Upon re-watering, the starch content in the non-irrigated plants recovered by 24.3 ± 3% but remained almost twofold lesser than in the irrigated plants (Figure 5F).
Increased accumulation of free amino acids, such as proline and soluble sugars, helps in re-adjusting the osmoticum to tackle the water deficit conditions (McCue and Hanson, 1990). Soluble sugars also underscore the capability of plants to recover from water deficit (Sheffer et al., 1979; Busso et al., 1990). Besides acting as osmoprotectants, soluble sugars are also involved in stress signaling during water deficit (Nelson et al., 1998). Accordingly, a higher content of free soluble sugars was observed in water-deprived S. hexandrum as compared with the irrigated ones. Higher free sugar content could be either due to increased biosynthesis or increased breakdown of starch (Pattanagul and Madore, 1999; Chaves et al., 2009). In non-irrigated S. hexandrum, starch content declined with stress in proportion to the increased levels of free sugars (Figures 5B–F), suggesting a breakdown of starch, as also reported earlier (Pattanagul and Madore, 1999; Chaves et al., 2009). In summary, increased content of proline and soluble sugars in S. hexandrum indicates efficient immobilization of amino acids and inclusive use of stored carbohydrate reserve for imparting better survival under water deficit and assistance in recovery afterward.
Water Deficit Promotes the Accumulation of Podophyllotoxin via Transcriptional Upregulation of Genes Involved in Its Biosynthesis and Is Coupled With Changes in Starch and Soluble Sugar Contents
Plants accumulate secondary metabolites when experiencing adverse environmental conditions (Savoi et al., 2016; Liu et al., 2019), which is mainly achieved via transcriptional reprogramming of genes involved in the biosynthesis of such metabolites. Therefore, investigating the expression of genes associated with secondary metabolite synthesis contributes to the understanding of stress-responsive mechanisms (Yuan et al., 2012).
Podophyllotoxin (PTOX) is a secondary metabolite, and is synthesized via phenylpropanoid pathway (Kumari et al., 2014). Given the negative effect of water deficit on growth and physiology, and PTOX being a secondary metabolite, its biosynthesis was anticipated to be affected. In both, irrigated and non-irrigated plants, PTOX content increased with age, irrespective of the watering condition (Figure 6A). In addition, although the difference was not significant on days 5 and 10, 12.1% more accumulation was observed on day 15 of stress in the non-irrigated plants as compared with the irrigated ones (Figure 6A). Upon re-watering, the PTOX content in the non-irrigated plants continued to increase. Interestingly, the rate of increase in the non-irrigated plants was significantly higher with 18.5% more PTOX content than in the irrigated ones (Figure 6A). These data suggested that both water deficit conditions as well as the release, promoted PTOX accumulation in the S. hexandrum plants.
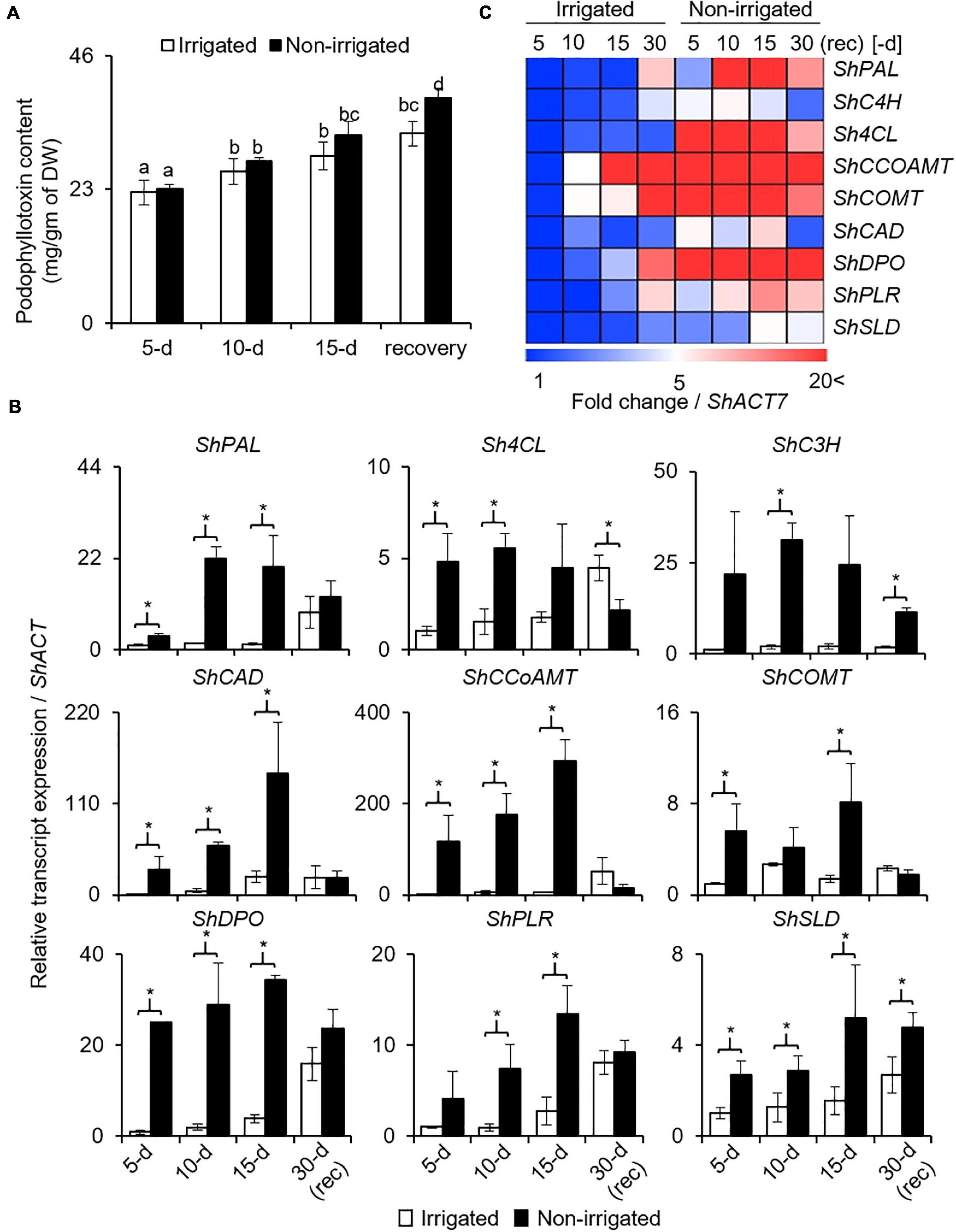
Figure 6. Podophyllotoxin (PTOX) content and expression of biosynthetic pathway genes in S. hexandrum increased in response to water deficit. (A) PTOX content. Data show mean ± SE of three independent biological replicates. Lowercase letters represent statistical significance of differences between the mean values (P < 0.05, Duncan’s multiple comparison test). (B) Transcript level expression of PTOX biosynthetic pathway genes. Gene names are prefixed with Sh that stands for Sinopodophyllum hexandrum. ShACTIN was used as an internal control. Data represent mean ± SD of three independent biological replicates. Asterisk show statistical significance of differences between the mean values (P < 0.05, Student’s t-test). (C) Heat map showing the expression of PTOX biosynthetic pathway genes as shown in panel (B).
Concomitantly, key genes of the phenylpropanoid pathway, namely, ShPAL, Sh4CL, ShC3H, ShCCoAOMT, ShCOMT, and ShCAD, exhibited upregulation upon experiencing water deprivation (Figures 6B,C). Interestingly, all these genes were sensitive to stress and increased rapidly in the initial phase of stress. Thereafter, upregulation was gradual. The increased expression of phenylpropanoid pathway genes indicated the accumulation of secondary metabolites, such as sinapic acid, lignin, and flavanols, which are known to have protective roles (Cheynier et al., 2013; Le Roy et al., 2016; Pereira, 2016). Interestingly, upon re-watering, the genes of the phenylpropanoid pathway exhibited downregulation, although the expression was slightly higher as compared to the irrigated control (Figures 6B,C).
Furthermore, three key genes of the PTOX biosynthetic pathway, ShDPO, ShPLR, and ShSLD, exhibited upregulation in response to water deprivation. Concomitantly, PTOX also accumulated during this time period, suggesting a role of these genes in PTOX biosynthesis and that both genes as well as metabolites responded to the condition of water deprivation (Figure 6A). Similar to phenylpropanoid pathway genes, the expression of PTOX-specific genes was also reduced upon re-watering; however, it remained higher than the irrigated controls. Comparatively, the higher expression of genes of both the phenylpropanoid and PTOX biosynthetic pathways explains why even after recovery, PTOX content was higher in S. hexandrum (Figure 6A).
Besides stress conditions, there was an age-dependent gradual increase in the expression of genes involved in both the phenylpropanoid and PTOX biosynthetic pathways. This result indicates why the accumulation of PTOX was increasing even in the absence of stress (Figures 6A–C). Although it needs further investigations, it is likely that increasing hormone levels with age could also play an important role in PTOX accumulation. This assumption agrees with earlier studies where methyl jasmonate-induced ROS generation was shown to stimulate the expression of genes of the PTOX biosynthetic pathway leading to PTOX accumulation (Hazra et al., 2017; Biswas et al., 2021). Changes in the expression level of ShPLR and ShSLD under drought-like conditions are in accordance with the presence of drought-responsive cis-elements in their promoters and indicate their engrossment in stress responses. Experimental validation in the future would narrow down the importance of these individual cis-elements.
Water deficit is known to influence the expression of genes involved in secondary metabolite biosynthesis leading to differential accumulation of phenolics and other secondary metabolites (Becerra-Moreno et al., 2015; Caser et al., 2019; Babaei et al., 2021; Jogawat et al., 2021). Although water deficit, like other stresses, has a positive impact on secondary metabolite synthesis, the accumulation varies in terms of carbon-based (phenolics and terpenes) and nitrogen-containing (alkaloids and cyanogenic glycosides) secondary metabolites (Al-Gabbiesh et al., 2015; Sanchita et al., 2015). For instance, Labisia pumila (Jaafar et al., 2012), Hypericum resilience (de Abreu and Mazzafera, 2005), Trachyspermum ammi (Azhar et al., 2011), and Pisum sativum (Nogués et al., 1998) accumulate total phenolics and flavonoids under water deficit conditions. Similarly, Salvia officinalis and Pinus sylvestris accumulate monoterpenes (Turtola et al., 2003; Nowak et al., 2010) upon water deficit. On the contrary, increased accumulation of alkaloids in Senecio longilobus (Briske and Camp, 1982), Lupinus angustifolius (Christiansen et al., 1997), and Catharanthus roseus (Jaleel et al., 2007) have been reported under reduced water availability conditions. According to carbon-nutrient balance (CNB) theory, the amount of secondary metabolites in plants is directly regulated by relative abundances of carbon and nitrogen (Bryant et al., 1983; Hamilton et al., 2001). When nitrogen deficiency limits plant growth, carbohydrates tend to accumulate and increase the synthesis and the accumulation of carbon−based secondary metabolites. Conversely, when photosynthesis rate is reduced because of reduced availability of CO2 or being under light fluctuation, carbohydrates get a push toward growth, leading to lower carbon-nitrogen (C/N) ratio. This is likely to reduce the synthesis of carbon−based secondary metabolites and increase the synthesis of nitrogenous secondary compounds (Bryant et al., 1983; Herms and Mattson, 1992; Peñuelas and Estiarte, 1998). When S. hexandrum is experiencing water deficit, despite having reduced photosynthetic rate, it showed increased expression of phenylpropanoid pathway genes, indicating increased accumulation of carbon-based secondary metabolites, such as PTOX, which seems contrasting to the CNB theory. It is possible that water deficit-induced reduction in SMC might affect nutrient uptake leading to reduced availability of nitrogen and results in higher C/N ratio, favoring carbon-based secondary metabolites. There is a possibility that carbon supply was ensured by increased starch degradation in roots. We assessed this possibility by estimating the starch and soluble sugar content in roots under irrigated and non-irrigated conditions. As observed in the leaves, the roots also exhibited decrease in starch content and increase in soluble sugars (inositol, glucose, and fructose), suggesting increased degradation of starch (Figures 7A,B). Interestingly, accumulation of PTOX was proportional to both decrease in starch and increase in soluble sugar contents with stress (Figures 7A,B). Although it is not clear how increased starch degradation is precisely linked with PTOX accumulation, it has been demonstrated that increased starch degradation compensates for the carbon demand under stress conditions (Pilkington et al., 2015; Jia et al., 2021). Further studies investigating the secondary metabolome in S. hexandrum upon stress conditions, such as water deficit, would probably shed light on the pattern of secondary metabolite accumulation in this plant as well as in other high-altitude plants. Nevertheless, it is conceivable that the suppression of growth is liable for the enhanced accumulation of secondary metabolites in the S. hexandrum plants exposed to water deficit.
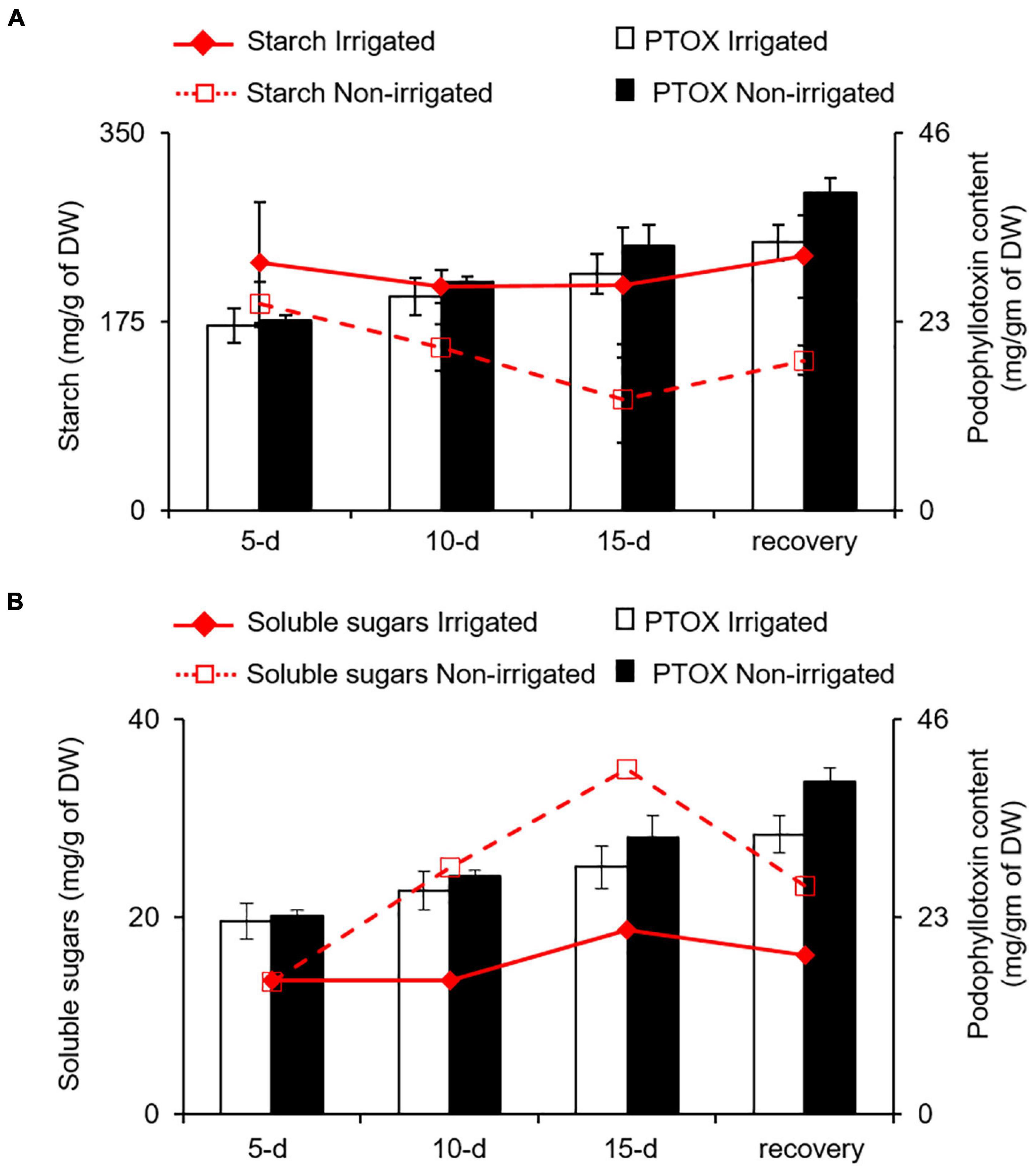
Figure 7. PTOX content in the roots of S. hexandrum under water deficit conditions is coupled with starch and soluble sugars contents. Line and bar graph showing the change in PTOX accumulation with (A) starch and (B) soluble sugar contents. In panel (B), soluble sugars represent the sum of inositol, glucose, and fructose in roots. The values represent the mean ± SE of three biological replicates.
Summarily, the presence of stress-responsive elements responding to light, temperature, and drought in the promoters of PTOX biosynthesis genes suggested their modulation under respective stress conditions. Accordingly, when subjected to water deficit, S. hexandrum showed better adaptation and sustainability (Figure 8). With reduced water availability, S. hexandrum plants exhibited reduced transpirational water loss. However, it declined gas exchange and CO2 availability that resulted in reduced photosynthetic rate. Reduced water availability also induced membrane damage, leading to cell death. Plants, in turn, accumulated less chlorophyll to avoid unnecessary harvesting of light, which could produce ROS if not used in photochemistry. Besides, osmoprotectants such as proline and free sugars were accumulated along with the PTOX. The increased accumulation of PTOX upon water deficit indicated its possible involvement in stress alleviation. However, this proposition awaits further investigations, which would also illuminate the biological role of PTOX.
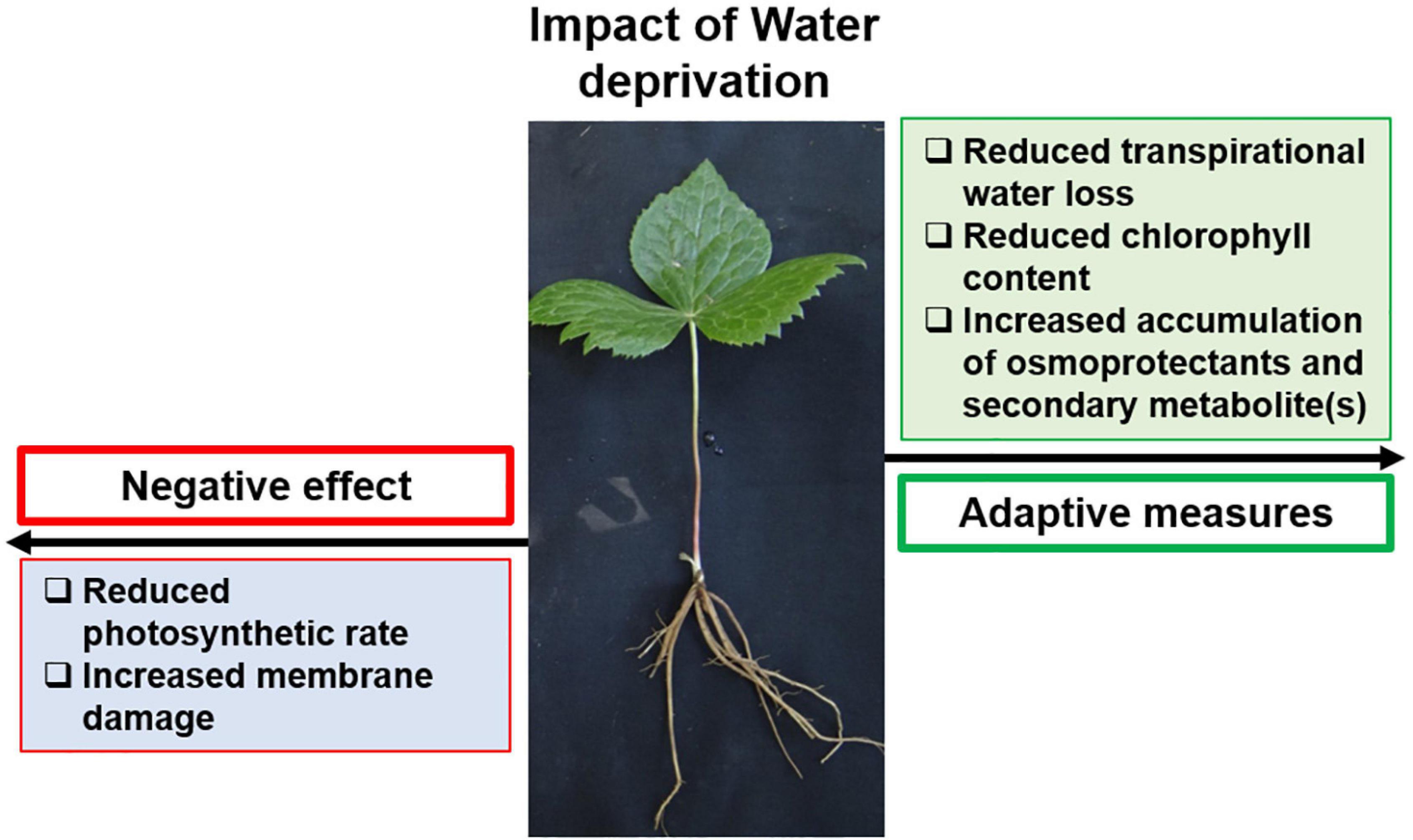
Figure 8. S. hexandrum implements an adaptive strategy to sustain under water deficit conditions. Water deprivation reduces soil moisture content and water availability to plants, and induces membrane damage and cell death. To counter this, S. hexandrum plants prevent excess transpirational water loss; however, this negatively impacts photosynthetic rate. In response, plants accumulate less chlorophyll, avoiding unnecessary light-harvesting and ROS generation. Besides, osmoprotectants, such as proline and free sugars, accumulate along with the secondary metabolite, PTOX, which helps the plants to sustain under non-permissive conditions.
Conclusion
In conclusion, the in silico analysis of the upstream regions of two PTOX biosynthesis pathway genes, namely ShPLR and ShSLD, identified stress-responsive elements implicated in response to water availability, light, and temperature stimuli, indicating that the expression of these genes could be modulated upon these environmental cues. Accordingly, both genes were upregulated under water-deprived conditions. In addition, S. hexandrum plants showed better adaptability to reduced water availability. Presence of cognate stress elements in the promoter regions of various genes seems to underscore the molecular basis of the better performance of S. hexandrum under water deficit conditions. Detailed characterization of these promoters would provide further insights into their applicability for engineering stress tolerance in other plants. Besides, increased accumulation of PTOX under limited water availability conditions indicates its possible involvement in stress endurance, helping the plant to adapt to non-permissive conditions. However, until now, there has been no direct experimental evidence supporting this proposition. Therefore, it is worth investigating the biosynthesis, steady-state levels, and mobilization of PTOX under stress conditions (namely high light, low temperature, and water deficit) and during recovery.
Data Availability Statement
The datasets presented in this study can be found in online repositories. The names of the repository/repositories and accession number(s) can be found in the article/Supplementary Material.
Author Contributions
SK conceived and designed the study and finalized the manuscript. AK performed the experimental work and wrote the initial draft of the manuscript. VD analyzed the data, prepared the figures, and wrote the manuscript. RJ helped in the drafting of the manuscript. All the authors have read and approved the final version of the manuscript.
Funding
This study was supported by the Council of Scientific and Industrial Research (CSIR), India, through the projects entitled “Pathway engineering and system biology approach toward homologous and heterologous expression of high-value phytoceuticals (artemisinin, picrosides, morphine, withanolides, podophyllotoxin (NWP-008),” “Plant diversity: studying adaptation biology and understanding/exploiting medicinally important plants for useful bioactives (SIMPLE-BSC, 0109),” and “Genomics of medicinal plants and agronomically important traits (PlaGen-BSC, 0107).” VD acknowledges the support from Ramalingaswami Re-entry Fellowship, Department of Biotechnology, Government of India (Grant No: BT/RLF/Re-entry/02/2019).
Conflict of Interest
The authors declare that the research was conducted in the absence of any commercial or financial relationships that could be construed as a potential conflict of interest.
Publisher’s Note
All claims expressed in this article are solely those of the authors and do not necessarily represent those of their affiliated organizations, or those of the publisher, the editors and the reviewers. Any product that may be evaluated in this article, or claim that may be made by its manufacturer, is not guaranteed or endorsed by the publisher.
Acknowledgments
The authors would like to thank the CSIR-IHBT for their infrastructural facilities. AK would like to thank to CSIR for the senior research fellowship. This study represents IHBT communication number 4742.
Supplementary Material
The Supplementary Material for this article can be found online at: https://www.frontiersin.org/articles/10.3389/fpls.2021.751846/full#supplementary-material
Abbreviations
DW, dry weight; FW, fresh weight; REL, relative electrolyte leakage; RWC, relative water content; SMC, soil moisture content.
Footnotes
- ^ https://www.dna.affrc.go.jp/PLACE/?action=newplace
- ^ http://bioinformatics.psb.ugent.be/webtools/plantcare/html/
- ^ http://www.metrohm.com/
References
Abe, H., Urao, T., Ito, T., Seki, M., Shinozaki, K., and Yamaguchi-Shinozaki, K. (2003). Arabidopsis AtMYC2 (bHLH) and AtMYB2 (MYB) function as transcriptional activators in abscisic acid signaling. Plant Cell 15, 63–78. doi: 10.1105/tpc.006130
Al-Gabbiesh, A., Kleinwächter, M., and Selmar, D. (2015). Influencing the contents of secondary metabolites in spice and medicinal plants by deliberately applying drought stress during their cultivation. Jord. J. Biol. Sci. 147, 1–10.
Altinkut, A., Kazan, K., Ipekci, Z., and Gozukirmizi, N. (2001). Tolerance to paraquat is correlated with the traits associated with water stress tolerance in segregating F2 populations of barley and wheat. Euphytica 121, 81–86.
Arnon, D. L. (1949). A copper enzyme is isolated chloroplast polyphenol oxidase in B eta vulgaris. Plant Physiol. 24, 1–15.
Azhar, N., Hussain, B., Ashraf, M. Y., and Abbasi, K. Y. (2011). Water stress mediated changes in growth, physiology and secondary metabolites of desi ajwain (Trachyspermum ammi L.). Pak. J. Bot. 43, 15–19.
Babaei, K., Moghaddam, M., Farhadi, N., and Pirbalouti, A. G. (2021). Morphological, physiological and phytochemical responses of Mexican marigold (Tagetes minuta L.) to drought stress. Sci. Hortic. 284:110116.
Barrs, H. D., and Weatherley, P. E. (1962). A re-examination of the relative turgidity technique for estimating water deficits in leaves. Aust. J. Biol. Sci. 15, 413–428.
Bates, L. S., Waldren, R. P., and Teare, I. D. (1973). Rapid determination of free proline for water-stress studies. Plant Soil 39, 205–207. doi: 10.1007/bf00018060
Becerra-Moreno, A., Redondo-Gil, M., Benavides, J., Nair, V., Cisneros-Zevallos, L., and Jacobo-Velázquez, D. A. (2015). Combined effect of water loss and wounding stress on gene activation of metabolic pathways associated with phenolic biosynthesis in carrot. Front. Plant Sci. 6:837. doi: 10.3389/fpls.2015.00837
Bhuria, M., Goel, P., Kumar, S., and Singh, A. K. (2016). The Promoter of AtUSP Is Co-regulated by Phytohormones and Abiotic Stresses in Arabidopsis thaliana. Front. Plant Sci. 7:1957. doi: 10.3389/fpls.2016.01957
Biłas, R., Szafran, K., Hnatuszko-Konka, K., and Kononowicz, A. K. (2016). Cis-regulatory elements used to control gene expression in plants. PCTOC 127, 269–287. doi: 10.1007/s11240-016-1057-7
Biswas, S., Hazra, S., and Chattopadhyay, S. (2021). Deep sequencing unravels methyl jasmonate responsive novel miRNAs in Podophyllum hexandrum. J. Plant Biochem. Biotechnol. doi: 10.1007/s13562-021-00698-6
Blum, A., and Ebercon, A. (1981). Cell membrane stability as a measure of drought and heat tolerance in wheat. Crop Sci. 21, 43–47.
Briske, D. D., and Camp, B. J. (1982). Water stress increases alkaloid concentrations in threadleaf groundsel (Senecio longilobus). Weed Sci. 30, 106–108.
Brodribb, T. J., and Holbrook, N. M. (2003). Stomatal closure during leaf dehydration, correlation with other leaf physiological traits. Plant Physiol. 132, 2166–2173. doi: 10.1104/pp.103.023879
Bryant, J. P., Chapin, F. S. III, Reichardt, P., and Clausen, T. (1983). Carbon/nutrient balance of boreal plants in relation to vertebrate herbivory. Oikos 40, 357–368. doi: 10.2307/3544308
Busso, C. A., Richards, J. H., and Chatterton, N. J. (1990). Non-structural carbohydrates and spring regrowth of two cool-season grasses: interaction of drought and clipping. J. Range Manage. 43, 336–343.
Caser, M., Chitarra, W., D’Angiolillo, F., Perrone, I., Demasi, S., Lovisolo, C., et al. (2019). Drought stress adaptation modulates plant secondary metabolite production in Salvia dolomitica Codd. Ind. Crops Prod. 129, 85–96. doi: 10.1016/j.indcrop.2018.11.068
Chaves, M. M., Flexas, J., and Pinheiro, C. (2009). Photosynthesis under drought and salt stress: regulation mechanisms from whole plant to cell. Ann. Bot. 103, 551–560. doi: 10.1093/aob/mcn125
Cheynier, V., Comte, G., Davies, K. M., Lattanzio, V., and Martens, S. (2013). Plant phenolics: recent advances on their biosynthesis, genetics, and ecophysiology. Plant Physiol. Biochem. 72, 1–20. doi: 10.1016/j.plaphy.2013.05.009
Chow, P. S., and Landhäusser, S. M. (2004). A method for routine measurements of total sugar and starch content in woody plant tissues. Tree Physiol. 24, 1129–1136. doi: 10.1093/treephys/24.10.1129
Christiansen, J. L., Jørnsgård, B., Buskov, S., and Olsen, C. E. (1997). Effect of drought stress on content and composition of seed alkaloids in narrow-leafed lupin, Lupinus angustifolius L. Eur. J. Agron. 7, 307–314.
Cotado, A., Garcia, M. B., and Munné-Bosch, S. (2020). Physiological seed dormancy increases at high altitude in Pyrenean saxifrage (Saxifraga longifolia Lapeyr.). Environ. Expt. Bot. 171:103929.
de Abreu, I. N., and Mazzafera, P. (2005). Effect of water and temperature stress on the content of active constituents of Hypericum brasiliense Choisy. Plant Physiol. Biochem. 43, 241–248. doi: 10.1016/j.plaphy.2005.01.020
Dogra, V., Ahuja, P. S., and Sreenivasulu, Y. (2013). Change in protein content during seed germination of a high altitude plant Podophyllum hexandrum Royle. J. Proteomics. 78, 26–38. doi: 10.1016/j.jprot.2012.10.025
Doyle, J., and Doyle, J. L. (1987). Genomic plant DNA preparation from fresh tissue-CTAB method. Phytochem. Bull. 19, 11–15.
Farahani, H. A., Valadabadi, S. A., Daneshian, J., Shiranirad, A. H., and Khalvati, M. A. (2009). Medicinal and aromatic plants farming under drought conditions. J. Hortic. For. 1, 086–092.
Farooq, M., Wahid, A., Kobayashi, N., Fujita, D., and Basra, S. M. A. (2009). “Plant drought stress: effects, mechanisms and management,” in Sustainable Agriculture, eds E. Lichtfouse, M. Navarrete, P. Debaeke, S. Véronique, and C. Alberola (Dordrecht: Springer), 153–188. doi: 10.1007/978-90-481-2666-8_12
Furlan, A., Llanes, A., Luna, V., and Castro, S. (2012). Physiological and biochemical responses to drought stress and subsequent rehydration in the symbiotic association peanut-Bradyrhizobium sp. SRN Agron. 366:318083. doi: 10.5402/2012/318083
Ghawana, S., Paul, A., Kumar, H., Kumar, A., Singh, H., Bhardwaj, P. K., et al. (2011). An RNA isolation system for plant tissues rich in secondary metabolites. BMC Res. Notes 4:85. doi: 10.1186/1756-0500-4-85
Hamilton, J. G., Zangerl, A. R., DeLucia, E. H., and Berenbaum, M. R. (2001). The carbon–nutrient balance hypothesis: its rise and fall. Ecol. Lett. 4, 86–95. doi: 10.1046/j.1461-0248.2001.00192.x
Hare, P., and Cress, W. (1997). Metabolic implications of stress-induced proline accumulation in plants. Plant Growth Regul. 21, 79–102.
Hazra, S., Bhattacharyya, D., and Chattopadhyay, S. (2017). Methyl jasmonate regulates podophyllotoxin accumulation in Podophyllum hexandrum by altering the ROS-responsive podophyllotoxin pathway gene expression additionally through the down regulation of few interfering miRNAs. Front. Plant Sci. 8:164. doi: 10.3389/fpls.2017.00164
Herms, D. A., and Mattson, W. J. (1992). The dilemma of plants: to grow or defend. Q. Rev. Biol. 67, 283–335. doi: 10.1086/417659
Hernandez-Garcia, C. M., and Finer, J. J. (2014). Identification and validation of promoters and cis-acting regulatory elements. Plant Sci. 217, 109–119. doi: 10.1016/j.plantsci.2013.12.007
Jaafar, H. Z., Ibrahim, M. H., Fakri, M., and Farhana, N. (2012). Impact of soil field water capacity on secondary metabolites, phenylalanine ammonia-lyase (PAL), maliondialdehyde (MDA) and photosynthetic responses of Malaysian Kacip Fatimah (Labisia pumila Benth). Molecules 17, 7305–7322. doi: 10.3390/molecules17067305
Jaleel, C. A., Manivannan, P., Sankar, B., Kishorekumar, A., Gopi, R., Somasundaram, R., et al. (2007). Induction of drought stress tolerance by ketoconazole in Catharanthus roseus is mediated by enhanced antioxidant potentials and secondary metabolite accumulation. Colloids Sur. B Biointerfaces 60, 201–206. doi: 10.1016/j.colsurfb.2007.06.010
Jamaux, I., Steinmetz, A., and Belhassen, E. (1997). Looking for molecular and physiological markers of osmotic adjustment in sunflower. New Phytol. 137, 117–127. doi: 10.1046/j.1469-8137.1997.00817.x
Janiak, A., Kwaśniewski, M., and Szarejko, I. (2016). Gene expression regulation in roots under drought. J. Exp. Bot. 67, 1003–1014. doi: 10.1093/jxb/erv512
Jia, X., Jia, X., Li, T., Wang, Y., Sun, X., Huo, L., et al. (2021). MdATG5a induces drought tolerance by improving the antioxidant defenses and promoting starch degradation in apple. Plant Sci. 312, 111052. doi: 10.1016/j.plantsci.2021.111052
Jin, B., Sheng, Z., Muhammad, I., Chen, J., and Yang, H. (2019). Cloning and functional analysis of the promoter of a stress-inducible gene (Zmap) in maize. PLoS One 14:e0211941. doi: 10.1371/journal.pone.0211941
Jogawat, A., Yadav, B., Lakra, N., Singh, A. K., and Narayan, O. P. (2021). Crosstalk between phytohormones and secondary metabolites in the drought stress tolerance of crop plants: a review. Physiol. Plant. 172, 1106–1132. doi: 10.1111/ppl.13328
Joshi, R., Sahoo, K. K., Singh, A. K., Anwar, K., Pundir, P., Gautam, R. K., et al. (2020). Enhancing trehalose biosynthesis improves yield potential in marker-free transgenic rice under drought, saline, and sodic conditions. J. Exp. Bot. 71, 653–668. doi: 10.1093/jxb/erz462
Kala, C. P. (2000). Status and conservation of rare and endangered medicinal plants in the Indian trans-Himalaya. Biol. Conserv. 93, 371–379. doi: 10.1016/s0006-3207(99)00128-7
Kawoosa, T., Gahlan, P., Devi, A. S., and Kumar, S. (2014). The GATA and SORLIP motifs in the 3-hydroxy-3-methylglutaryl-CoA reductase promoter of Picrorhiza kurrooa for the control of light-mediated expression. Funct. Integr. Genomics 14, 191–203. doi: 10.1007/s10142-013-0350-3
Kawoosa, T., Singh, H., Kumar, A., Sharma, S. K., Devi, K., Dutt, S., et al. (2010). Light and temperature regulated terpene biosynthesis: hepatoprotective monoterpene picroside accumulation in Picrorhiza kurrooa. Funct. Integr. Genomics 10, 393–404. doi: 10.1007/s10142-009-0152-9
Keyvan, S. (2010). The effects of drought stress on yield, relative water content, proline, soluble carbohydrates and chlorophyll of bread wheat cultivars. J. Anim. Plant Sci. 8, 1051–1060.
Kharkwal, A. C., Kushwaha, R., Prakash, O., Ogra, R. K., Bhattacharya, A., Nagar, P. K., et al. (2008). An efficient method of propagation of Podophyllum hexandrum: an endangered medicinal plant of the Western Himalayas under ex situ conditions. J. Nat. Med. 62, 211–216. doi: 10.1007/s11418-007-0217-9
Kitchlu, S., Ram, G., Koul, S., Koul, K., Gupta, K. K., and Ahuja, A. (2011). Podophyllum lignans array of Podophyllum hexandrum Royle populations from semi-desert alpine region of Zanskar valley in Himalayas. Ind. Crops Prod. 33, 584–587. doi: 10.1016/j.indcrop.2010.12.010
Klok, E. J., Wilson, I. W., Wilson, D., Chapman, S. C., Ewing, R. M., Somerville, S. C., et al. (2002). Expression profile analysis of the low-oxygen response in Arabidopsis root cultures. Plant Cell 14, 2481–2494. doi: 10.1105/tpc.004747
Kumari, A., Singh, D., and Kumar, S. (2017). Biotechnological interventions for harnessing podophyllotoxin from plant and fungal species: current status, challenges, and opportunities for its commercialization. Crit. Rev. Biotechnol. 37, 739–753. doi: 10.1080/07388551.2016.1228597
Kumari, A., Singh, H. R., Jha, A., Swarnkar, M. K., Shankar, R., and Kumar, S. (2014). Transcriptome sequencing of rhizome tissue of Sinopodophyllum hexandrum at two temperatures. BMC Genomics 15:871. doi: 10.1186/1471-2164-15-871
Laxa, M., Liebthal, M., Telman, W., Chibani, K., and Dietz, K. J. (2019). The role of the plant antioxidant system in drought tolerance. Antioxidants 8:94. doi: 10.3390/antiox8040094
Le Roy, J., Huss, B., Creach, A., Hawkins, S., and Neutelings, G. (2016). Glycosylation is a major regulator of phenylpropanoid availability and biological activity in plants. Front. Plant Sci. 7:735. doi: 10.3389/fpls.2016.00735
Li, M., Lv, M., Yang, D., Wei, J., Xing, H., and Paré, P. W. (2020). Temperature-regulated anatomical and gene-expression changes in Sinopodophyllum hexandrum seedlings. Ind. Crops Prod. 152:112479. doi: 10.1016/j.indcrop.2020.112479
Liu, F., Xie, L., Yao, Z., Zhou, Y., Zhou, W., Wang, J., et al. (2019). Caragana korshinskii phenylalanine ammonialyase is up-regulated in the phenylpropanoid biosynthesis pathway in response to drought stress. Biotechnol. Biotechnol. Equip. 33, 842–854. doi: 10.1080/13102818.2019.1623718
Liu, J., Osbourn, A., and Ma, P. (2015). MYB transcription factors as regulators of phenylpropanoid metabolism in plants. Mol. Plant 8, 689–708. doi: 10.1016/j.molp.2015.03.012
Liu, W., Yin, D., Liu, J., and Li, N. (2014). Genetic diversity and structure of Sinopodophyllum hexandrum (Royle) Ying in the Qinling Mountains, China. PLoS One 9:e110500. doi: 10.1371/journal.pone.0110500
Lv, M., Zhang, Y., Wang, F., Zhang, S., and Xu, H. (2020). Non-food renewable and bioactive forest products for pest management: valuation of agricultural properties of podophyllotoxin analogs derived from Podophyllum hexandrum as botanical pesticides. Ind. Crops Prod. 153:112608. doi: 10.1016/j.indcrop.2020.112608
McCue, K. F., and Hanson, A. D. (1990). Drought and salt tolerance: towards understanding and application. Trends Biotechnol. 8, 358–362. doi: 10.1016/0167-7799(90)90225-m
Morgan, J. M. (1984). Osmoregulation and water stress in higher plants. Annu. Rev. Plant Physiol. 35, 299–319. doi: 10.1146/annurev.pp.35.060184.001503
Nelson, D. E., Rammesmayer, G., and Bohnert, H. J. (1998). Regulation of cell-specific inositol metabolism and transport in plant salinity tolerance. Plant Cell 10, 753–764. doi: 10.1105/tpc.10.5.753
Nguyen, K. H., Van Ha, C., Nishiyama, R., Watanabe, Y., Leyva-González, M. A., Fujita, Y., et al. (2016). Arabidopsis type B cytokinin response regulators ARR1, ARR10, and ARR12 negatively regulate plant responses to drought. Proc. Natl. Acad. Sci. U.S.A. 113, 3090–3095. doi: 10.1073/pnas.1600399113
NOAA (2020a). National Centers for Environmental Information, State of the Climate: Global Climate Report for Annual 2020. Available online at: https://www.ncdc.noaa.gov/sotc/global/202013 (accessed March 15, 2021).
NOAA (2020b). Climate Change: Atmospheric Carbon Dioxide. Available online at: https://www.ncdc.noaa.gov/sotc/global/202013 (accessed October 7, 2021).
Nogués, S., Allen, D. J., Morison, J. I., and Baker, N. R. (1998). Ultraviolet-B radiation effects on water relations, leaf development, and photosynthesis in droughted pea plants. Plant Physiol. 117, 173–181. doi: 10.1104/pp.117.1.173
Nowak, M., Kleinwaechter, M., Manderscheid, R., Weigel, H. J., and Selmar, D. (2010). Drought stress increases the accumulation of monoterpenes in sage (Salvia officinalis), an effect that is compensated by elevated carbon dioxide concentration. J. Appl. Bot. Food Qual. 83, 133–136.
Osmolovskaya, N., Shumilina, J., Kim, A., Didio, A., Grishina, T., Bilova, T., et al. (2018). Methodology of drought stress research: experimental setup and physiological characterization. Int. J. Mol. Sci. 19:4089. doi: 10.3390/ijms19124089
Pattanagul, W., and Madore, M. A. (1999). Water deficit effects on raffinose family oligosaccharide metabolism in Coleus. Plant Physiol. 121, 987–993. doi: 10.1104/pp.121.3.987
Peñuelas, J., and Estiarte, M. (1998). Can elevated CO2 affect secondary metabolism and ecosystem function? Trends Ecol. Evol. 13, 20–24. doi: 10.1016/s0169-5347(97)01235-4
Pereira, A. (2016). Plant abiotic stress challenges from the changing environment. Front. Plant Sci. 7:1123. doi: 10.3389/fpls.2016.01123
Pfaffl, W. (2012). “Quantification strategies in real-time polymerase chain reaction,” in Quantitative Real-Time PCR in Applied Microbiology ed. M. Filion (Norfolk, UK: Caister Academic press), 53–61.
Pilkington, S. M., Encke, B., Krohn, N., Höhne, M., Stitt, M., and Pyl, E. T. (2015). Starch degradation and carbon demand. Plant Cell Environ. 38, 157–171. doi: 10.1111/pce.12381
Rao, N. S., Bhatt, R. M., and Sadashiva, A. T. (2000). Tolerance to water stress in tomato cultivars. Photosynthetica 38, 465–467. doi: 10.1023/a:1010902427231
Rather, M. A., and Amin, S. (2016). A comprehensive review on the phytochemical and pharmacological aspects of Podophyllum hexandrum: a high value medicinal plant. Adv. Biomed. Pharm. 3, 216–226. doi: 10.19046/abp.v03i04.06
Ritchie, S. W., Nguyen, H. T., and Holaday, A. S. (1990). Leaf water content and gas-exchange parameters of two wheat genotypes differing in drought resistance. Crop Sci. 30, 105–111. doi: 10.2135/cropsci1990.0011183x003000010025x
Sanchita, Singh, R., Mishra, A., Dhawan, S. S., Shirke, P. A., Gupta, M. M., and Sharma, A. (2015). Physiological performance, secondary metabolite and expression profiling of genes associated with drought tolerance in Withania somnifera. Protoplasma 252, 1439–1450. doi: 10.1007/s00709-015-0771-z
Savoi, S., Wong, D. C., Arapitsas, P., Miculan, M., Bucchetti, B., Peterlunger, E., et al. (2016). Transcriptome and metabolite profiling reveals that prolonged drought modulates the phenylpropanoid and terpenoid pathway in white grapes (Vitis vinifera L.). BMC Plant Biol. 16:67. doi: 10.1186/s12870-016-0760-1
Sengupta, S., Mangu, V., Sanchez, L., Bedre, R., Joshi, R., Rajasekaran, K., et al. (2019). An actin-depolymerizing factor from the halophyte smooth cordgrass, Spartina alterniflora (SaADF2), is superior to its rice homolog (OsADF2) in conferring drought and salt tolerance when constitutively overexpressed in rice. Plant Biotechnol. J. 17, 188–205. doi: 10.1111/pbi.12957
Shah, N. C. (2006). Podophyllum hexandrum and its conservation status in India. Med. Plant Conserv. 12, 42–47.
Sheffer, K. M., Watschke, T. L., and Duich, J. M. (1979). Carbohydrate Sampling in Kentucky Bluegrass Turf1. Agron. J. 71, 301–304. doi: 10.2134/agronj1979.00021962007100020020x
Simpson, S. D., Nakashima, K., Narusaka, Y., Seki, M., Shinozaki, K., and Yamaguchi-Shinozaki, K. (2003). Two different novel cis-acting elements of erd1, a clpA homologous Arabidopsis gene function in induction by dehydration stress and dark-induced senescence. Plant J. 33, 259–270. doi: 10.1046/j.1365-313x.2003.01624.x
Singh, A., and Purohit, A. N. (1998). Light and temperature effects on physiological reactions on alpine and temperate populations of Podophyllum hexandrum Royle. J. Herbs Spices Med. Plants 5, 57–66. doi: 10.1300/j044v05n02_08
Singh, D., and Laxmi, A. (2015). Transcriptional regulation of drought response: a tortuous network of transcriptional factors. Front. Plant Sci. 6:895. doi: 10.3389/fpls.2015.00895
Soltys-Kalina, D., Plich, J., Strzelczyk-Żyta, D., Śliwka, J., and Marczewski, W. (2016). The effect of drought stress on the leaf relative water content and tuber yield of a half-sib family of ‘Katahdin”Katahdin’-derived potato cultivars. Breed. Sci. 66, 328–331. doi: 10.1270/jsbbs.66.328
Sreenivasulu, Y., Chanda, S. K., and Ahuja, P. S. (2009). Endosperm delays seed germination in Podophyllum hexandrum Royle-an important medicinal herb. Seed Sci. Technol. 37, 10–16. doi: 10.15258/sst.2009.37.1.02
Szabados, L., and Savoure, A. (2010). Proline: a multifunctional amino acid. Trends Plant Sci. 15, 89–97. doi: 10.1016/j.tplants.2009.11.009
Tamagnone, L., Merida, A., Parr, A., Mackay, S., Culianez-Macia, F. A., Roberts, K., et al. (1998). The AmMYB308 and AmMYB330 transcription factors from Antirrhinum regulate phenylpropanoid and lignin biosynthesis in transgenic tobacco. Plant Cell 10, 135–154. doi: 10.1105/tpc.10.2.135
Tewari, V. P., Verma, R. K., and Von Gadow, K. (2017). Climate change effects in the Western Himalayan ecosystems of India: evidence and strategies. For. Ecosyst. 4, 1–9.
Turtola, S., Manninen, A. M., Rikala, R., and Kainulainen, P. (2003). Drought stress alters the concentration of wood terpenoids in Scots pine and Norway spruce seedlings. J. Chem. Ecol. 29, 1981–1995. doi: 10.1023/a:1025674116183
Venkataraman, K. (2009). India’s Biodiversity Act 2002 and its role in conservation. Trop. Ecol. 50, 23–30.
Wang, J. J., Zhi, X. Y., Yu, X., and Xu, H. (2013). Synthesis and insecticidal activity of new deoxypodophyllotoxin-based phenazine analogues against Mythimna separata Walker. J. Agric. Food Chem. 61, 6336–6343. doi: 10.1021/jf4011033
Wungrampha, S., Joshi, R., Rathore, R. S., Singla-Pareek, S. L., and Pareek, A. (2019). CO2 uptake and chlorophyll a fluorescence of Suaeda fruticosa grown under diurnal rhythm and after transfer to continuous dark. Photosyn. Res. 142, 211–227. doi: 10.1007/s11120-019-00659-0
Xu, C., McDowell, N. G., Fisher, R. A., Wei, L., Sevanto, S., Christoffersen, B. O., et al. (2019). Increasing impacts of extreme droughts on vegetation productivity under climate change. Nat. Clim. Change 9, 948–953.
Xu, Z., Wang, M., Guo, Z., Zhu, X., and Xia, Z. (2019). Identification of a 119-bp promoter of the maize sulfite oxidase gene (ZmSO) that confers high-level gene expression and ABA or drought inducibility in transgenic plants. Int. J. Mol. Sci. 20:3326. doi: 10.3390/ijms20133326
Keywords: adaptation, cis-element, podophyllotoxin (PTOX), secondary metabolism, water-deficit stress
Citation: Kumari A, Dogra V, Joshi R and Kumar S (2022) Stress-Responsive cis-Regulatory Elements Underline Podophyllotoxin Biosynthesis and Better Performance of Sinopodophyllum hexandrum Under Water Deficit Conditions. Front. Plant Sci. 12:751846. doi: 10.3389/fpls.2021.751846
Received: 02 August 2021; Accepted: 09 November 2021;
Published: 04 January 2022.
Edited by:
Jai Rohila, Agricultural Research Service, United States Department of Agriculture (USDA), United StatesReviewed by:
Dipto Bhattacharyya, Bennett University, IndiaSiddhartha Kanrar, Agricultural Research Service, United States Department of Agriculture (USDA), United States
Copyright © 2022 Kumari, Dogra, Joshi and Kumar. This is an open-access article distributed under the terms of the Creative Commons Attribution License (CC BY). The use, distribution or reproduction in other forums is permitted, provided the original author(s) and the copyright owner(s) are credited and that the original publication in this journal is cited, in accordance with accepted academic practice. No use, distribution or reproduction is permitted which does not comply with these terms.
*Correspondence: Sanjay Kumar, sanjayplp1@gmail.com, sanjaykumar@ihbt.res.in
†Present address: Anita Kumari, Department of Ornamental Plants and Agricultural Biotechnology, Agricultural Research Organization, Volcani Center, Rishon LeZion, Israel