Prenatal Immunization to Prevent Viral Disease Outcomes During Pregnancy and Early Life
- 1Division of Infectious Diseases, Department of Pediatrics, Weill Cornell Medicine, New York, NY, United States
- 2Duke Human Vaccine Institute, Duke University Medical Center, Durham, NC, United States
- 3Department of Pediatrics, Duke University Medical Center, Durham, NC, United States
- 4Duke University School of Medicine, Duke University, Durham, NC, United States
- 5Department of Surgery, Duke University Medical Center, Durham, NC, United States
Pregnancy significantly elevates the risk of developing severe viral diseases, which can have a detrimental effect on fetal development and increases maternal mortality. In addition, certain viruses can be transmitted vertically from mother to babies, either in utero, during delivery, or postnatally during breastfeeding, resulting in congenital or neonatal diseases and associated sequelae. While neonates are highly susceptible to viral infections and severe disease outcomes, due to the immaturity of their developing immune system, virus-specific maternal antibodies transferred either trans-placentally or via breast milk provide protection to infants against intestinal, respiratory, or systemic infections, during the first months of life. Thus, maternal prenatal immunization is important not only to protect pregnant women from viral diseases, but also to prevent infection and/or improve disease outcomes for the fetuses and neonates via passively transferred antibodies. In this review, we discuss the protective role of maternal antibodies against three categories of viruses: (i) viruses that cause severe maternal disease outcomes with mainly indirect consequences to the fetus (e.g. SARS-CoV-2, influenza, DENV, filovirus), (ii) those that are vertically transmitted from mother to their infants and cause congenital diseases (e.g. HIV, ZIKV and CMV), and (iii) those that cause elevated disease severity among neonates and infants postnatally (e.g. RSV, Rotavirus, Norovirus, HSV and HBV). Furthermore, we review relevant pre-clinical animal models that can be employed to develop novel immunization strategies against these viruses to enhance protection of pregnant women and their babies.
Introduction
Pregnancy is a period of increased risk for a variety of viral infections and associated disease severity (1), due to the unique biological, physiological, and immunological alterations in mothers in order to tolerate the fetus (2). Viral infection in pregnant women often results in severe or fatal complications such as intrauterine growth restriction, preterm birth, stillbirth, or miscarriage (1). For instance, the currently ongoing Coronavirus disease 2019 (COVID-19) pandemic resulted in a higher risk of severe maternal infections, hospitalization, maternal mortality, preterm birth, and severe neonatal and perinatal morbidity index, compared to uninfected pregnant women (3). Additionally, viral infection during pregnancy results in immune inflammation at the placental-fetal interface, which might interfere with fetal development (4). Moreover, several viruses such as cytomegalovirus (CMV) and Zika virus (ZIKV) can cross the placenta and infect the fetus, resulting in severe congenital diseases in newborns (4), whereas others such as HIV and hepatitis B (HBV) can lead to chronic infection. Infants during their first few months of life represents another population at increased risk for poor virus-associated disease outcomes (5). For instance, a prospective study performed between 2015 and 2016 at US pediatric hospital sites indicated that hospitalization rate due to RSV infection was highest in infants <6 months of age (6). Despite the elevated risk of morbidity and mortality among pregnant women, their unborn fetus, and infants, limited preventative or therapeutic strategies are available for these populations. Notably, vaccination is the most effective strategy to prevent virus-related disease complications during pregnancy; Yet vaccines are not available against several viruses associated with poor maternal and/or fetal outcomes. Importantly, prenatal active immunization does not only protect pregnant women from the deadly consequences of infection by generating maternal immunity but can also protect their offspring via transfer of the protective maternal immunity, trans-placentally or via breast milk to the fetus and the newborns, respectively. One of the main limitations of prenatal vaccination research is exclusion of pregnant women from vaccine safety and efficacy trials, which limits or delays the availability of vaccines to pregnant women in need. Moreover, because pregnancy is associated with an altered immunological landscape, some vaccines such as live attenuated vaccines are contraindicated during this period (7). Hence, there remains a need for developing vaccine formulations that are safe for pregnant women and tailored to the unique gestational immune landscape.
Here we review the current state of prenatal immunization to prevent viral disease outcomes during pregnancy and early life. We have categorized viruses into three categories: (i) viruses that cause heightened maternal diseases severity with mainly indirect consequences to the fetus (SARS-CoV-2, influenza, DENV, filoviruses), (ii) viruses that are vertically transmitted from mother to their infants and cause congenital abnormalities (HIV, ZIKV and CMV) and (iii) viruses that cause elevated disease severity among neonates and infants (Rotavirus, Norovirus, RSV, HSV and HBV). We focus on a few key representative viruses (Figure 1), and discuss the associated complications, with special emphasis on pregnant women and infants. Additionally, for each of the viruses, we highlight recent advances and ongoing studies in development of prenatal immunization strategies, particularly focusing on the protective roles of trans-placentally and postnatally transmitted maternal antibodies to improve infant disease outcomes. Finally, we review preclinical animal models that have been developed to date to study viral pathogenesis and clinical course of infection. Such knowledge can be utilized to improve our understanding of the interactions of the virus with pregnant women, their unborn fetus, and infants, which will ultimately guide development of effective prenatal vaccination strategies. We acknowledge that additional categories of viruses may cause elevated disease severity in both pregnant mothers and their infants yet are rarely associated with congenital infections. An example of such a virus is varicella zoster virus (VZV). These additional categories of viruses were excluded to keep the scope of the review focused. Of note, throughout our review, we refer to congenital transmission as viral transmission in utero, perinatal transmission as transmission during delivery and postnatal transmission as transmission via breastfeeding.
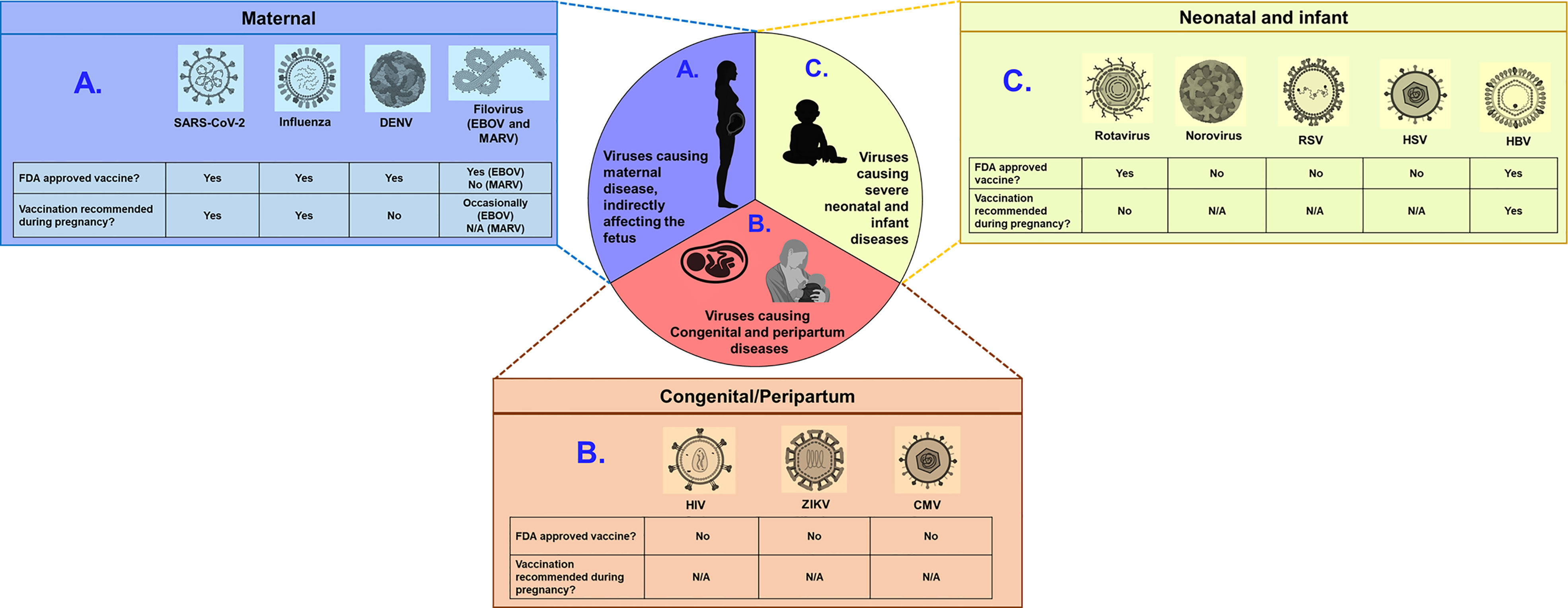
Figure 1 Examples of viruses with the potential to cause severe disease in pregnant women, fetuses, and infants. Viruses can be broadly categorized into three groups: (A) those causing severe maternal disease, indirectly affecting the fetus (SARS-CoV-2, Influenza, DENV and Filovirus) (B) those vertically transmitted from mothers to fetus causing congenital defects (HIV, ZIKV, and CMV) and (C) those causing severe disease outcomes in infants and neonates (rotavirus, norovirus, RSV, HSV and HBV). Schematic depicts whether FDA approved vaccine is available and whether such vaccines are recommended for pregnant women, for each virus. N/A indicates not applicable, due to unavailability of approved vaccines. Of note, EBOV vaccine is occasionally recommended for pregnant women, when benefit outweights risks. Figure constructed using Biorender.
Viruses Causing Maternal Disease, Indirectly Affecting the Fetus
Alterations in hormonal levels such as estrogen, progesterone and glucocorticoids and an anti-inflammatory immune response associated with pregnancy impact maternal immune response to pathogens (2). As a consequence, pregnant women are either more vulnerable to viral infections and/or have increased risk of severe viral diseases. Some of these adverse outcomes of viral infection in pregnancy are due to the abnormal release of pro-inflammatory cytokines in the infected mothers that disrupt fetal development (4, 8). Maternal infection with viruses can also lead to psychological anxiety and stress in the pregnant women (9), that might influence fetal neurodevelopment (10, 11). Hence, maternal immunization before conception or during pregnancy is beneficial to generate antibody responses that will protect the mother and their fetus from adverse virus-associated disease outcomes.
Severe Acute Respiratory Syndrome-Coronavirus-2 (SARS-CoV-2)
SARS-CoV-2, the causative agent of COVID-19, is a positive-sense single-stranded RNA virus, belonging to the Coronaviridae family (12–14). Since its emergence in 2019, this virus has afflicted over 250 million people and resulted in >5 million deaths (15), worldwide. The primary mode of transmission of this virus is via exposure to infectious respiratory droplets (16). Since January 2020, the US has reported over 150,000 cases of COVID-19 among pregnant women, of which >25,000 resulted in hospitalizations and >250 resulted in death (17). A higher risk of SARS-CoV-2 infection-associated maternal mortality and hospitalization along with neonatal complication was also observed in a multinational cohort-based study, in which pregnant women were enrolled from 18 different countries (18).
Clinical Presentation and Associated Complications
SARS-CoV-2 infection during pregnancy results in increased mortality and adverse pregnancy outcomes. A study conducted in Washington State estimated that 10% of SARS-CoV-2-infected pregnant women required hospitalization, whereas for non-pregnant adults this number was only 2.8% (19). In addition, pregnant women diagnosed with COVID-19 are at higher risk for preeclampsia/eclampsia, severe infections, intensive care unit admission, maternal mortality, preterm birth, still birth, and severe neonatal and perinatal morbidity index, compared to uninfected pregnant women (3, 20). Current data indicate that mother-to-child transmission (MTCT) rarely occurs. Notably, in a recent study, SARS-CoV-2 RNA was only detected in the umbilical cord plasma and placental tissue of 2 out of 31 infants born to SARS-CoV-2 positive mothers (21), indicating limited replication capacity of the virus in the in-utero environment. This phenomenon may be explained by the unfavorable localization and scarcity of ACE2 and TMPRSS2 receptors in the placenta (22, 23). Similarly, only a few studies have reported the detection of SARS-CoV-2 RNA in the breast milk of infected mothers (24, 25). Zhu et al, reported an association between the presence of SARS-CoV-2 RNA in the breast milk and mild symptoms in infants (25), but this association was not observed in other studies (26). Importantly, the detection of viral RNA does not necessarily indicate the presence of replication competent SARS-CoV-2. In fact, to date, infectious SARS-CoV-2 particles have not been isolated from breast milk (27), highlighting the need for further investigations to confirm if postnatal MTCT of the virus via breast milk can occur.
Maternally Acquired Antibodies and Implications for Prenatal Immunization
Several studies have demonstrated that SARS-CoV-2-specific antibodies generated in the infected women during pregnancy are transferred to the neonates (28, 29). Flannery et al, reported that sera of 72 out of 83 infants born to SARS-CoV-2 IgG and/or IgM positive pregnant women had detectable IgG responses, and SARS-CoV-2 IgG concentrations in maternal sera correlated with those of cord blood (29). The timing of maternal infection may critically impact the efficiency of placental antibody transfer, as pregnant women with recent or ongoing infection have been reported to have overall reduced transfer of SARS-CoV-2-specific IgG against the spike, receptor binding domain, and nucleocapsid antigens compared to SARS-CoV-2 recovered individuals (30). This suggests that the timing of maternal vaccination could profoundly influence the ability to achieve neonatal protection (31, 32). SARS-CoV-2-specific maternal antibodies could also be transferred to babies through breast milk. In a recent study, 84% of SARS-CoV-2 positive and COVID-recovered mothers had IgG, IgA or IgM against the receptor binding domain in breast milk, with 53% being positive for all three isotypes (24). Conti et al, reported significantly higher levels of SARS-CoV-2 spike-specific IgA in the saliva of infants born to COVID positive women who were exclusively breast-fed compared to those that were formula-fed (28). Notably, both IgG and IgA responses in breast milk exhibited neutralization activity against live SARS-CoV-2 virus (33), indicating that immunization of pregnant women could induce infant protection via the transfer maternally acquired antibodies.
After initial hesitation to recommend SARS-CoV-2 immunization in pregnant women due to lack of safety data, both the CDC and the WHO now recommend the use of the COVID-19 vaccine during pregnancy, with several studies reporting its safety (34, 35). Similar to infected women, women who received SARS CoV-2 vaccine during pregnancy transferred virus-specific antibodies to their babies (36, 37). In a recent study, Low et al. demonstrated that two doses of a SARS-CoV-2 mRNA vaccine in lactating mother was able to elicit virus-specific IgG and IgA antibodies in the breastmilk (38). Antibody titers (36, 38) as well as durability (37) of the maternally transferred antibodies are typically higher in infants born to vaccinated mothers compared to infants born to unvaccinated infected mothers, suggesting that humoral responses transferred from mothers following prenatal vaccination could provide a better protection of infants than those obtained from natural infection, although larger clinical studies will be needed to verify such a hypothesis. Moreover, prenatal vaccination has the advantage of protecting the mother from severe disease consequences (39), thereby preventing development of adverse maternal-fetal consequences. For instance, fully vaccinated pregnant women infected with SARS-CoV-2 during the Omicron wave had lower disease severity, compared to unvaccinated women (40). Importantly, since the timing of maternal vaccination may impact the efficiency of IgG transfer, the prenatal immunization schedule must be carefully tailored to ensure optimal protection of both mother and infant (41). In addition, to design a protective prenatal immunization regimen, it will be important to understand the kinetics and durability of maternally transferred antibodies and their possible interference with the developing immune system of infants.
Animal Models to Guide Prenatal Vaccination Research
A variety of animal models including humanized mice, hamsters, ferrets, pigs and non-human primates (NHP) are used to study SARS-CoV-2 infection, pathogenesis and immune responses. While all of these species express ACE-2 receptors and can be infected by the virus (42), only a few of these animal models are able to recapitulate human disease. Golden Syrian hamsters are considered as valuable models for therapeutic research due to their ease of handling, low cost and similar lung pathological phenotypes post-SARS-CoV-2 infection (42, 43). Vaccinology research however has shown particular interest in using rhesus macaques (RMs), owing to their similarity to human anatomy, physiology and immunology and ability to generate neutralizing antibody responses (44). Moreover, like humans, SARS-CoV-2 replicates in the respiratory tract of RMs and viral infection results in pulmonary infiltrates and a shedding pattern similar to mild to moderate human infections (45, 46). To date, there is no published animal model of SARS-CoV-2 infection during gestation or preclinical animal studies testing the safety and efficacy of SARS-CoV-2 vaccines during pregnancy. The development of such animal models will be critical to design and evaluate prenatal immunization regimens that will confer maximum protection to the mother and their neonates against SARS-CoV-2 infection.
Influenza Virus
Influenza virus is a member of the Orthomyxoviridae family, with a segmented, negative-stranded RNA genome (14, 47). This virus spreads via respiratory droplets and approximately 8% of the US population get sick with the seasonal virus each year (48). In 2018-2019, 29 million confirmed influenza cases were reported in the US, of which 380,000 were hospitalized and 28,000 died (49). The virus is categorized into three main types: influenza A virus (IAV), influenza B virus (IBV) and influenza C virus (ICV) (47), of which IAVs are responsible for most seasonal cases and outbreaks of influenza.
Clinical Presentation and Associated Complications
Pregnant women are highly vulnerable to influenza virus infection and virus-associated severe disease outcomes, resulting in increased rates of mortality (50). During the 2009 H1N1 pandemic, pregnant women accounted for 5% of deaths, while representing less than 1% population (51). While the risk of mother-to-child transplacental transmission of influenza is extremely rare (52), maternal infection during pregnancy is associated with adverse maternal-fetal outcomes such as preterm delivery, spontaneous abortion, low birth weight and fetal death (50). In addition, maternal influenza virus infection disrupts the cytokine and hormonal signaling pathways responding to respiratory pathogens, compromising lung and placental architecture, and impacting the health of both mothers and their offspring (53). Maternal influenza infection may also have an impact on the neonatal immune response. Notably, offspring of mice infected with influenza during pregnancy demonstrated significant challenges in clearing secondary respiratory infections compared to offspring of healthy dams and it has been hypothesized that reduced levels of natural killer and B cells in the pups of influenza-infected dams might contribute to the increased vulnerability to infection (54). Whether infants of women who experience influenza infection during pregnancy demonstrate a similar increased susceptibility to respiratory infections remains unclear.
Maternally Acquired Antibodies and Implications for Prenatal Immunization
To prevent maternal influenza infection and reduce the risk of complications during pregnancy, the CDC recommends that all pregnant women receive the inactivated influenza vaccine (55). The safety of maternal vaccination has been widely demonstrated (56). Vaccination during pregnancy reduced the risk of influenza-associated acute respiratory infection by one-half, lowering the risk of being hospitalized with virus-associated disease by 40 percent (57).
Maternal Influenza vaccination also provides passive immune protection to the infants, with virus-specific IgG transferred through the placenta and virus-specific IgA transferred through breastfeeding (58, 59). These antibodies are particularly important for protecting infants during their first few months of life, as influenza vaccination during the first 6 months of life is not advised. In fact, maternal vaccination during pregnancy reduced infant hospitalization due to influenza infection by up to 91% (60–63). In addition, cord blood antigen-specific hemagglutination inhibition (HAI) titer was found to correlate with protection from IBV during the first 2 months of life and higher titers were associated with reduction in the risk of infections (64). In fact, trans-placentally transferred IAV-specific IgG antibodies in cord blood neutralized IAV in vitro (65). Moreover, anti-influenza IgA antibodies transferred to infants from their vaccinated mothers during breastfeeding significantly decreased the number of respiratory illnesses during early life (58).
Animal Models to Guide Prenatal Vaccination Research
Unfortunately, to date the efficacy of seasonal influenza vaccines is fairly limited, usually around 40–60%, even dropping to as low as 20% in certain seasons (66). Thus, investigations of novel vaccination platforms and universal influenza immunization strategies that will confer protection against multiple influenza strains are warranted. Potential vaccination strategies will need to demonstrate efficacy, immunogenicity and safety in pre-clinical animal models, before proceeding to human clinical trials. To date, several animal models have been used for influenza vaccinology research, including mice and ferrets. Although NHPs are not natural hosts for influenza viruses, they can be experimentally infected with human isolates and exhibit disease symptoms, as observed in humans (67). To specifically study mother-to-child transfer of antibodies post-immunization, mice models have been mostly employed (68). While primary findings from rodent models can provide the proof of concept that maternal antibodies can confer protection to their offspring, the differences in placentation and mechanisms of antibody transfer between rodents and humans, must be taken into consideration and there remains a need for more physiologically relevant models.
Dengue Virus (DENV)
DENV is a positive-sense single stranded RNA virus belonging to the Flaviviridae family (14, 69). Transmission of the four similar but antigenically distinct DENV serotypes (DENV-1, DENV-2, DENV-3, DENV-4) occur primarily through the bite of the female Aedes mosquito in tropical and subtropical countries, but can also occur via blood transfusions and vertically from mother to their infants (70–75). Each year ~400 million people are infected with DENV, of which 40,000 die from severe DENV disease. All four serotypes of DENV can co-circulate during an outbreak (76). In addition, co-infection between DENV serotypes and other flaviviruses such as ZIKV has also been reported (77). DENV infection is of high concern for pregnant women due to virus-associated severe maternal and fetal outcomes (78). Pregnant women are approximately 3.5 times more susceptible to development of severe DENV-disease and the mortality rate of DENV-infected pregnant women is also significantly higher, compared to non-pregnant individuals (79, 80).
Clinical Presentation and Associated Complications
DENV is endemic in over 100 countries including central and south America, the middle east, Asia, and the Pacific Islands (81). DENV infection and re-infection was ranked the second most severe vector born disease worldwide (82) as it can cause varying levels of disease, ranging from asymptomatic infection to dengue hemorrhagic fever, dengue shock syndrome and death (81, 83). Viral infection during pregnancy is associated with premature birth, fetal death, low birth weight, acute fetal distress, and hemorrhage (71, 73, 84, 85). DENV transmission from mothers to their infants have been reported. In a prospective study, DENV transmission to their infants was reported in 22.7% women infected during the 2012-2013 epidemic in French Guiana (74). However, the pathogenesis of DENV infection in neonates and infants is poorly understood. DENV transmission from mother to child often occurs during delivery, although, in-utero transmission of DENV through the placenta has been observed (74). Interestingly, Vats et al., demonstrated that umbilical cord blood cells are highly permissive to DENV infection, and hence were thought of a site of viral amplification before vertical transmission (86). To date, although DENV virus has been found in breastmilk (73), the virus has been rarely documented to be transferred postnatally during breastfeeding. Hence, CDC still encourages breastfeeding for all mothers even if those from DENV endemic areas.
Maternally Acquired Antibodies and Implications for Prenatal Immunization
Maternal DENV-specific IgG antibodies are efficiently transferred across the placenta to the fetus and persists in the infants during the first year of their life (87). Presence of maternally acquired DENV-specific antibodies has been associated with low prevalence of symptomatic DENV infection in infants <3-4 months of age (88). Presence of sub-neutralizing levels of antibodies generated during primary DENV infection in adults has been suggested to cause antibody-dependent enhancement (ADE) during a subsequent DENV exposure, leading to severe disease outcomes (89, 90). In neonates and infants, ADE is especially relevant due to presence of trans-placentally transferred maternal antibodies in the first year of their life. Although the pathogenesis of DENV infection in infants is poorly understood, the presence of maternally derived sub neutralizing levels of anti-DENV antibodies were postulated to be a critical risk factor for severe DENV disease outcomes in infants (91). A study conducted with 75 Vietnamese infants with primary DENV infection indicated that infants experienced dengue hemorrhagic fever when maternally derived neutralizing antibody titers have declined (92). Additionally, Ribeiro et al. reported DENV in fetal tissues of pregnant women diagnosed with DENV, when a spectrum of clinical presentations ranging from asymptomatic to dengue hemorrhagic fever and dengue shock syndrome was observed in the newborn. However, whether sub-neutralization levels of maternally derived anti-DENV serotypes enhanced the risk of newborn disease was not defined (93). This finding highlights that maternal immunization against DENV, followed by boosting during the first year of life might protect mothers, their fetus and their infants from severe consequences of DENV-associated diseases but also urges for caution as sub-optimal vaccination may promote ADE. To date, a live attenuated tetravalent vaccine (Dengvaxia®) is licensed for use in dengue endemic regions. However, the use of this vaccine is contraindicated during pregnancy, although accidental administration in pregnant women was not associated with adverse outcomes (94). Hence, mother-infant pre-clinical models to study DENV pathogenesis, maternal antibody associated ADE and protective efficacy of trans-placentally acquired antibodies will be instrumental for development of safe and protective vaccine strategies targeted to this population.
Animal Models to Guide Prenatal Vaccination Research
Development of a suitable DENV pathogenesis model has been challenging due to the poor replication competence of clinical DENV strains in animals. To study peripheral replication of DENV, IFNα/β and γ deficient mice (AG129) have been used (95). Furthermore, mouse-adapted DENV strains were used to identify antiviral therapeutics for DENV replication. To mimic human dengue like clinical symptoms, humanized mice were generated by engrafting human hematopoietic progenitors in immunodeficient mice (96). Efficacy of DENV vaccine candidates has been tested in both immunocompetent and immunocompromised mice models (95). NHPs such as RMs intravenously infected with DENV clinical isolates resulted in hemorrhagic manifestations as observed in humans (97). Even though NHPs do not exactly recapitulate the clinical manifestations of human DENV infection, they develop comparable anti-DENV antibody kinetics to those observed in humans (98). More recently, Tree shrews infected with DENV clinical isolates resembled DENV disease outcomes as observed in humans. Tree Shrew infection model also resulted in development of neutralizing antibodies (99). While maternal immunization for DENV infection is crucial, testing the safety and efficacy of such vaccines are challenging due to lack of reliable animal models that can results in high viremia and mimic DENV clinical manifestation as observed in humans. Hence, there is an urgent need to develop such pregnant DENV transmission animal models, perhaps by utilizing the pregnant infection models already developed for other flavivirus infections, such as ZIKV, described below. Importantly, the co-circulation of different DENV strains and co-infection with other flaviviruses must be considered while developing these models.
Filoviruses
Filoviruses are negative-sense single-stranded RNA viruses belonging to the Filoviridae family (14, 100). The majority of Filovirus-associated pregnancy complications are caused by Ebolavirus (EBOV) and Marburg virus (MARV), which will therefore be the focus of this review. EBOV and MARV-associated diseases are characterized by fever, malaise, body aches, diarrhea, and severe cases of hemorrhagic fever, petechiaeand death (101). EBOV and MARV are transmitted through contact with contaminated body fluids. These viruses have been detected in blood, saliva, amniotic fluids, and seminal fluids (102). Outbreaks of EBOV or MARV are sporadic and range from small scale (<100 cases) to large scale (>200 cases), the largest to date being the West African outbreak which caused over 28,000 cases of Ebola virus disease in 2014-2016 (103).
Clinical Presentation and Associated Complications
EBOV and MARV associated febrile hemorrhagic disease symptoms begin 3-21 days after exposure and typically increase in severity. Filovirus-induced diarrhea can be quite serious, reaching 10 liters per day and resulting in severe dehydration, contributing to the pathogenesis of these viruses (104). Hemorrhage presents as petechiae, bleeding from gums, venipuncture sites, subconjunctival hemorrhage, as well as blood in vomit and stool (104). Patients typically experience multiorgan failure and hypovolemic shock leading to death (104).
Pregnant women and infants face an elevated risk of Ebola hemorrhagic fever and increased risk of mortality (105). Previous outbreaks have recorded extremely high case fatality rates for pregnant women and newborn infants, reaching 90% and 100% respectively (106). Filovirus infection during pregnancy often leads to fetal loss (107), but MTCT is not well defined, due to the sporadic and often rural nature of outbreaks as well as the potential of the viruses to penetrate multiple tissue compartments. The detection of EBOV RNA in the amniotic fluid well after viral clearance in the mother, suggests that transplacental viral transmission might be feasible (108). In addition, EBOV RNA was recovered from breastmilk (109, 110) and multiple studies suggest possible MTCT of EBOV during breastfeeding (111, 112).
Maternally Acquired Antibodies and Implications for Prenatal Immunization
To date, there is one FDA approved vaccine (Ervebo®) for EBOV infection for adults >18 years of age. Ervebo® is a replication competent recombinant vesicular stomatitis virus (VSV) expressing EBOV glycoprotein. This vaccine elicits potent humoral responses generating high titers of IgG (geometric mean titer of 969.9-1307.3, day 28 post vaccination) as well as high titers of neutralizing antibody (geometric mean titer of 122.8-211.7, day 28 post vaccination) (113). The efficacy of Ervebo® was evaluated during an outbreak in Guinea and was found to be 100% at preventing EBOV infection (113). While there are no universally established immune correlates of protection for the EBOV vaccine, the role of humoral responses in EBOV-associated disease outcomes has been implicated (114).
Currently, there are no vaccines tested prenatally for the prevention of EBOV or MARV. Clinical trials for development of the Ervebo® vaccine did not explicitly involve pregnant women. In fact, this population was considered an exclusion criterion. However, follow up studies with women who became pregnant during the course of the clinical trial demonstrated that pregnancy loss amongst the immunized group was not statistically different than that of unvaccinated group (115). Based on these safety data, WHO now recommends Ervebo® during pregnancy under certain circumstances, when the benefits outweigh risks. Very recently, Johnson and Johnson have set up a phase 3 clinical trial to investigate the safety and immunogenicity of this vaccine in pregnant women (116). There are currently no vaccine options for MARV. However, preclinical studies of replication-competent VSV-based vaccines demonstrate protective efficacy in NHPs (117).
Animal Models to Guide Prenatal Vaccination Research
Mice, guineapigs, hamsters and NHPs have been widely used for filovirus research (118). As expected, NHPs such as, marmosets have similar MARV disease pathology to humans (118, 119), and different filoviral vaccine platforms have been studied in nonpregnant NHP models (120). However, to date, animal models of pregnant filoviral infections are lacking and data for vaccine efficacy during pregnancy remains limited. Thus, preclinical animal models that were utilized for development of the FDA-approved. EBOV vaccine for non-pregnant individuals could be employed to investigate the safety and efficacy of maternal vaccination against Filoviruses.
Viruses Vertically Transmitted From Mother to Infants Causing Congenital Defects and Neonatal Abnormalities
Several viruses such as HIV, ZIKV and CMV can be vertically transmitted from infected pregnant women to their babies. Vertical virus transmission can occur in utero by crossing the placental barrier, during delivery in the birth canal, or postnatally during breastfeeding. Congenital or neonatal infections can result in lifelong infection, developmental defects, physical deformities, and lifelong disabilities. In addition, maternal infection may result in pregnancy complications, including intrauterine growth restriction and miscarriages. For many congenital infections, timing of infection during gestation plays a critical role in disease severity and fetal outcome. Hence, immunization regimens that can generate protective maternal immunity prior to conception would be optimal to prevent such vertical transmissions and associated abnormalities.
Human Immunodeficiency Virus (HIV)
HIV is a member of the Retroviridae family comprising of a single-stranded, positive-sense RNA genome (14, 121). HIV is a major global public health concern with ~1.5 million people getting newly infected with the virus each year, globally (122). The virus is transmitted via exchange of body fluids such as blood, semen and vaginal secretions from infected individual. In addition, HIV can be vertically transmitted congenitally and postnatally from mother to their offspring in utero, during delivery or during breastfeeding (123). HIV continues to be a major threat for pregnant women, adolescent girls of childbearing age and children. In 2020, ~4200 adolescent girls and young women aged 15–24 years became infected with HIV every week, accounting for 50% of the total annual HIV infections. In addition, there were 150,000 new HIV infection among children (124).
Clinical Presentation and Associated Complications
Good clinical management such as early antiretroviral therapy (ART) initiation and adherence to treatment can reduce the risk of MTCT of HIV to <1-2% (125, 126), but implementation challenges such as suboptimal ART coverage of pregnant and breastfeeding women, poor access or adherence to ART, and late presentation for prenatal care prevent the complete elimination of vertical HIV transmission through ART alone. In addition, ~50% of the new infant HIV infections occur when a newly infected mother, who is not on therapy unknowingly transfers the virus to their offspring (127). Infants and children who are infected with HIV early in life from their mothers have to rely on lifelong ART to keep viral replication suppressed, and as a consequence, they are predisposed to ART-associated metabolic complications (128). Moreover, some ART regimens during pregnancy are associated with fetal complications such as neural tube disorders (129), preterm birth (130), hematological abnormalities (131), and mitochondrial dysfunction, later in life (129, 131). Congenital defects and neonatal abnormalities of HIV infection include developmental delays, neurological dysfunction, susceptibility to bacterial, viral and yeast infections, high persistent fever and weight loss (132).
Maternally Acquired Antibodies and Implications for Prenatal Vaccination
Studies from the pre-ART era have indicated that in the absence of any intervention, ~45% of infected pregnant women would transmit the virus to their babies (133). The fact that more than 50% of exposed infants remain uninfected, suggests that maternal genetic factors such as receptor and HLA subtypes, as discussed in (134) or immune factors could play a crucial role in providing partial protection against infant infections. Several studies have attempted to identify the maternal immune correlates of protection against vertical transmission. Notably, maternal antibodies against conserved regions of the HIV Env protein responses have been associated with reduced MTCT risk in multiple studies. For instance, the magnitude of maternal IgG antibodies specific for Env third variable loop (V3), neutralization of tier 1 viruses and the magnitude of CD4- binding site blocking antibodies were associated with reduced risk of MTCT in subtype B HIV-infected maternal cohort (135). The specificity of maternal V3-specific IgG responses that were associated with reduced MTCT risk was further mapped to the C-terminal region of the V3 loop (136). Another study linked maternal Env-specific Abs targeting the membrane-proximal external region (MPER) of gp41 with reduced risk of vertical HIV transmission among HIV subtype C-infected ART-experienced mothers (137). In contrast, maternal antibodies against certain HIV Env protein regions have also been associated with increased risk of MTCT. For instance, gp41 ectodomain targeting Abs have been associated with increased MTCT risk in clade C HIV-infected, ART-naïve, breastfeeding mother-infant pairs (138). Additionally, in a cohort of clade C HIV-infected, breastfeeding, ART-experienced women, IgG against the variable loops 1 and 2 (V1V2) and anti-CD4 binding-site Abs were associated with increased risk of MTCT of HIV, and no association was observed with maternal V3-specific IgG binding nor tier 1 virus-neutralizing responses (139). Thus, these findings suggest that maternal immune correlates of protection against MTCT might differ based on infecting virus clade, transmission mode and ART status.
Maternal autologous virus-specific neutralizing antibody responses have also been studied in the setting on MTCT of HIV. Some of these studies have indicated that viruses transmitted to infants are generally resistant to neutralization by maternal plasma suggesting that viral escape to neutralization may be important during vertical transmission (140–143). Additionally, maternal broadly neutralizing antibody responses may select for neutralization resistant T/F variants (144). Nevertheless, other studies have reported higher levels of neutralizing antibodies in women who transmitted the virus to their babies as compared to non-transmitting infected mothers (145). Thus, our understanding of the role of neutralizing antibodies during vertical transmission and the potential impact of transmission mode remains incomplete. Moreover, the role of maternal non-neutralizing antibody responses and MTCT risk also remains poorly defined. Breast milk Env-specific IgG responses with antibody-dependent cellular cytotoxicity (ADCC) activity have been associated with reduced risk of MTCT in some studies (146) but not in others (147). Interestingly, some studies have reported that ADCC activity of passively acquired antibody is associated with improved survival in HIV-infected infants, whereas neutralization activity is not (148, 149).
Boosting maternal immunity has been explored as a possible strategy to reduce vertical transmission. HIV Phase I vaccine regimens have demonstrated high levels of immunogenicity and safety in nonpregnant adult populations. Few therapeutic vaccine trials in pregnant women also demonstrated good safety profile (150). However, these limited sample size trials were not designed to evaluate efficacy. A recent analysis of samples from 11 HIV infected women who received Env-subunit vaccines during pregnancy reported an increase in binding antibody responses following vaccination. However, autologous neutralization potency in this small group of vaccinated women were comparable to that of 6 unvaccinated HIV infected pregnant women (151). As novel HIV vaccine immunogens are under development, further exploration of maternal immunization strategies to limit infant infection especially in the setting of postnatal breast milk transmission should be pursued.
Animal Models to Guide Development of Prenatal Vaccination Strategies
A globally effective HIV vaccine strategy must cope with the broad genetic diversity of HIV viruses, its ability to escape neutralizing antibodies, as well as different transmission routes, including MTCT. Preclinical NHP models, such as RMs infected with Simian/Simian-Human Immunodeficiency Virus (SIV/SHIV) recapitulates most virological and immunological hallmarks of HIV infection in humans and have been widely used in testing HIV vaccine immunogens. To model viral transmission during pregnancy, female RMs have been experimentally infected with SIV during various stages of gestation (152–154). Additionally, breastmilk transmission of HIV to infants has been mimicked by orally infecting infant NHPs (152, 154, 155). Using such an infant RM model of oral SHIV infection, the ability of human breastmilk-derived polyfunctional antibodies, with weak neutralization and antibody-dependent cellular cytotoxicity functions, to reduce HIV transmission was demonstrated (156). In addition, infant RM models have been utilized to study HIV in early life and to evaluate candidate pediatric vaccine regimens (157, 158) and immunotherapies (159–161).
Zika Virus (ZIKV)
ZIKV is a positive-sense single stranded RNA virus, belonging to the Flaviviridae family (14, 162). It is a mosquito-borne virus that was first identified in the Ziika forest of Uganda in 1947 (163). Since then, rare sporadic cases of ZIKV outbreak have been reported regionally in different parts of the world, including Asia, Americas, Africa and Pacific. These outbreaks were of little concern as they primarily caused asymptomatic infections or resulted in mild febrile symptoms. In 2015-16, ZIKV outbreak in Latin America and the Caribbean resulted in a public health emergency of international concern, particularly for pregnant women (163). It is estimated that during the 2015-16 ZIKV outbreak, ~1.5 million people were infected by the virus (164). During the peak of the ZIKV epidemic in 2016, more than 200,000 cases of ZIKV disease were reported in Brazil (165). In addition, in the US, ZIKV-associated birth defect occurred among 5-10% of babies born to ZIKV positive mothers (166).
Clinical Presentation and Associated Complications
ZIKV is primarily transmitted by Aedes mosquito bites, however, sexual transmission and transmission via blood transfusion has been reported (167). In the 2015-2016 outbreak, ZIKV was also identified to be transmitted vertically from mothers to their infants during pregnancy (163). In healthy individuals including pregnant mothers, ZIKV infection is typically asymptomatic or might cause mild febrile disease with rashes, conjunctivitis and arthralgia (168). However, vertical transmission results in neonatal birth defects. The congenital birth defects and abnormalities caused by ZIKV infection during pregnancy, also known as congenital zika syndrome (CZS), results in neurodevelopmental disabilities, severe microcephaly, visual impairments, motor delay and increased morbidity in the newborn (169, 170). ZIKV is transmitted from mother to child in 1 out of 10 ZIKV-infected pregnancies (169–171). Interestingly, although vertical transmission of the virus can occur at any time during gestation, first- and second-trimester infections have the highest risk of developing CZS in the newborn (172). Whether ZIKV can be transmitted during breastfeeding is currently unknown. Although ZIKV RNA and infectious virus has been detected in the breastmilk of ZIKV-infected mothers, viral transmission during breastfeeding has not been confirmed (173), and future studies with bigger cohorts will be needed to completely rule out the possibility of MTCT of ZIKV via breast milk
Maternally Acquired Antibodies and Implications for Prenatal Immunization
To date, there is no approved vaccine to prevent ZIKV infection. Diverse vaccine platforms including DNA, mRNA, subunit, live-attenuated and purified inactivated vaccines have been tested in non-pregnant animal models of ZIKV infection, and several of them have already entered Phase I/II clinical trials. In addition to being protective against ZIKV infection, an ideal vaccine candidate should be able to prevent vertical transmission of the virus and associated fetal complications. Studies have indicated that humoral immunity, specifically neutralizing antibodies play an important role in achieving protection against ZIKV infection (174, 175). Furthermore, ZIKV-specific IgG antibodies were found to efficiently cross the placenta (176). Hence developing a prenatal vaccination regimen able to protect mothers from infection and prevent vertical transmission seems to be an attractive strategy. Since ZIKV infection early during gestation has been associated with increased fetal disease severity (172), it is likely that vaccination prior to conception or early during pregnancy would be necessary to prevent transmission and improve fetal outcomes. Furthermore, delineating the correlates of fetal protection and most importantly, levels of antibodies necessary to achieve protection against in-utero transmission will be necessary to design a protective vaccination regimen. The fact that ZIKV is only one serotype makes vaccine design slightly less challenging as neutralizing antibodies developed against one strain would potentially neutralize others. However, a key concern for ZIKV vaccine design is elicitation of cross-reactive antibody responses with co-endemic DENV serotypes, which are antigenically similar flaviviruses (177). Cross reactive antibodies that can target conserved epitopes of ZIKV and DENV serotypes, may contribute to ADE of infection against subsequent viral infections (178, 179).
Animal Models to Guide Prenatal Vaccination Research
To determine the mechanisms of severe CZS in the fetus and ability of maternal vaccination to prevent vertical ZIKV transmission, relevant animal models to study maternal immune response and fetal ZIKV pathogenesis will be necessary. Mice models are often utilized to study ZIKV pathogenesis. However, for efficient replication of the virus in mouse models, transgenic type I interferon knockout mice or wild type dams treated with type I IFN receptor specific blocking antibody are preferred (180). More recently, several groups have also successfully recapitulated vertical ZIKV transmission and adverse fetal outcomes in wild type mice (181, 182). In the context of pregnancy, while the short breeding times and large litter size of mice is definitely advantageous, this model cannot accurately mimic human pregnancy primarily owing to the differences in the placental organization (183). Other animal models such as rats and pigs have been used to study vertical ZIKV transmission (180). The most relevant and widely used model for studying vertical ZIKV transmission during pregnancy and CZS-associated pathogenesis are NHPs such as RMs. In fact, ZIKV was originally isolated from a RM in 1947 from the Ziika forest and hence, RMs serve as natural ZIKV hosts (184). Coffey et al. established an intravenous and intraamniotic ZIKV-infected pregnant RM model, in which ZIKV pathogenesis and ZIKV-specific immune response comparable to humans were observed (185). In addition, ZIKV-infected RM model was also able to mimic the higher rates of congenital defects in fetus of mothers infected during first trimester vs. those infected during third trimester (186, 187). These models are currently used to assess the efficacy of prenatal ZIKV vaccination to prevent fetal transmission and associated abnormalities. Notably, Van Rompay et al. assessed a DNA vaccine regimen in pregnant RM model of ZIKV infection, where RMs were immunized before conception. While this vaccine was not able to completely prevent vertical transmission of the virus, maternal viral loads and fetal disease severity was reduced by vaccination (188), highlighting the need for further research in this area.
Cytomegalovirus (CMV)
CMVs are large double-stranded DNA viruses belonging to the Herpesviridae family (14). CMV prevalence is estimated at 83% globally and at 86% for women of childbearing age (189). Transmission occurs by direct contact with bodily fluids, blood products, and following organ transplants (190, 191). In addition, the virus is also transmitted vertically from mother to the fetus via placenta. In fact, in-utero transmission of CMV is the most common cause of congenital infection, as 1 in every 200 babies are born annually with congenital CMV infections (192). The greatest challenge with CMV infection, is their lifelong latency in the host and their ability to reactivate. The risk of placental CMV transmission is greater for seronegative women who have primary CMV infection during pregnancy (30-50%), as compared to chronically infected women (1-4%) (193, 194). In contrast, among seropositive women, reactivation of latent CMV or chronic infection accounts for majority of the congenital infections (60-90%) (195, 196). Due to the global high prevalence of CMV, congenital CMV infections more commonly attributable to reactivations of maternal infection than to primary infections during pregnancy.
Clinical Presentation and Associated Complications
Among healthy individuals including pregnant women, CMV infection, is typically asymptomatic or mild with flu-like symptoms (197, 198). Congenitally infected infants develops symptoms such as rash, jaundice, microcephaly, hepatosplenomegaly and vision loss, (199–201). In 20% of cases, congenital CMV is associated with hearing loss and developmental delays (194, 202, 203). In addition to in-utero transmission, vertical transmission of CMV can also occur through viruses secreted in the birth canal, saliva, and urine (204, 205). Moreover, the virus can be transmitted postnatally via breastfeeding causing symptomatic disease in preterm (<32 weeks) and very low weight infants (205–211), while, breastmilk transmission in full-term infants is usually asymptomatic (212). The risk for in-utero congenital transmission following primary maternal infection increases with gestational age with 40-70% transmission risk at the third trimester of pregnancy (213–216). However, the risk of an infant developing defects and sequelae is greatest when transmission occurs early in gestation (217).
Maternally Acquired Antibodies and Implications for Prenatal Immunization
CMV-specific antibodies transferred from mothers to infants in utero and during breastfeeding confers partial protection from CMV infection to infants (218). Interestingly, Saccoccio et al., determined no association between plasma levels of CMV virion-specific IgG and postnatal CMV, yet identified a weak association between infant glycoprotein B (gB)-specific IgG levels and protection against mucosal virus acquisition (219). Although CMV-specific antibodies in breastmilk may confer some protection in infants, there are instances of CMV reactivation in the mother, resulting in viral shedding in the breast milk (218) that can result in infection of the infant. To avoid this mode of transmission to a vulnerable preterm infant population, in France, breast milk pasteurization is recommended for premature infants (<32 weeks gestational age, <1500g birth weight) (220). Currently, there is no licensed CMV vaccine, both due to lack of defined immune correlates of protection and the vast immune evasion techniques of the virus (221). Of the 12 vaccines under clinical development since 2017, the gB/MF59 subunit vaccine has been one of the most successful to date, conferring approximately 50% efficacy in prevention of CMV acquisition in two studies. The elicited antibodies exhibited limited virus neutralization, highlighting the potential importance of non-neutralizing antibody functions (221–223). Moreover, it has been hypothesized that T-cell responses and both neutralizing and non-neutralizing antibody functions will likely be needed for a protective CMV vaccine (224). An mRNA-based vaccine (mRNA-1647) comprised of mRNAs of CMV surface proteins glycoprotein B and pentameric complex was tested for safety and immunogenicity in a phase I trial by Moderna (225). The advantage of this vaccine over a gB vaccine was inclusion of pentameric complex (PC), since antibodies to PC can neutralize infection of epithelial cells, endothelial cells and myeloid cells (228, 229), as compared to gB, that can only neutralize infection of fibroblasts (230, 231). In addition, the majority of the neutralizing antibodies in CMV-seropositive individuals are targeted against CMV PC (228). Based on the Phase I data for this vaccine, a phase II safety and efficacy trial involving healthy seronegative and seropositive individuals is underway (226) and very recently, the company has launched a phase III trial to test the safety and efficacy for this vaccine in women of childbearing age (227).
Animal Models to Guide Development of Prenatal Vaccination Strategies
Mice, rat, and guineapig models using species-specific CMV are the primary small animal models used to study viral dissemination (232). The guinea pig model can also be used to study vertical transmission at a much lower resource cost compared to NHPs, but it may be less advantageous and clinically relevant because of differences in the virus homology, placental anatomy, and pathogenesis in guineapigs vs. human (233–237). Species-specific CMV infecting RMs (RhCMV) and those infecting humans (HCMV) are similar in their genomic and amino acid composition, viral pathogenesis, fetal transmission, fetal sequelae (238). HCMV and RhCMV share 60% identity in their gB protein which is critical to further study the current vaccine platforms (237, 239). Notably, Bialas et al. developed a RM model of congenital CMV infection and demonstrated that maternal CD4+ T cell immunity during primary rhCMV infection is important for controlling maternal viremia and inducing protective immune responses that prevent severe CMV-associated-fetal disease (240). Furthermore, this RM model have also been utilized to study the protective roles of passively infused maternal antibodies (241) and hence are instrumental for pre-clinical studies to guide development of a protective vaccine for prevention of congenital CMV infections (238, 242).
Viruses Causing Severe Diseases in Neonates and Infants
Owing to the developing immune system, neonates and infants are particularly vulnerable to infectious diseases during the first few months of their life. Infectious diseases are responsible for >60% of child mortality, of which >40% occur during the first month of life (5). During this period, when the neonatal immune system is undergoing maturation, maternal IgG transferred across the placenta and maternal IgA transferred via breastmilk are crucial to provide protection against infectious diseases (243). Vaccination during pregnancy will be able to generate robust maternal antibody responses that will be transferred to the newborns, thereby protecting them against severe infections. However, predicting the correct timing of immunization of the mother and having an understanding of the antibody kinetics and half-life will be crucial to achieve maximum protection of the newborn.
Rotavirus (RV)
RV is an infectious double stranded RNA virus belonging to the Reoviridae family (14, 244) that can be transmitted through the fecal-oral route via contaminated fomites and aerosolized droplets generated while projectile vomiting (245). While RV infection is typically mild in adults, infection with this virus causes severe gastroenteritis in infants and young children, which could result in death (246). In fact, RV is the most common cause of diarrhea in neonates and infants, the highest risk group being infants between 3 and 24 months of age (245). In 2016, this virus caused over 258 million cases of diarrhea globally in children < 5 years of age, and ~215,000 children died of RV-induced diarrheal disease (246, 247).
Clinical Presentation and Associated Complications
RV mainly infects cells in the villi of intestines and virions are shed in high titers in stool (>1010-1011 RV particles/gram of stool) and vomitus (248). Vertical transmission of RV perinatally or during breastfeeding has not been reported to date. In adults, RV infection can be asymptomatic, or associated with nausea, malaise, headache, abdominal cramping, mild diarrhea and fever (249, 250). RV disease symptoms in infants and children typically begin within 48 hours of infections and include diarrhea, intestinal pain and vomiting (245). Children over 3 months of age and immunocompromised infants usually experience prolonged disease which can increase risk of complications such as dehydration, electrolyte imbalance and metabolic acidosis (251).
Maternally Acquired Antibodies and Implications for Prenatal and Infant Immunization
To date, there are 4 licensed vaccines for prevention of RV-associated gastroenteritis in infants, of which 2 are primarily recommended by FDA and WHO (Rotarix and Rotateq) (251). Currently, CDC recommends infant RV immunizations in 2-3 doses with in the first 6 months of life, but these pediatric vaccines do not provide protection during the first months of life, highlighting that a maternal immunization approach will be needed to ensure protection of newborns. Payne et al, recently demonstrated the presence of maternal RV-specific IgG in infants born to naturally RV-infected US mothers (252), highlighting the feasibility of maternal vaccine-induced antibody transfer in infants. However, to achieve maximum protection in the first months of life, the timing of maternal RV immunization in the setting of current infant vaccination schedule needs to be evaluated. Interference from maternally acquired antibodies is considered as a major correlate for reduced infant vaccine efficacy, particularly those from the low-and-middle income countries (LMICs) (253). For instance, a clinical trial of Rotavac in Indian infants determined an inverse relationship between maternal IgG levels and infant vaccine response (254). Similarly, anti-RV antibodies in breastmilk have been associated with decreased vaccine efficacy in infants (255). To circumvent this maternal antibody interference, vaccinating the pregnant women and delaying the timing of infant immunization until the maternal antibody wanes could be beneficial. This strategy would passively protect the infants from RV-associated diseases for the first few months of life, followed by boosting of immune response with infant vaccination. Innate susceptibility factors such as polymorphism in specific genes (alpha 1,2 fructosyltransferase, FUT2) can impact development of RV and norovirus (NV)-specific IgG, IgA and neutralizing antibody titers both in adults and infants (256). Hence, while developing RV or NV maternal vaccines, the roles of such innate susceptibility factors need to be taken into consideration.
Animal Models to Guide Development of Prenatal Vaccination Strategies
Several pre-clinical animal models of RV infection and pathogenesis are available that has the potential to be developed as tools to investigate prenatal vaccination strategies and protective efficacy of maternally derived antibodies in infants. RV disease and lethality are studied in wild type and immunodeficient mouse models, although mouse-specific RV strains are critical to infect wild type mice, due to lack of lethality and limited symptoms of human strains in such models (257). Mice also lack emetic response which makes studying vomiting, a hallmark symptom of RV infection, in the mouse model challenging (250). Very recently, Langel et al. has highlighted the protective efficacy of maternally-transferred dimeric IgA on suckling mouse pups (258). Guinea pigs are often used in infectious disease and vaccine development studies as they more closely model disease pathology to humans than mice (259, 260). A recent study utilized guinea pigs in a pre-clinical trivalent RV vaccine study to determine immunogenicity characteristics (261). Neonatal and gnotobiotic piglets have been used to study breastmilk-induced protection against rotavirus C (RVC), where lower levels of milk IgG and IgA in nursing pigs resulted in higher levels of clinical symptoms in their offspring (262). RV disease pathogenesis has also been tested in NHP models such as neonatal RMs (263). While maternal RV vaccination to attain protection in infants are being studied using mouse models (264), development of a RM models that can mimic clinical symptoms of RV-induced diseases, such as vomiting, to study maternal immunization and transplacental transfer of maternal antibodies will be crucial.
Norovirus (NV)
NV is a positive-sense single stranded RNA virus belonging to the Caliciviridae family (14, 265). NV is the leading cause of foodborne disease outbreaks worldwide, resulting in contagious gastroenteritis (266). Although this virus can infect individuals from all age groups, higher incidence is typically observed in children. In the US, NV is responsible for nearly 1 million pediatric medical care visits annually (268). In LMICs, NV infection is associated with approximately 200,000 deaths in children under the age of 5, annually (266). This virus spreads through the fecal-oral route and is transmitted via fomites in crowded or close living areas. Childcare, college campuses, cruise ships, and hospitals, and catered events are often sources of NV outbreaks (267).
Clinical Presentation and Associated Complications
Signs and symptoms of NV-associated disease include diarrhea, nausea, vomiting, stomach pains, fever and body aches. This virus has a brief incubation of only 12-48 hours post exposure, and complications from infection arise mainly from dehydration and electrolyte imbalance. Children under the age of 5 are more likely to develop dehydration with NV acute gastroenteritis (269), however neonates often experience asymptomatic infection with NV (269, 270), suggesting that maternally acquired virus-specific antibodies might play a crucial role in protection against NV during early life. Although, genetic factors such as presence of histo blood group antigens (HBGA) may also play crucial role (271, 272). NV evolution results in novel dominant pandemic strains every 2-5 years (273), and owing to the fluctuations in prevalence and a lack of reliable culturing system, there are currently no maternal or infant licensed vaccines for NVs (274). MTCT of NV is uncommon.
Maternally Acquired Antibodies and Implications for Prenatal Immunization
The protective role of maternal breast milk antibodies against NV has been highlighted in recent studies, where maternal anti-NV-IgA was associated with lower prevalence and less severity of infant NV diarrheal disease (270, 275). Interestingly, while there was no difference in disease severity with breastfeeding in older infants (276), other studies have found that high titers of NV-specific IgA in breastmilk was protective against symptomatic NV infection in infants (275), potentially suggesting that virus-specific IgA are important immune correlate of NV-associated severe disease outcome. Hence, maternal vaccines that can elicit robust NV-specific mucosal IgA responses, that eventually gets transferred to infants via breastfeeding needs to be explored as a strategy to mitigate infant NV, RV or other gastrovirus-associateddiarrheal disease.
Animal Models to Guide Development of Prenatal Vaccination Research
NV pathogenesis, host immune response and vaccination approaches are studied in multiple animal models such as transgenic mouse, gnotobiotic pigs, calves, pigtailed macaques, tamarins and RMs. (277, 278). However, a challenge of employing some of these models such as mice and calves to study maternal vaccination is their dissimilarities in placentation mechanisms compared to humans and lack of transplacental IgG transfer. Therefore, further research in developing NHP models such as RMs which have long gestation period, and a hemochorial placenta like humans (279) to study NV pathogenesis and maternal antibody transfer remains critical.
Respiratory Syncytial Virus (RSV)
RSV is a common respiratory virus belonging to the Pneumoviridae family (14) that is transmitted through contact with droplets from infected people. RSV has a single negative-stranded RNA genome (280). In the US, nearly all children become infected with RSV by the age of 2 with 75,000-125,000 hospitalizations per year. RSV infection is the most common cause of bronchiolitis and pneumonia in children less than 1 year of age, with infant <6 months of age responsible for 50% infections (6, 281).
Clinical Presentation and Associated Complications
RSV infection in healthy adults typically resolve in a week or two, however the virus can cause severe disease manifestations, resulting in hospitalization in infants and children. Severe RSV diseases is associated with breathing difficulties, cough, respiratory distress syndrome, pneumonia and respiratory failure (282). RSV infection during pregnancy also results in acute respiratory illness, which may be clinically severe and lead to hospitalization (283, 284). While evidence of MTCT of this virus is rare, maternal RSV infection can potentially alter infant immunity, resulting in lower airway lymphocyte and cytokine profiles that are significantly different from that of an RSV-naïve host during a first postnatal RSV lower respiratory tract infection (285).
Maternally Acquired Antibodies and Implications for Prenatal Immunization
The protective role of RSV-specific antibodies that are trans-placentally transferred from mother to infants have been recently studied in a cohort of 587 mother-infant pairs who were followed from the time of birth to 6 months of age (286). While a strong correlation was observed between maternal and infant RSV antibody titers, it was not very clear why certain RSV-infected infants suffer from severe disease symptoms even in the presence of high levels of RSV-specific antibodies from their mothers. In order to define immune correlates of protection against RSV-infected in infants, Jans et al, compared the RSV-specific antibody responses between infected hospitalized infants to those of uninfected infants. While infected infants demonstrated lower RSV-specific avidity as compared to uninfected infants, there was no association between neutralization potency or antibody epitope-specificity and disease severity (287). Identifying the functional response of antibodies associated with RSV-protection is critical to facilitate the development of maternal vaccination approaches that can elicit protective antibody response to prevent RSV infection severity in infants.
There are currently no licensed vaccines against RSV, although several platforms are under investigation in both non pregnant and pregnant groups. For instance, a RSVpreF3 vaccine was found to be well tolerated and immunogenic in a cohort of non-pregnant women (288). In addition, a single intramuscular dose of RSV fusion protein-based nanoparticle vaccine in pregnant women showed protection against RSV infections in infants, with 1.5% of infants in the vaccine group getting infected compared to 2.4% in the placebo group. This immunization regimen was also able to successfully reduce the percent of hospitalization from 3.7% in the placebo group to 2.1% in the vaccine group (289). This immunization regimen did not result in any adverse side effects in pregnant women. However, this study was not powered to estimate the vaccine efficacy, and hence, better powered, and extensive studies of maternal immunization and protection from RSV infection during infancy are warranted.
Animal Models to Guide Prenatal Vaccination Regimens
To study RSV replication and pathogenesis, animal models such as mice, cotton rats, sheep and most commonly NHPs have been infected with human RSV (290). Chimpanzees have been previously used in vaccinology research to evaluate the protective efficacy of RSV vaccine candidates (291). However, due to their restricted use in research, NHP models such as African Green Monkeys (AGM) and macaques are often used. AGM is only semi-permissive to the virus, whereas RMs do not mimic the clinical symptoms observed in humans (290). In a recent study, three different NHP models, baboons, AGM and RMs were experimentally infected with RSV and the kinetics and titers of virus-specific antibody development was evaluated (292). Of these NHP models, AGMs demonstrated high levels of RSV-specific antibody titers and serum IgG levels of the mother correlated with IgG levels of the infants that remained detectable 4-5 months post birth. Hence this model was selected as most suitable for studying prenatal vaccination strategies. This study indicated that, immunization of pregnant mothers with RSV prefusion F protein resulted in virus-specific antibodies in the infants that were both higher in magnitude and durability compared to those of unvaccinated controls. Further studies to evaluate protective efficacy of these antibodies against infant RSV challenge will be crucial.
Herpes Simplex Virus (HSV)
HSVs are large double-stranded DNA viruses belonging to the Herpesviridae family (14), that can be categorized into two types: HSV-1 and HSV-2. Globally, ~ 67% (under the age of 50) and 13% (aged 15-49) individuals are infected with HSV-1 and HSV-2, respectively (293). HSV is common among women of reproductive age, and it is estimated to affect 2-3% of pregnant women (294). Pregnant women infected with HSV-1 and HSV-2 can transmit the virus to their newborns resulting in neonatal HSV infection (295).
Clinical Presentation and Associated Complications
In adults, HSV is primarily a sexually transmitted virus associated with physical symptoms like cold sores and genital lesions. HSV can be largely asymptomatic as the virus persist in a latent state throughout the lifespan of the infected individual, with occasional reactivation (296, 297). Infant’s symptoms can vary and may include ulcerations of eye or mucous membranes, lethargy, seizures, encephalitis, respiratory failure, and mortality (298). Up to 85% of MTCT of HSV occurs in the birth canal during the time of delivery from exposure to HSV in the vaginal mucous membranes, whereas 10% of transmissions occur postnatally, when HSV is primarily transmitted to the infant through microlabial or cutaneous lesions (298–300). In-utero transmission is rare, occurring in 1-5% of cases (298). In 2017, HSV-1 and HSV-2 were estimated to cause 14,000 annual infections globally among neonates (301).
Maternally Acquired Antibodies and Implications for Prenatal Immunization
HSV-specific antibodies with potent neutralizing functions have been detected from cord blood and neonatal sera of infants born to HSV-1 and HSV-2-infected mothers, highlighting the efficient transplacental transfer of HSV-specific antibodies (302, 303). Using a mouse model, successful maternal transfer of HSV-specific IgG to the neonatal trigeminal ganglion (304) and maternal breast milk was also demonstrated (305). These findings indicate that a maternal vaccine for HSV infection might be able to protect neonates via placentally-transferred HSV-specific IgG during their first few months of life. However, to date, there is no licensed HSV vaccine available for non-pregnant or pregnant populations (306, 307). HSV’s immune evasion techniques including cell to cell spread, Fc receptor analog production, inhibition of complement-mediated lysis, and the establishment of latency makes vaccine design especially difficult (308–314). Furthermore, a randomized, double blind efficacy trial conducted in 2012 with over 8,000 women found that even though an HSV-2 gD subunit vaccine elicited neutralizing antibodies, vaccine efficacy was only 20% (223, 315), further underscoring the need for investigating the potential protective role of additional antibody and other immune functions, in order to guide development of an efficacious vaccine.
Animal Model to Guide Development of Prenatal Vaccination Strategies
Pregnant and neonatal mice models have been used to study HSV infection (316, 317). A mouse model has been used to study the trivalent HSV-2 subunit, replication defective live attenuated d15-29 and HSV-1 0ΔNLS vaccine candidates (307). Cotton rats have also been used for HSV vaccinology research ( (316).The guinea pig model of genital herpes best mimics human disease and has been currently employed for HSV vaccine research. This model allows for assessment of acute and recurrent HSV disease and viral shedding (306, 316). RM model of HSV-2 infection demonstrates histologic evidence of acute pathology, latency establishment in the ganglia, and viral reactivation. However, further modification will be necessary to conduct studies of maternal infection and placental antibody transfer in the RM model, as HSV-2-specific antibodies were only detected in 30% of non-pregnant animals after vaginal inoculation of HSV-2 strains (318).
Hepatitis B Virus (HBV)
HBV is one of the few members of the hepadnaviridae family with a partially double stranded DNA genome (319) (14). It is one of the most prevalent viruses with over 250 million people estimated to be chronically infected, globally (320, 321). The virus is transmitted mainly through bodily fluids, the most common routes of transmission being perinatally from mothers to their offspring during delivery, and between adults through sexual intercourse, percutaneous injection, piercings, tattoos, and intravenous drug use (322). Apart from getting infected during delivery, children may be infected with HBV through body fluid exposure from an infected individual (323). But to date, there is no strong evidence to suggest that the virus can be transmitted via breastfeeding. Individuals infected as neonates and children have the highest burden of chronic HBV. Without postexposure immune-prophylaxis, approximately 40% of the infants born to HBV-infected mothers will develop chronic HBV (324).
Clinical Presentation and Associated Complications
As a leading cause of liver fibrosis, hepatocellular carcinoma, and other related comorbidities, the effect of HBV infection on health is profound (325, 326). There are two major courses of HBV pathogenesis; acute hepatitis that resolves completely over the course of weeks to months, and chronic hepatitis that results in a carrier state in the infected individual (322). While chronic hepatitis is largely asymptomatic, there is ongoing viral replication that over the course of years often progresses to fibrosis and is associated with high risk of hepatocellular carcinoma, (327). Chronic HBV is also associated with extrahepatic diseases including serum-like sickness, vasculitis (polyarteritis nodosa), and kidney diseases (membranous glomerulonephritis, and membranoproliferative glomerulonephritis) (328). Individuals infected as neonates and children have the highest burden of HBV disease due to the length of chronicity. Additionally, the likelihood of acute hepatitis progressing to chronic one in an individual is largely dependent on their age at the time of infection. Adults infected with HBV have a 1-5% chance of becoming carriers, children have a 20-30% chance, and neonates have a 90-95% chance of chronic infection (329–333).
Maternally Acquired Antibodies and Implications for Prenatal Immunization
Because of the risk associated with perinatal HBV transmission due to primary or chronic maternal infection, pregnant women are routinely screened for HBV surface antigen. If the pregnant women is HBV surface antigen negative and at high risk of acquiring HBV, maternal vaccination is recommended to achieve protection against HBV infection. Alternatively, if the mother screens positive for current infection at delivery, the neonate receives hepatitis B immunoglobulin (HBIg) prophylaxis in addition to the standard first dose of the HBV vaccination series within 12-24 hours of birth. Subsequent boosters for the vaccination regimen occur at 1 month and 6 months of age (334). The virus usually takes more than 24 hours to establish chronicity and hence HBIg treatment is capable of reducing infant infcetion by more than 90% (335). The major barriers currently faced in this effort is the lack of maternal screening in endemic areas and failure of implementation of this prophylactic regimen at birth. Moreover, even in areas of widespread adoption of this practice, there are still 1-9% of cases of breakthrough infection, mainly associated with delayed or incomplete immunoprophylaxis (336). Notably, cord blood HBV antibodies correlated strongly with maternal HBV antibody levels post maternal vaccination, highlighting prenatal vaccination could be an additional approach to protect the newborn against HBV infection (337). Importantly, a three dose HBV vaccine regimen has been found to be safe when administered during pregnancy (338).
Animal Model to Guide Development of Prenatal Vaccination Strategies
HBV’s complex life cycle and narrow host range has significantly limited the availability of animal models that can recapitulate HBV pathogenesis in humans. Consequently, animal models to study HBV prenatal vaccinations are lacking. Chimpanzee is the only NHP model which closely mimics human HBV disease pathogenesis and has been previously used for HBV vaccine and therapeutic research (339). However, chimpanzee models are no longer used for research purposes due to limited animal availability and ethical constraints. The other available models to study HBV pathogenesis are mouse models than can be manipulated to support infection, and mammals and bird species that support hepatitis viruses with a similar life cycle to HBV (340, 341). However, mouse models require genetic manipulation, humanization, and immune cell depletion to support infection and mammalian and bird models such as tree shrew, woodchuck, and duck do not closely recapitulate HBV MTCT. Although there is a lack of appropriate animal models for HBV vaccine research, models for MTCT are limited as effective vaccines and immunoprophylaxis have already been developed and are widely and successfully utilized.
Conclusion
Strategic maternal immunization protocols can allow to simultaneously protect pregnant women, their unborn fetus and neonates from disease severity due to viral infections. The key to the success of such prenatal immunization regimens will be their ability to induce protective humoral responses and the timing of administration, which will likely depend on the viral disease. Hence, mechanistic understanding of the disease pathogenesis in the setting of pregnancy will be crucial to optimize currently available and novel vaccine platforms. In addition, since pregnancy is a period associated with physiological and immunological alterations, and due to the high potential benefits of building protective and safe vaccination regimens tailored to pregnant women, this population needs to be included in early-stage vaccine research. Hence, the development of relevant pre-clinical animal models of infection whose pregnancy, placentation and IgG transfer mechanisms are closely related to that of humans needs to be prioritized. Finally, successful implementation and maximum coverage of maternal vaccines will require extensive education and communication with the entire community and pregnant women as well as improvement in prenatal health care access to all women.
Author Contributions
RG, CG, IM, SB, CW, DR, MG, CP, and AB performed literature search for the review. RG generated figures for the review. RG, CG, IM, SB, CW, DR, MG, CP, AB, JP, SP, and GF drafted the manuscript. All authors contributed to the article and approved the submitted version.
Funding
The work was supported by National Institutes of Health grants R01 AI131978 (GF) and R01 AI161008-01 (GF), P01 AI129859 (SP), R21 AI147992 (SP), P01 AI117915 (SP), R01 AI162245 (SP) K01OD024877 (JP) and Duke University Interdisciplinary Research and Training Program in AIDS fellowship 5T32AI007392-31 (SB). The funders had no role in study design, data collection and analysis, decision to publish, or preparation of the manuscript.
Conflict of Interest
SP provides individual consulting services to Moderna, Merck, Dynavax, Pfizer, and HOOKIPA Biotech GmbH. Merck Vaccines and Moderna have provided grants for her Institutional sponsored programs.
The remaining authors declare that the research was conducted in the absence of any commercial or financial relationships that could be construed as a potential conflict of interest.
Publisher’s Note
All claims expressed in this article are solely those of the authors and do not necessarily represent those of their affiliated organizations, or those of the publisher, the editors and the reviewers. Any product that may be evaluated in this article, or claim that may be made by its manufacturer, is not guaranteed or endorsed by the publisher.
References
1. Silasi M, Cardenas I, Kwon JY, Racicot K, Aldo P, Mor G. Viral Infections During Pregnancy. Am J Reprod Immunol (2015) 73:199–213. doi: 10.1111/aji.12355
2. Robinson DP, Klein SL. Pregnancy and Pregnancy-Associated Hormones Alter Immune Responses and Disease Pathogenesis. Horm Behav (2012) 62:263–71. doi: 10.1016/j.yhbeh.2012.02.023
3. Villar J, Ariff S, Gunier RB, Thiruvengadam R, Rauch S, Kholin A, et al. Maternal and Neonatal Morbidity and Mortality Among Pregnant Women With and Without Covid-19 Infection: The INTERCOVID Multinational Cohort Study. JAMA Pediatr (2021) 175:817–26. doi: 10.1001/jamapediatrics.2021.1050
4. Racicot K, Mor G. Risks Associated With Viral Infections During Pregnancy. J Clin Invest (2017) 127:1591–9. doi: 10.1172/JCI87490
5. Liu L, Johnson HL, Cousens S, Perin J, Scott S, Lawn JE, et al. Global, Regional, and National Causes of Child Mortality: An Updated Systematic Analysis for 2010 With Time Trends Since 2000. Lancet (2012) 379:2151–61. doi: 10.1016/S0140-6736(12)60560-1
6. Rha B, Curns AT, Lively JY, Campbell AP, Englund JA, Boom JA, et al. Respiratory Syncytial Virus-Associated Hospitalizations Among Young Children: 2015-2016. Pediatrics (2020) 146(1):e20193611. doi: 10.1542/peds.2019-3611
7. Cdc. Guidelines for Vaccinating Pregnant Women (2016). Available at: https://www.cdc.gov/vaccines/pregnancy/hcp-toolkit/guidelines.html#:~:text=Live%20vaccines%20administered%20to%20a,generally%20are%20contraindicated%20during%20pregnancy (Accessed 2022).
8. Ganguli S, Chavali PL. Intrauterine Viral Infections: Impact of Inflammation on Fetal Neurodevelopment. Front Neurosci (2021) 15. doi: 10.3389/fnins.2021.771557
9. Murphy SK, Fineberg AM, Maxwell SD, Alloy LB, Zimmermann L, Krigbaum NY, et al. Maternal Infection and Stress During Pregnancy and Depressive Symptoms in Adolescent Offspring. Psychiatry Res (2017) 257:102–10. doi: 10.1016/j.psychres.2017.07.025
10. Estes ML, Mcallister AK. Maternal Immune Activation: Implications for Neuropsychiatric Disorders. Science (2016) 353:772–7. doi: 10.1126/science.aag3194
11. Van Den Bergh BRH, Van Den Heuvel MI, Lahti M, Braeken M, De Rooij SR, Entringer S, et al. Prenatal Developmental Origins of Behavior and Mental Health: The Influence of Maternal Stress in Pregnancy. Neurosci Biobehav Rev (2020) 117:26–64. doi: 10.1016/j.neubiorev.2017.07.003
12. Pal M, Berhanu G, Desalegn C, Kandi V. Severe Acute Respiratory Syndrome Coronavirus-2 (SARS-Cov-2): An Update. Cureus (2020) 12:e7423. doi: 10.7759/cureus.7423
13. Zhou P, Yang X-L, Wang X-G, Hu B, Zhang L, Zhang W, et al. A Pneumonia Outbreak Associated With a New Coronavirus of Probable Bat Origin. Nature (2020) 579:270–3. doi: 10.1038/s41586-020-2012-7
14. Ictv. International Committee on Taxonomy of Viruses. (2022). Available at: https://talk.ictvonline.org/taxonomy/.
15. Who. Who Coronavirus (Covid-19) Dashboard (2022). Available at: https://covid19.who.int/ (Accessed 2022).
16. Cdc. Scientific Brief: Sars-CoV-2 Transmission (2021). Available at: https://www.cdc.gov/coronavirus/2019-ncov/science/science-briefs/sars-cov-2-transmission.html (Accessed 2022).
17. Cdc. Data on COVID-19 During Pregnancy: Severity of Maternal Illness (2022). Available at: https://covid.cdc.gov/covid-data-tracker/#pregnant-population (Accessed 2022).
18. Villar J, Ariff S, Gunier RB, Thiruvengadam R, Rauch S, Kholin A, et al. Maternal and Neonatal Morbidity and Mortality Among Pregnant Women With and Without Covid-19 Infection: The INTERCOVID Multinational Cohort Study. JAMA Pediatr (2021) 175:817–26. doi: 10.1001/jamapediatrics.2021.1050
19. Lokken EM, Huebner EM, Taylor GG, Hendrickson S, Vanderhoeven J, Kachikis A, et al. Disease Severity, Pregnancy Outcomes, and Maternal Deaths Among Pregnant Patients With Severe Acute Respiratory Syndrome Coronavirus 2 Infection in Washington State. Am J Obstet Gynecol (2021) 225:77 e71–14. doi: 10.1016/j.ajog.2020.12.1221
20. DeSisto CL, Wallace B, Simeone RM, Polen K, Ko JY, Meaney-Delman D, Ellington SR. Risk for Stillbirth Among Women With and Without COVID-19 at Delivery Hospitalization — United States, March 2020–September 2021. MMWR Morb Mortal Wkly Rep (2021) 70(47):1640–5. doi: 10.15585/mmwr.mm7047e1
21. Fenizia C, Biasin M, Cetin I, Vergani P, Mileto D, Spinillo A, et al. Analysis of SARS-CoV-2 Vertical Transmission During Pregnancy. Nat Commun (2020) 11:5128. doi: 10.1038/s41467-020-18933-4
22. Hecht JL, Quade B, Deshpande V, Mino-Kenudson M, Ting DT, Desai N, et al. Sars-CoV-2 can Infect the Placenta and is Not Associated With Specific Placental Histopathology: A Series of 19 Placentas From COVID-19-positive Mothers. Mod Pathol (2020) 33:2092–103. doi: 10.1038/s41379-020-0639-4
23. Pique-Regi R, Romero R, Tarca AL, Luca F, Xu Y, Alazizi A, et al. Does the Human Placenta Express the Canonical Cell Entry Mediators for SARS-Cov-2? Elife (2020) 9:e58716. doi: 10.7554/eLife.58716
24. Bauerl C, Randazzo W, Sanchez G, Selma-Royo M, Garcia Verdevio E, Martinez L, et al. SARS-Cov-2 RNA and Antibody Detection in Breast Milk From a Prospective Multicentre Study in Spain. Arch Dis Child Fetal Neonatal Ed (2021) 107(2):216–21. doi: 10.1136/archdischild-2021-322463
25. Zhu F, Zozaya C, Zhou Q, De Castro C, Shah PS. Sars-CoV-2 Genome and Antibodies in Breastmilk: A Systematic Review and Meta-Analysis. Arch Dis Child Fetal Neonatal Ed (2021) 106:514–21. doi: 10.1136/archdischild-2020-321074
26. Kunjumon B, Wachtel EV, Lumba R, Quan M, Remon J, Louie M, et al. Breast Milk and Breastfeeding of Infants Born to SARS-CoV-2 Positive Mothers: A Prospective Observational Cohort Study. Am J Perinatol (2021) 38:1209–16. doi: 10.1055/s-0041-1731451
27. Chambers C, Krogstad P, Bertrand K, Contreras D, Tobin NH, Bode L, et al. Evaluation for SARS-CoV-2 in Breast Milk From 18 Infected Women. Jama (2020) 324:1347–8. doi: 10.1001/jama.2020.15580
28. Conti MG, Terreri S, Piano Mortari E, Albano C, Natale F, Boscarino G, et al. Immune Response of Neonates Born to Mothers Infected With SARS-Cov-2. JAMA Network Open (2021) 4:e2132563–e2132563. doi: 10.1001/jamanetworkopen.2021.32563
29. Flannery DD, Gouma S, Dhudasia MB, Mukhopadhyay S, Pfeifer MR, Woodford EC, et al. Assessment of Maternal and Neonatal Cord Blood SARS-Cov-2 Antibodies and Placental Transfer Ratios. JAMA Pediatr (2021) 175:594–600. doi: 10.1001/jamapediatrics.2021.0038
30. Gee S, Chandiramani M, Seow J, Pollock E, Modestini C, Das A, et al. The Legacy of Maternal SARS-CoV-2 Infection on the Immunology of the Neonate. Nat Immunol (2021) 22:1490–502. doi: 10.1038/s41590-021-01049-2
31. Rottenstreich A, Zarbiv G, Oiknine-Djian E, Zigron R, Wolf DG, Porat S. Efficient Maternofetal Transplacental Transfer of Anti- Severe Acute Respiratory Syndrome Coronavirus 2 (SARS-Cov-2) Spike Antibodies After Antenatal SARS-CoV-2 Bnt162b2 Messenger Rna Vaccination. Clin Infect Dis (2021) 73:1909–12. doi: 10.1093/cid/ciab266
32. Rottenstreich A, Zarbiv G, Oiknine-Djian E, Vorontsov O, Zigron R, Kleinstern G, et al. Timing of SARS-CoV-2 Vaccination During the Third Trimester of Pregnancy and Transplacental Antibody Transfer: A Prospective Cohort Study. Clin Microbiol Infect (2022) 28:419–25. doi: 10.1016/j.cmi.2021.10.003
33. Young BE, Seppo AE, Diaz N, Rosen-Carole C, Nowak-Wegrzyn A, Cruz Vasquez JM, et al. Association of Human Milk Antibody Induction, Persistence, and Neutralizing Capacity With SARS-Cov-2 Infection vs mRNA Vaccination. JAMA Pediatr (2021) 176(2):159–68. doi: 10.1001/jamapediatrics.2021.4897
34. Bleicher I, Kadour-Peero E, Sagi-Dain L, Sagi S. Early Exploration of COVID-19 Vaccination Safety and Effectiveness During Pregnancy: Interim Descriptive Data From a Prospective Observational Study. Vaccine (2021) 39:6535–8. doi: 10.1016/j.vaccine.2021.09.043
35. Shimabukuro TT, Kim SY, Myers TR, Moro PL, Oduyebo T, Panagiotakopoulos L, et al. Preliminary Findings of Mrna Covid-19 Vaccine Safety in Pregnant Persons. N Engl J Med (2021) 384:2273–82. doi: 10.1056/NEJMoa2104983
36. Kashani-Ligumsky L, Lopian M, Cohen R, Senderovich H, Czeiger S, Halperin A, et al. Titers of SARS CoV-2 Antibodies in Cord Blood of Neonates Whose Mothers Contracted SARS CoV-2 (Covid-19) During Pregnancy and in Those Whose Mothers Were Vaccinated With mRNA to SARS CoV-2 During Pregnancy. J Perinatol (2021) 41:2621–4. doi: 10.1038/s41372-021-01216-1
37. Shook LL, Atyeo CG, Yonker LM, Fasano A, Gray KJ, Alter G, et al. Durability of anti-Spike Antibodies in the Infant After Maternal COVID-19 Vaccination. JAMA (2022) 327(11):1087–9. doi: 10.1001/jama.2022.1206
38. Low JM, Gu Y, Ng MSF, Amin Z, Lee LY, Ng YPM, et al. Codominant IgG and IgA Expression With Minimal Vaccine mRNA in Milk of BNT162b2 Vaccinees. NPJ Vaccines (2021) 6:105. doi: 10.1038/s41541-021-00370-z
39. Butt AA, Chemaitelly H, Al Khal A, Coyle PV, Saleh H, Kaleeckal AH, et al. Sars-CoV-2 Vaccine Effectiveness in Preventing Confirmed Infection in Pregnant Women. J Clin Invest (2021) 131(23):e153662. doi: 10.1172/JCI153662
40. Birol Ilter P, Prasad S, Berkkan M, Mutlu MA, Tekin AB, Celik E, et al. Clinical Severity of SARS-CoV-2 Infection Among Vaccinated and Unvaccinated Pregnancies During the Omicron Wave. Ultrasound Obstet Gynecol (2022) 59:560–2. doi: 10.1002/uog.24893
41. Atyeo C, Deriso EA, Davis C, Bordt EA, Deguzman RM, Shook LL, et al. Covid-19 mRNA Vaccines Drive Differential Fc-functional Profiles in Pregnant, Lactating, and non-Pregnant Women. Sci Transl Med (2021) 3(617):eabi8631. doi: 10.1126/scitranslmed.abi8631
42. Damas J, Hughes GM, Keough KC, Painter CA, Persky NS, Corbo M, et al. Broad Host Range of SARS-CoV-2 Predicted by Comparative and Structural Analysis of ACE2 in Vertebrates. Proc Natl Acad Sci USA (2020) 117:22311–22. doi: 10.1073/pnas.2010146117
43. Chan JF, Zhang AJ, Yuan S, Poon VK, Chan CC, Lee AC, et al. Simulation of the Clinical and Pathological Manifestations of Coronavirus Disease 2019 (Covid-19) in a Golden Syrian Hamster Model: Implications for Disease Pathogenesis and Transmissibility. Clin Infect Dis (2020) 71:2428–46. doi: 10.1093/cid/ciaa644
44. Corbett KS, Gagne M, Wagner DA, Connell SO, Narpala SR, Flebbe DR, et al. Protection Against SARS-CoV-2 Beta Variant in mRNA-1273 Vaccine–boosted Nonhuman Primates. Science (2021) 374:1343–53. doi: 10.1126/science.abl8912
45. Munster VJ, Feldmann F, Williamson BN, Van Doremalen N, Pérez-Pérez L, Schulz J, et al. Respiratory Disease in Rhesus Macaques Inoculated With SARS-Cov-2. Nature (2020) 585:268–72. doi: 10.1038/s41586-020-2324-7
46. Shan C, Yao YF, Yang XL, Zhou YW, Gao G, Peng Y, et al. Infection With Novel Coronavirus (SARS-CoV-2) Causes Pneumonia in Rhesus Macaques. Cell Res (2020) 30:670–7. doi: 10.1038/s41422-020-0364-z
48. Tokars JI, Olsen SJ, Reed C. Seasonal Incidence of Symptomatic Influenza in the United States. Clin Infect Dis (2017) 66:1511–8. doi: 10.1093/cid/cix1060
49. Cdc. Estimated Flu-Related Illnesses, Medical Visits, Hospitalizations, and Deaths in the United States — 2018–2019 Flu Season (2021). Available at: https://www.cdc.gov/flu/about/burden/2018-2019.html (Accessed 2022).
50. Callaghan WM, Creanga AA, Jamieson DJ. Pregnancy-Related Mortality Resulting From Influenza in the United States During the 2009-2010 Pandemic. Obstet Gynecol (2015) 126:486–90. doi: 10.1097/AOG.0000000000000996
51. Siston AM, Rasmussen SA, Honein MA, Fry AM, Seib K, Callaghan WM, et al. Pandemic 2009 Influenza A(H1N1) Virus Illness Among Pregnant Women in the United States. JAMA (2010) 303:1517–25. doi: 10.1001/jama.2010.479
52. Irving WL, James DK, Stephenson T, Laing P, Jameson C, Oxford JS, et al. Influenza Virus Infection in the Second and Third Trimesters of Pregnancy: A Clinical and Seroepidemiological Study. BJOG (2000) 107:1282–9. doi: 10.1111/j.1471-0528.2000.tb11621.x
53. Littauer EQ, Esser ES, Antao OQ, Vassilieva EV, Compans RW, Skountzou I. H1N1 Influenza Virus Infection Results in Adverse Pregnancy Outcomes by Disrupting Tissue-Specific Hormonal Regulation. PloS Pathog (2017) 13:e1006757. doi: 10.1371/journal.ppat.1006757
54. Jacobsen H, Walendy-Gnirss K, Tekin-Bubenheim N, Kouassi NM, Ben-Batalla I, Berenbrok N, et al. Offspring Born to Influenza A Virus Infected Pregnant Mice Have Increased Susceptibility to Viral and Bacterial Infections in Early Life. Nat Commun (2021) 12:4957. doi: 10.1038/s41467-021-25220-3
55. Cdc. Flu & Pregnancy (2021). Available at: https://www.cdc.gov/flu/highrisk/pregnant.htm (Accessed 2022).
56. Juvet LK, Robertson AH, Laake I, Mjaaland S, Trogstad L. Safety of Influenza A H1n1pdm09 Vaccines: An Overview of Systematic Reviews. Front Immunol (2021) 12:740048. doi: 10.3389/fimmu.2021.740048
57. Thompson MG, Kwong JC, Regan AK, Katz MA, Drews SJ, Azziz-Baumgartner E, et al. Influenza Vaccine Effectiveness in Preventing Influenza-Associated Hospitalizations During Pregnancy: A Multi-Country Retrospective Test Negative Design Study 2010-2016. Clin Infect Dis (2019) 68:1444–53. doi: 10.1093/cid/ciy737
58. Schlaudecker EP, Steinhoff MC, Omer SB, Mcneal MM, Roy E, Arifeen SE, et al. Iga and Neutralizing Antibodies to Influenza a Virus in Human Milk: A Randomized Trial of Antenatal Influenza Immunization. PloS One (2013) 8:e70867. doi: 10.1371/journal.pone.0070867
59. Albrecht M, Pagenkemper M, Wiessner C, Spohn M, Lutgehetmann M, Jacobsen H, et al. Infant Immunity Against Viral Infections is Advanced by the Placenta-Dependent Vertical Transfer of Maternal Antibodies. Vaccine (2021) 40(11):1563–71. doi: 10.1016/j.vaccine.2020.12.049
60. Zaman K, Roy E, Arifeen SE, Rahman M, Raqib R, Wilson E, et al. Effectiveness of Maternal Influenza Immunization in Mothers and Infants. N Engl J Med (2008) 359:1555–64. doi: 10.1056/NEJMoa0708630
61. Benowitz I, Esposito DB, Gracey KD, Shapiro ED, Vazquez M. Influenza Vaccine Given to Pregnant Women Reduces Hospitalization Due to Influenza in Their Infants. Clin Infect Dis (2010) 51:1355–61. doi: 10.1086/657309
62. Regan AK, De Klerk N, Moore HC, Omer SB, Shellam G, Effler PV. Effect of Maternal Influenza Vaccination on Hospitalization for Respiratory Infections in Newborns: A Retrospective Cohort Study. Pediatr Infect Dis J (2016) 35:1097–103. doi: 10.1097/INF.0000000000001258
63. Nunes MC, Cutland CL, Jones S, Downs S, Weinberg A, Ortiz JR, et al. Efficacy of Maternal Influenza Vaccination Against All-Cause Lower Respiratory Tract Infection Hospitalizations in Young Infants: Results From a Randomized Controlled Trial. Clin Infect Dis (2017) 65:1066–71. doi: 10.1093/cid/cix497
64. Cowling BJ, Perera R, Fang VJ, Chu DKW, Hui APW, Yeung APC, et al. Maternal Antibodies Against Influenza in Cord Blood and Protection Against Laboratory-Confirmed Influenza in Infants. Clin Infect Dis (2020) 71:1741–8. doi: 10.1093/cid/ciz1058
65. Albrecht M, Pagenkemper M, Wiessner C, Spohn M, Lütgehetmann M, Jacobsen H, et al. Infant Immunity Against Viral Infections is Advanced by the Placenta-Dependent Vertical Transfer of Maternal Antibodies. Vaccine (2022) 40:1563–71. doi: 10.1016/j.vaccine.2020.12.049
66. Cdc. Cdc Seasonal Flu Vaccine Effectiveness Studies (2022). Available at: https://www.cdc.gov/flu/vaccines-work/effectiveness-studies.htm (Accessed 2022).
67. Roubidoux EK, Schultz-Cherry S. Animal Models Utilized for the Development of Influenza Virus Vaccines. Vaccines (Basel) (2021) 9(7):787. doi: 10.3390/vaccines9070787
68. Van Der Lubbe JEM, Vreugdenhil J, Damman S, Vaneman J, Klap J, Goudsmit J, et al. Maternal Antibodies Protect Offspring From Severe Influenza Infection and do Not Lead to Detectable Interference With Subsequent Offspring Immunization. Virol J (2017) 14:123. doi: 10.1186/s12985-017-0787-4
69. Murugesan A, Manoharan M. Dengue Virus. Emerg Reemerg Viral Pathog (2020) 281–359. doi: 10.1016/B978-0-12-819400-3.00016-8
70. Chen LH, Wilson ME. Transmission of Dengue Virus Without a Mosquito Vector: Nosocomial Mucocutaneous Transmission and Other Routes of Transmission. Clin Infect Dis (2004) 39:e56–60. doi: 10.1086/423807
71. Basurko C, Carles G, Youssef M, Guindi WE. Maternal and Fetal Consequences of Dengue Fever During Pregnancy. Eur J Obstet Gynecol Reprod Biol (2009) 147:29–32. doi: 10.1016/j.ejogrb.2009.06.028
72. Wiwanitkit V. Unusual Mode of Transmission of Dengue. J Infect Dev Ctries (2009) 4:51–4. doi: 10.3855/jidc.145
73. Arragain L, Dupont-Rouzeyrol M, O'connor O, Sigur N, Grangeon JP, Huguon E, et al. Vertical Transmission of Dengue Virus in the Peripartum Period and Viral Kinetics in Newborns and Breast Milk: New Data. J Pediatr Infect Dis Soc (2017) 6:324–31.
74. Basurko C, Matheus S, Hilderal H, Everhard S, Restrepo M, Cuadro-Alvarez E, et al. Estimating the Risk of Vertical Transmission of Dengue: A Prospective Study. Am J Trop Med Hyg (2018) 98:1826–32. doi: 10.4269/ajtmh.16-0794
75. Islam MT, Quispe C, Herrera-Bravo J, Sarkar C, Sharma R, Garg N, et al. Production, Transmission, Pathogenesis, and Control of Dengue Virus: A Literature-Based Undivided Perspective. BioMed Res Int (2021) 2021:4224816. doi: 10.1155/2021/4224816
76. Racherla RG, Pamireddy ML, Mohan A, Mudhigeti N, Mahalakshmi PA, Nallapireddy U, et al. Co-Circulation of Four Dengue Serotypes at South Eastern Andhra Pradesh, India: A Prospective Study. Indian J Med Microbiol (2018) 36:236–40. doi: 10.4103/ijmm.IJMM_18_109
77. Estofolete CF, Terzian ACB, Colombo TE, De Freitas Guimarães G, Ferraz HCJ, Da Silva RA, et al. Co-Infection Between Zika and Different Dengue Serotypes During DENV Outbreak in Brazil. J Infect Public Health (2019) 12:178–81. doi: 10.1016/j.jiph.2018.09.007
78. Ferreira-De-Lima VH, Lima-Camara TN. Natural Vertical Transmission of Dengue Virus in Aedes Aegypti and Aedes Albopictus: A Systematic Review. Parasit Vectors (2018) 11:77. doi: 10.1186/s13071-018-2643-9
79. Machado CR, Machado ES, Rohloff RD, Azevedo M, Campos DP, De Oliveira RB, et al. Is Pregnancy Associated With Severe Dengue? A Review of Data From the Rio De Janeiro Surveillance Information System. PloS Negl Trop Dis (2013) 7:e2217. doi: 10.1371/journal.pntd.0002217
80. Sondo KA, Ouattara A, Diendéré EA, Diallo I, Zoungrana J, Zémané G, et al. Dengue Infection During Pregnancy in Burkina Faso: A Cross-Sectional Study. BMC Infect Dis (2019) 19:997. doi: 10.1186/s12879-019-4587-x
81. Mulik V, Dad N, Buhmaid S. Dengue in Pregnancy: Review Article. Eur J Obstet Gynecol Reprod Biol (2021) 261:205–10. doi: 10.1016/j.ejogrb.2021.04.035
82. Jing Q, Wang M. Dengue Epidemiology. Global Health J (2019) 3:37–45. doi: 10.1016/j.glohj.2019.06.002
83. Bhatt P, Sabeena SP, Varma M, Arunkumar G. Current Understanding of the Pathogenesis of Dengue Virus Infection. Curr Microbiol (2021) 78:17–32. doi: 10.1007/s00284-020-02284-w
84. Tan PC, Rajasingam G, Devi S, Omar SZ. Dengue Infection in Pregnancy: Prevalence, Vertical Transmission, and Pregnancy Outcome. Obstet Gynecol (2008) 111:1111–7. doi: 10.1097/AOG.0b013e31816a49fc
85. Pouliot SH, Xiong X, Harville E, Paz-Soldan V, Tomashek KM, Breart G, et al. Maternal Dengue and Pregnancy Outcomes: A Systematic Review. Obstet Gynecol Surv (2010) 65:107–18. doi: 10.1097/OGX.0b013e3181cb8fbc
86. Vats A, Ho TC, Puc I, Chen YJ, Chang CH, Chien YW, et al. Evidence That Hematopoietic Stem Cells in Human Umbilical Cord Blood is Infectable by Dengue Virus: Proposing a Vertical Transmission Candidate. Heliyon (2021) 7:e06785. doi: 10.1016/j.heliyon.2021.e06785
87. Watanaveeradej V, Endy TP, Samakoses R, Kerdpanich A, Simasathien S, Polprasert N, et al. Transplacentally Transferred Maternal-Infant Antibodies to Dengue Virus. Am J Trop Med Hyg (2003) 69:123–8. doi: 10.4269/ajtmh.2003.69.123
88. Halstead SB, Lan NT, Myint TT, Shwe TN, Nisalak A, Kalyanarooj S, et al. Dengue Hemorrhagic Fever in Infants: Research Opportunities Ignored. Emerg Infect Dis (2002) 8:1474–9. doi: 10.3201/eid0812.020170
89. Katzelnick LC, Gresh L, Halloran ME, Mercado JC, Kuan G, Gordon A, et al. Antibody-Dependent Enhancement of Severe Dengue Disease in Humans. Sci (New York NY) (2017) 358:929–32. doi: 10.1126/science.aan6836
90. Narayan R, Tripathi S. Intrinsic ADE: The Dark Side of Antibody Dependent Enhancement During Dengue Infection. Front Cell Infect Microbiol (2020) 10:580096. doi: 10.3389/fcimb.2020.580096
91. Chau TN, Quyen NT, Thuy TT, Tuan NM, Hoang DM, Dung NT, et al. Dengue in Vietnamese Infants–Results of Infection-Enhancement Assays Correlate With Age-Related Disease Epidemiology, and Cellular Immune Responses Correlate With Disease Severity. J Infect Dis (2008) 198:516–24. doi: 10.1086/590117
92. Simmons CP, Chau TN, Thuy TT, Tuan NM, Hoang DM, Thien NT, et al. Maternal Antibody and Viral Factors in the Pathogenesis of Dengue Virus in Infants. J Infect Dis (2007) 196:416–24. doi: 10.1086/519170
93. Ribeiro CF, Lopes VG, Brasil P, Coelho J, Muniz AG, Nogueira RM. Perinatal Transmission of Dengue: A Report of 7 Cases. J Pediatr (2013) 163:1514–6. doi: 10.1016/j.jpeds.2013.06.040
94. Skipetrova A, Wartel TA, Gailhardou S. Dengue Vaccination During Pregnancy - An Overview of Clinical Trials Data. Vaccine (2018) 36:3345–50. doi: 10.1016/j.vaccine.2018.04.050
95. Zompi S, Harris E. Animal Models of Dengue Virus Infection. Viruses (2012) 4:62–82. doi: 10.3390/v4010062
96. Zellweger RM, Shresta S. Mouse Models to Study Dengue Virus Immunology and Pathogenesis. Front Immunol (2014) 5. doi: 10.3389/fimmu.2014.00151
97. Onlamoon N, Noisakran S, Hsiao HM, Duncan A, Villinger F, Ansari AA, et al. Dengue Virus-Induced Hemorrhage in a Nonhuman Primate Model. Blood (2010) 115:1823–34. doi: 10.1182/blood-2009-09-242990
98. Muhammad Azami NA, Takasaki T, Kurane I, Moi ML. Non-Human Primate Models of Dengue Virus Infection: A Comparison of Viremia Levels and Antibody Responses During Primary and Secondary Infection Among Old World and New World Monkeys. Pathog (Basel Switzerland) (2020) 9:247. doi: 10.3390/pathogens9040247
99. Jiang L, Lu C, Sun Q. Tree Shrew as a New Animal Model for the Study of Dengue Virus. Front Immunol (2021) 12. doi: 10.3389/fimmu.2021.621164
100. Bradley JH, Harrison A, Corey A, Gentry N, Gregg RK. Ebola Virus Secreted Glycoprotein Decreases the Anti-Viral Immunity of Macrophages in Early Inflammatory Responses. Cell Immunol (2018) 324:24–32. doi: 10.1016/j.cellimm.2017.11.009
101. Dhama K, Karthik K, Khandia R, Chakraborty S, Munjal A, Latheef SK, et al. Advances in Designing and Developing Vaccines, Drugs, and Therapies to Counter Ebola Virus. Front Immunol (2018) 9:1803. doi: 10.3389/fimmu.2018.01803
102. Brainard J, Hooper L, Pond K, Edmunds K, Hunter PR. Risk Factors for Transmission of Ebola or Marburg Virus Disease: A Systematic Review and Meta-Analysis. Int J Epidemiol (2016) 45:102–16. doi: 10.1093/ije/dyv307
103. Cdc. 2014-2016 Ebola Outbreak in West Africa (2019). Available at: https://www.cdc.gov/vhf/ebola/history/2014-2016-outbreak/index.html (Accessed 2022).
104. Feldmann H, Sprecher A, Geisbert TW. Ebola. N Engl J Med (2020) 382:1832–42. doi: 10.1056/NEJMra1901594
105. World Health Organization. Guidelines for the Management of Pregnant and Breastfeeding Women in the Context of Ebola Virus Disease Licence: Cc BY-NC-SA 3.0 Igo. Geneva: World Health Organization (2020).
106. Erland E, Dahl B. Midwives' Experiences of Caring for Pregnant Women Admitted to Ebola Centres in Sierra Leone. Midwifery (2017) 55:23–8. doi: 10.1016/j.midw.2017.08.005
107. Medina-Rivera M, Centeno-Tablante E, Finkelstein JL, Rayco-Solon P, Pena-Rosas JP, Garcia-Casal MN, et al. Presence of Ebola Virus in Breast Milk and Risk of Mother-to-Child Transmission: Synthesis of Evidence. Ann N Y Acad Sci (2021) 1488:33–43. doi: 10.1111/nyas.14519
108. Foeller ME, Carvalho Ribeiro Do Valle C, Foeller TM, Oladapo OT, Roos E, Thorson AE. Pregnancy and Breastfeeding in the Context of Ebola: A Systematic Review. Lancet Infect Dis (2020) 20:e149–58. doi: 10.1016/S1473-3099(20)30194-8
109. Bausch DG, Towner JS, Dowell SF, Kaducu F, Lukwiya M, Sanchez A, et al. Assessment of the Risk of Ebola Virus Transmission From Bodily Fluids and Fomites. J Infect Dis (2007) 196(Suppl 2):S142–7. doi: 10.1086/520545
110. Medina-Rivera M, Centeno-Tablante E, Finkelstein JL, Rayco-Solon P, Pena-Rosas JP, Garcia-Casal MN, et al. Presence of Ebola Virus in Breast Milk and Risk of Mother-to-Child Transmission: Synthesis of Evidence. Ann N Y Acad Sci (2020) 1488(1):33–43. doi: 10.1111/nyas.14519
111. Bausch DG, Towner JS, Dowell SF, Kaducu F, Lukwiya M, Sanchez A, et al. Assessment of the Risk of Ebola Virus Transmission From Bodily Fluids and Fomites. J Infect Dis (2007) 196:S142–7. doi: 10.1086/520545
112. Sissoko D, Keïta M, Diallo B, Aliabadi N, Fitter DL, Dahl BA, et al. Ebola Virus Persistence in Breast Milk After No Reported Illness: A Likely Source of Virus Transmission From Mother to Child. Clin Infect Dis (2017) 64:513–6. doi: 10.1093/cid/ciw793
113. Wolf J, Jannat R, Dubey S, Troth S, Onorato MT, Coller BA, et al. Development of Pandemic Vaccines: ERVEBO Case Study. Vaccines (Basel) (2021) 9(3):190. doi: 10.3390/vaccines9030190
114. Colavita F, Biava M, Castilletti C, Lanini S, Miccio R, Portella G, et al. Inflammatory and Humoral Immune Response During Ebola Virus Infection in Survivor and Fatal Cases Occurred in Sierra Leone During the 2014(-)2016 Outbreak in West Africa. Viruses (2019) 11(4):373. doi: 10.3390/v11040373
115. Legardy-Williams JK, Carter RJ, Goldstein ST, Jarrett OD, Szefer E, Fombah AE, et al. Pregnancy Outcomes Among Women Receiving Rvsvdelta-ZEBOV-GP Ebola Vaccine During the Sierra Leone Trial to Introduce a Vaccine Against Ebola. Emerg Infect Dis (2020) 26:541–8. doi: 10.3201/eid2603.191018
116. Nih. A Study of a 2-Dose Ebola Vaccine Regimen of Ad26.ZEBOV Followed by MVA-BN-Filo in Healthy Pregnant Women (Ingabo) (2020). Available at: https://clinicaltrials.gov/ct2/show/NCT04556526 (Accessed 2021).
117. Marzi A, Jankeel A, Menicucci AR, Callison J, O'donnell KL, Feldmann F, et al. Single Dose of a VSV-Based Vaccine Rapidly Protects Macaques From Marburg Virus Disease. Front Immunol (2021) 12:774026. doi: 10.3389/fimmu.2021.774026
118. Shifflett K, Marzi A. Marburg Virus Pathogenesis - Differences and Similarities in Humans and Animal Models. Virol J (2019) 16:165. doi: 10.1186/s12985-019-1272-z
119. Bennett RS, Logue J, Liu DX, Reeder RJ, Janosko KB, Perry DL, et al. Kikwit Ebola Virus Disease Progression in the Rhesus Monkey Animal Model. Viruses (2020) 12(7):753. doi: 10.3390/v12070753
120. Bradfute SB, Dye JM Jr., Bavari S. Filovirus Vaccines. Hum Vaccin (2011) 7:701–11. doi: 10.4161/hv.7.6.15398
121. German Advisory Committee Blood, S.a.O.P.T.B.B. Human Immunodeficiency Virus (Hiv). Transfus Med Hemother (2016) 43:203–22. doi: 10.1159/000445852
122. Unaids. Global Hiv & AIDS Statistics — Fact Sheet. Available at: https://www.unaids.org/en/resources/fact-sheet.
123. Who. Hiv/Aids (2021). Available at: https://www.who.int/news-room/fact-sheets/detail/hiv-aids (Accessed 2022).
124. Unaids. Get on the Fast-Track: The Life-Cycle Approach to HIV (2016). Available at: http://www.unaids.org/sites/default/files/media_asset/Get-on-the-Fast-Track_en.pdf.
125. Nesheim SR, Fitzharris LF, Mahle Gray K, Lampe MA. Epidemiology of Perinatal Hiv Transmission in the United States in the Era of Its Elimination. Pediatr Infect Dis J (2019) 38:611–6. doi: 10.1097/INF.0000000000002290
127. Kourtis AP, Butera S, Ibegbu C, Belec L, Duerr A. Breast Milk and HIV-1: Vector of Transmission or Vehicle of Protection? Lancet Infect Dis (2003) 3:786–93. doi: 10.1016/S1473-3099(03)00832-6
128. Barlow-Mosha L, Eckard AR, Mccomsey GA, Musoke PM. Metabolic Complications and Treatment of Perinatally HIV-infected Children and Adolescents. J Int AIDS Soc (2013) 16:18600. doi: 10.7448/IAS.16.1.18600
129. Boswell R, Foisy MM, Hughes CA. Dolutegravir Dual Therapy as Maintenance Treatment in HIV-Infected Patients: A Review. Ann Pharmacother (2018) 52:681–9. doi: 10.1177/1060028018758432
130. Powis KM, Kitch D, Ogwu A, Hughes MD, Lockman S, Leidner J, et al. Increased Risk of Preterm Delivery Among HIV-infected Women Randomized to Protease Versus Nucleoside Reverse Transcriptase Inhibitor-Based HAART During Pregnancy. J Infect Dis (2011) 204:506–14. doi: 10.1093/infdis/jir307
131. Jao J, Abrams EJ. Metabolic Complications of In Utero Maternal HIV and Antiretroviral Exposure in HIV-exposed Infants. Pediatr Infect Dis J (2014) 33:734–40. doi: 10.1097/INF.0000000000000224
132. Richardson BA, Nduati R, Mbori-Ngacha D, Overbaugh J, John-Stewart GC. Acute HIV Infection Among Kenyan Infants. Clin Infect Dis (2008) 46:289–95. doi: 10.1086/524748
133. Avert. Prevention of Mother-to-Child Transmission (PMTCT) of Hiv (2018). Available at: https://www.avert.org/printpdf/node/375#:~:text=Prevention%20of%20mother%2Dto%2Dchild%20transmission%20(PMTCT)%20programmes,throughout%20pregnancy%2C%20labour%20and%20breastfeeding (Accessed 2022).
134. Ellington SR, King CC, Kourtis AP. Host Factors That Influence Mother-to-Child Transmission of HIV-1: Genetics, Coinfections, Behavior and Nutrition. Future Virol (2011) 6:1451–69. doi: 10.2217/fvl.11.119
135. Permar SR, Fong Y, Vandergrift N, Fouda GG, Gilbert P, Parks R, et al. Maternal HIV-1 Envelope-Specific Antibody Responses and Reduced Risk of Perinatal Transmission. J Clin Invest (2015) 125:2702–6. doi: 10.1172/JCI81593
136. Martinez DR, Vandergrift N, Douglas AO, Mcguire E, Bainbridge J, Nicely NI, et al. Maternal Binding and Neutralizing Igg Responses Targeting the C-Terminal Region of the V3 Loop Are Predictive of Reduced Peripartum HIV-1 Transmission Risk. J Virol (2017) 91(9):e02422–16. doi: 10.1128/JVI.02422-16
137. Diomede L, Nyoka S, Pastori C, Scotti L, Zambon A, Sherman G, et al. Passively Transmitted gp41 Antibodies in Babies Born From HIV-1 Subtype C-seropositive Women: Correlation Between Fine Specificity and Protection. J Virol (2012) 86:4129–38. doi: 10.1128/JVI.06359-11
138. Naiman NE, Slyker J, Nduati R, Overbaugh JM. Maternal Envelope Gp41 Ectodomain-Specific Antibodies Are Associated With Increased Mother-to-Child Transmission of Human Immunodeficiency Virus-1. J Infect Dis (2020) 221:232–7. doi: 10.1093/infdis/jiz444
139. Mutucumarana CP, Eudailey J, Mcguire EP, Vandergrift N, Tegha G, Chasela C, et al. Maternal Humoral Immune Correlates of Peripartum Transmission of Clade C HIV-1 in the Setting of Peripartum Antiretrovirals. Clin Vaccine Immunol (2017) 24(8):e00062–17. doi: 10.1128/CVI.00062-17
140. Dickover R, Garratty E, Yusim K, Miller C, Korber B, Bryson Y. Role of Maternal Autologous Neutralizing Antibody in Selective Perinatal Transmission of Human Immunodeficiency Virus Type 1 Escape Variants. J Virol (2006) 80:6525–33. doi: 10.1128/JVI.02658-05
141. Wu X, Parast AB, Richardson BA, Nduati R, John-Stewart G, Mbori-Ngacha D, et al. Neutralization Escape Variants of Human Immunodeficiency Virus Type 1 are Transmitted From Mother to Infant. J Virol (2006) 80:835–44. doi: 10.1128/JVI.80.2.835-844.2006
142. Goo L, Milligan C, Simonich CA, Nduati R, Overbaugh J. Neutralizing Antibody Escape During HIV-1 Mother-to-Child Transmission Involves Conformational Masking of Distal Epitopes in Envelope. J Virol (2012) 86:9566–82. doi: 10.1128/JVI.00953-12
143. Kumar A, Smith CEP, Giorgi EE, Eudailey J, Martinez DR, Yusim K, et al. Infant Transmitted/Founder HIV-1 Viruses From Peripartum Transmission are Neutralization Resistant to Paired Maternal Plasma. PloS Pathog (2018) 14:e1006944. doi: 10.1371/journal.ppat.1006944
144. Martinez DR, Tu JJ, Kumar A, Mangold JF, Mangan RJ, Goswami R, et al. Maternal Broadly Neutralizing Antibodies can Select for Neutralization-Resistant, Infant-Transmitted/Founder HIV Variants. mBio (2020) 11:e00176–00120. doi: 10.1128/mBio.00176-20
145. Ghulam-Smith M, Olson A, White LF, Chasela CS, Ellington SR, Kourtis AP, et al. Maternal But Not Infant Anti-HIV-1 Neutralizing Antibody Response Associates With Enhanced Transmission and Infant Morbidity. mBio (2017) 8(5):e01373–17. doi: 10.1128/mBio.01373-17
146. Mabuka J, Nduati R, Odem-Davis K, Peterson D, Overbaugh J. HIV-Specific Antibodies Capable of ADCC are Common in Breastmilk and are Associated With Reduced Risk of Transmission in Women With High Viral Loads. PloS Pathog (2012) 8:e1002739. doi: 10.1371/journal.ppat.1002739
147. Pollara J, Mcguire E, Fouda GG, Rountree W, Eudailey J, Overman RG, et al. Association of HIV-1 Envelope-Specific Breast Milk IgA Responses With Reduced Risk of Postnatal Mother-to-Child Transmission of HIV-1. J Virol (2015) 89:9952–61. doi: 10.1128/JVI.01560-15
148. Milligan C, Richardson BA, John-Stewart G, Nduati R, Overbaugh J. Passively Acquired Antibody-Dependent Cellular Cytotoxicity (ADCC) Activity in HIV-infected Infants is Associated With Reduced Mortality. Cell Host Microbe (2015) 17:500–6. doi: 10.1016/j.chom.2015.03.002
149. Yaffe ZA, Naiman NE, Slyker J, Wines BD, Richardson BA, Hogarth PM, et al. Improved HIV-positive Infant Survival is Correlated With High Levels of HIV-specific ADCC Activity in Multiple Cohorts. Cell Rep Med (2021) 2:100254. doi: 10.1016/j.xcrm.2021.100254
150. Wright PF, Lambert JS, Gorse GJ, Hsieh RH, Mcelrath MJ, Weinhold K, et al. Immunization With Envelope MN rgp120 Vaccine in Human Immunodeficiency Virus-Infected Pregnant Women. J Infect Dis (1999) 180:1080–8. doi: 10.1086/314985
151. Hompe ED, Mangold JF, Kumar A, Eudailey JA, Mcguire E, Haynes BF, et al. Induction of Neutralizing Responses Against Autologous Virus in Maternal Hiv Vaccine Trials. mSphere (2020) 5(3):e00254–20. doi: 10.1128/mSphere.00254-20
152. Jayaraman P, Haigwood NL. Animal Models for Perinatal Transmission of HIV-1. Front Biosci (2006) 11:2828–44. doi: 10.2741/2012
153. Abel K. The Rhesus Macaque Pediatric SIV Infection Model - a Valuable Tool in Understanding Infant HIV-1 Pathogenesis and for Designing Pediatric HIV-1 Prevention Strategies. Curr HIV Res (2009) 7:2–11. doi: 10.2174/157016209787048528
154. Garcia-Tellez T, Huot N, Ploquin MJ, Rascle P, Jacquelin B, Muller-Trutwin M. Non-Human Primates in HIV Research: Achievements, Limits and Alternatives. Infect Genet Evol (2016) 46:324–32. doi: 10.1016/j.meegid.2016.07.012
155. Goswami R, Nelson AN, Tu JJ, Dennis M, Feng L, Kumar A, et al. Analytical Treatment Interruption After Short-Term Antiretroviral Therapy in a Postnatally Simian-Human Immunodeficiency Virus-Infected Infant Rhesus Macaque Model. mBio (2019) 10(5):e01971–19. doi: 10.1128/mBio.01971-19
156. Himes JE, Goswami R, Mangan RJ, Kumar A, Jeffries TL Jr., Eudailey JA, et al. Polyclonal HIV Envelope-Specific Breast Milk Antibodies Limit Founder SHIV Acquisition and Cell-Associated Virus Loads in Infant Rhesus Monkeys. Mucosal Immunol (2018) 11:1716–26. doi: 10.1038/s41385-018-0067-7
157. Phillips B, Fouda GG, Eudailey J, Pollara J, Curtis AD 2nd, Kunz E, et al. Impact of Poxvirus Vector Priming, Protein Coadministration, and Vaccine Intervals on HIV Gp120 Vaccine-Elicited Antibody Magnitude and Function in Infant Macaques. Clin Vaccine Immunol (2017) 24(10):e00231-17. doi: 10.1128/CVI.00231-17
158. Phillips B, Van Rompay KKA, Rodriguez-Nieves J, Lorin C, Koutsoukos M, Tomai M, et al. Adjuvant-Dependent Enhancement of HIV Env-Specific Antibody Responses in Infant Rhesus Macaques. J Virol (2018) 92(20):e01051-18. doi: 10.1128/JVI.01051-18
159. Ruprecht RM, Hofmann-Lehmann R, Smith-Franklin BA, Rasmussen RA, Liska V, Vlasak J, et al. Protection of Neonatal Macaques Against Experimental SHIV Infection by Human Neutralizing Monoclonal Antibodies. Transfus Clin Biol (2001) 8:350–8. doi: 10.1016/S1246-7820(01)00187-2
160. Shapiro MB, Cheever T, Malherbe DC, Pandey S, Reed J, Yang ES, et al. Single-Dose bNAb Cocktail or Abbreviated ART Post-Exposure Regimens Achieve Tight SHIV Control Without Adaptive Immunity. Nat Commun (2020) 11:70. doi: 10.1038/s41467-019-13972-y
161. Rosenberg YJ, Jiang X, Cheever T, Coulter FJ, Pandey S, Sack M, et al. Protection of Newborn Macaques by Plant-Derived Hiv Broadly Neutralizing Antibodies: A Model for Passive Immunotherapy During Breastfeeding. J Virol (2021) 95:e0026821. doi: 10.1128/JVI.00268-21
162. Sirohi D, Kuhn RJ. Zika Virus Structure, Maturation, and Receptors. J Infect Dis (2017) 216:S935–s944. doi: 10.1093/infdis/jix515
163. Who. Zika Virus (2018). Available at: https://www.who.int/news-room/fact-sheets/detail/zika-virus (Accessed 2022).
164. Sakkas H, Economou V, Papadopoulou C. Zika Virus Infection: Past and Present of Another Emerging Vector-Borne Disease. J Vector Borne Dis (2016) 53:305–11.
165. Lowe R, Barcellos C, Brasil P, Cruz OG, Honório NA, Kuper H, et al. The Zika Virus Epidemic in Brazil: From Discovery to Future Implications. Int J Environ Res Public Health (2018) 15:96. doi: 10.3390/ijerph15010096
166. Cdc. Data and Statistics on Zika and Pregnancy (2022). Available at: https://www.cdc.gov/pregnancy/zika/data/index.html (Accessed 2022).
167. Cdc. Zika Transmission (2019). Available at: https://www.cdc.gov/zika/prevention/transmission-methods.html (Accessed 2022).
168. Duffy MR, Chen T-H, Hancock WT, Powers AM, Kool JL, Lanciotti RS, et al. Zika Virus Outbreak on Yap Island, Federated States of Micronesia. New Engl J Med (2009) 360:2536–43. doi: 10.1056/NEJMoa0805715
169. Polonio CM, De Freitas CL, Zanluqui NG, Peron JPS. Zika Virus Congenital Syndrome: Experimental Models and Clinical Aspects. J Venomous Anim Toxins including Trop Dis (2017) 23:41. doi: 10.1186/s40409-017-0131-x
170. Reynolds MR, Jones AM, Petersen EE, Lee EH, Rice ME, Bingham A, et al. Vital Signs: Update on Zika Virus-Associated Birth Defects and Evaluation of All U.s. Infants With Congenital Zika Virus Exposure - U.S. Zika Pregnancy Registry 2016. MMWR Morb Mortal Wkly Rep (2017) 66:366–73. doi: 10.15585/mmwr.mm6613e1
171. Rasmussen SA, Jamieson DJ, Honein MA, Petersen LR. Zika Virus and Birth Defects — Reviewing the Evidence for Causality. N Engl J Med (2016) 374:1981–7. doi: 10.1056/NEJMsr1604338
172. Lin HZ, Tambyah PA, Yong EL, Biswas A, Chan SY. A Review of Zika Virus Infections in Pregnancy and Implications for Antenatal Care in Singapore. Singapore Med J (2017) 58:171–8. doi: 10.11622/smedj.2017026
173. Centeno-Tablante E, Medina-Rivera M, Finkelstein JL, Herman HS, Rayco-Solon P, Garcia-Casal MN, et al. Update on the Transmission of Zika Virus Through Breast Milk and Breastfeeding: A Systematic Review of the Evidence. Viruses (2021) 13(1):123. doi: 10.3390/v13010123
174. Abbink P, Larocca RA, de la Barrera RA, Bricault CA, Moseley ET, Boyd M, et al. Protective Efficacy of Multiple Vaccine Platforms Against Zika Virus Challenge in Rhesus Monkeys. Science (2016) 353:1129–32. doi: 10.1126/science.aah6157
175. Larocca RA, Abbink P, Peron JP, Zanotto PM, Iampietro MJ, Badamchi-Zadeh A, et al. Vaccine Protection Against Zika Virus From Brazil. Nature (2016) 536:474–8. doi: 10.1038/nature18952
176. Singh T, Lopez CA, Giuberti C, Dennis ML, Itell HL, Heimsath HJ, et al. Efficient Transplacental IgG Transfer in Women Infected With Zika Virus During Pregnancy. PloS Negl Trop Dis (2019) 13:e0007648. doi: 10.1371/journal.pntd.0007648
177. Stettler K, Beltramello M, Espinosa DA, Graham V, Cassotta A, Bianchi S, et al. Specificity, Cross-Reactivity, and Function of Antibodies Elicited by Zika Virus Infection. Science (2016) 353:823–6. doi: 10.1126/science.aaf8505
178. Dejnirattisai W, Supasa P, Wongwiwat W, Rouvinski A, Barba-Spaeth G, Duangchinda T, et al. Dengue Virus Sero-Cross-Reactivity Drives Antibody-Dependent Enhancement of Infection With Zika Virus. Nat Immunol (2016) 17:1102–8. doi: 10.1038/ni.3515
179. Bardina SV, Bunduc P, Tripathi S, Duehr J, Frere JJ, Brown JA, et al. Enhancement of Zika Virus Pathogenesis by Preexisting Antiflavivirus Immunity. Science (2017) 356:175–80. doi: 10.1126/science.aal4365
180. Narasimhan H, Chudnovets A, Burd I, Pekosz A, Klein SL. Animal Models of Congenital Zika Syndrome Provide Mechanistic Insight Into Viral Pathogenesis During Pregnancy. PloS Negl Trop Dis (2020) 14:e0008707. doi: 10.1371/journal.pntd.0008707
181. Lazear HM, Govero J, Smith AM, Platt DJ, Fernandez E, Miner JJ, et al. A Mouse Model of Zika Virus Pathogenesis. Cell Host Microbe (2016) 19:720–30. doi: 10.1016/j.chom.2016.03.010
182. Rossi SL, Tesh RB, Azar SR, Muruato AE, Hanley KA, Auguste AJ, et al. Characterization of a Novel Murine Model to Study Zika Virus. Am J Trop Med Hyg (2016) 94:1362–9. doi: 10.4269/ajtmh.16-0111
183. Hemberger M, Hanna CW, Dean W. Mechanisms of Early Placental Development in Mouse and Humans. Nat Rev Genet (2020) 21:27–43. doi: 10.1038/s41576-019-0169-4
184. Dick GW. Epidemiological Notes on Some Viruses Isolated in Uganda; Yellow Fever, Rift Valley Fever, Bwamba Fever, West Nile, Mengo, Semliki Forest, Bunyamwera, Ntaya, Uganda S and Zika Viruses. Trans R Soc Trop Med Hyg (1953) 47:13–48. doi: 10.1016/0035-9203(53)90021-2
185. Coffey LL, Keesler RI, Pesavento PA, Woolard K, Singapuri A, Watanabe J, et al. Intraamniotic Zika Virus Inoculation of Pregnant Rhesus Macaques Produces Fetal Neurologic Disease. Nat Commun (2018) 9:2414. doi: 10.1038/s41467-018-04777-6
186. Nguyen SM, Antony KM, Dudley DM, Kohn S, Simmons HA, Wolfe B, et al. Highly Efficient Maternal-Fetal Zika Virus Transmission in Pregnant Rhesus Macaques. PloS Pathog (2017) 13:e1006378. doi: 10.1371/journal.ppat.1006378
187. Mohr EL, Block LN, Newman CM, Stewart LM, Koenig M, Semler M, et al. Ocular and Uteroplacental Pathology in a Macaque Pregnancy With Congenital Zika Virus Infection. PloS One (2018) 13:e0190617. doi: 10.1371/journal.pone.0190617
188. Van Rompay KKA, Keesler RI, Ardeshir A, Watanabe J, Usachenko J, Singapuri A, et al. DNA Vaccination Before Conception Protects Zika Virus-Exposed Pregnant Macaques Against Prolonged Viremia and Improves Fetal Outcomes. Sci Transl Med (2019) 11(523):eaay2736. doi: 10.1126/scitranslmed.aay2736
189. Zuhair M, Smit GSA, Wallis G, Jabbar F, Smith C, Devleesschauwer B, et al. Estimation of the Worldwide Seroprevalence of Cytomegalovirus: A Systematic Review and Meta-Analysis. Rev Med Virol (2019) 29:e2034. doi: 10.1002/rmv.2034
190. Arens R, Remmerswaal EB, Bosch JA, Van Lier RA. 5(Th) International Workshop on CMV and Immunosenescence - A Shadow of Cytomegalovirus Infection on Immunological Memory. Eur J Immunol (2015) 45:954–7. doi: 10.1002/eji.201570044
191. Winter JR, Taylor GS, Thomas OG, Jackson C, Lewis JEA, Stagg HR. Factors Associated With Cytomegalovirus Serostatus in Young People in England: A Cross-Sectional Study. BMC Infect Dis (2020) 20:875. doi: 10.1186/s12879-020-05572-9
192. Cdc. Cytomegalovirus (CMV) and Congenital Cmv Infection (2020). Available at: https://www.cdc.gov/cmv/index.html (Accessed 2022).
193. Stagno S, Pass RF, Cloud G, Britt WJ, Henderson RE, Walton PD, et al. Primary Cytomegalovirus Infection in Pregnancy: Incidence, Transmission to Fetus, and Clinical Outcome. JAMA (1986) 256:1904–8. doi: 10.1001/jama.1986.03380140074025
194. Kenneson A, Cannon MJ. Review and Meta-Analysis of the Epidemiology of Congenital Cytomegalovirus (CMV) Infection. Rev Med Virol (2007) 17:253–76. doi: 10.1002/rmv.535
195. Wang C, Zhang X, Bialek S, Cannon MJ. Attribution of Congenital Cytomegalovirus Infection to Primary Versus non-Primary Maternal Infection. Clin Infect Dis (2011) 52:e11–13. doi: 10.1093/cid/ciq085
196. Gerna G, Lilleri D. Human Cytomegalovirus (HCMV) Infection/Re-Infection: Development of a Protective HCMV Vaccine. N Microbiol (2019) 42:1–20.
197. Neill L, Peggs K. Cell Therapy for Cytomegalovirus Infection. Expert Opin Biol Ther (2021) 21:649–59. doi: 10.1080/14712598.2021.1857720
198. Pesch MH, Saunders NA, Abdelnabi S. Cytomegalovirus Infection in Pregnancy: Prevention, Presentation, Management and Neonatal Outcomes. J Midwifery Womens Health (2021) 66:397–402. doi: 10.1111/jmwh.13228
199. Akpan US, Pillarisetty LS. Congenital Cytomegalovirus Infection. Treasure Island, FL: StatPearls (2021).
200. Konopka W, Smiechura-Ganczarczyk M, Pepas R. Cytomegalovirus Infections in Pregnant Women as a Risk of Congenital Deafness in a Child. Prz Menopauzalny (2021) 20:122–6. doi: 10.5114/pm.2021.109391
201. Pessoa FS, Goncalves VC, Lacerda E. Haemophagocytic Lymphohistiocytosis Secondary to Intrauterine Cytomegalovirus Infection. Rev Inst Med Trop Sao Paulo (2021) 63:e15. doi: 10.1590/s1678-9946202163015
202. Ross DS, Dollard SC, Victor M, Sumartojo E, Cannon MJ. The Epidemiology and Prevention of Congenital Cytomegalovirus Infection and Disease: Activities of the Centers for Disease Control and Prevention Workgroup. J Womens Health (Larchmt) (2006) 15:224–9. doi: 10.1089/jwh.2006.15.224
203. Dollard SC, Grosse SD, Ross DS. New Estimates of the Prevalence of Neurological and Sensory Sequelae and Mortality Associated With Congenital Cytomegalovirus Infection. Rev Med Virol (2007) 17:355–63. doi: 10.1002/rmv.544
204. Richardson BA, John-Stewart G, Atkinson C, Nduati R, Asbjornsdottir K, Boeckh M, et al. Vertical Cytomegalovirus Transmission From Hiv-Infected Women Randomized to Formula-Feed or Breastfeed Their Infants. J Infect Dis (2016) 213:992–8. doi: 10.1093/infdis/jiv515
205. Kadambari S, Whittaker E, Lyall H. Postnatally Acquired Cytomegalovirus Infection in Extremely Premature Infants: How Best to Manage? Arch Dis Child Fetal Neonatal Ed (2020) 105:334–9. doi: 10.1136/archdischild-2019-317650
206. Asanuma H, Numazaki K, Nagata N, Hotsubo T, Horino K, Chiba S. Role of Milk Whey in the Transmission of Human Cytomegalovirus Infection by Breast Milk. Microbiol Immunol (1996) 40:201–4. doi: 10.1111/j.1348-0421.1996.tb03335.x
207. Hamprecht K, Maschmann J, Vochem M, Dietz K, Speer CP, Jahn G. Epidemiology of Transmission of Cytomegalovirus From Mother to Preterm Infant by Breastfeeding. Lancet (2001) 357:513–8. doi: 10.1016/S0140-6736(00)04043-5
208. Maschmann J, Hamprecht K, Dietz K, Jahn G, Speer CP. Cytomegalovirus Infection of Extremely Low-Birth Weight Infants Via Breast Milk. Clin Infect Dis (2001) 33:1998–2003. doi: 10.1086/324345
209. Twite N, Andrei G, Kummert C, Donner C, Perez-Morga D, De Vos R, et al. Sequestration of Human Cytomegalovirus by Human Renal and Mammary Epithelial Cells. Virology (2014) 460-461:55–65. doi: 10.1016/j.virol.2014.04.032
210. Maschmann J, Goelz R, Witzel S, Strittmatter U, Steinmassl M, Jahn G, et al. Characterization of Human Breast Milk Leukocytes and Their Potential Role in Cytomegalovirus Transmission to Newborns. Neonatology (2015) 107:213–9. doi: 10.1159/000371753
211. Martins-Celini FP, Yamamoto AY, Passos DM, Do Nascimento SD, Lima EV, Di Giovanni CM, et al. Incidence, Risk Factors, and Morbidity of Acquired Postnatal Cytomegalovirus Infection Among Preterm Infants Fed Maternal Milk in a Highly Seropositive Population. Clin Infect Dis (2016) 63:929–36. doi: 10.1093/cid/ciw394
212. Lanari M, Sogno Valin P, Natale F, Capretti MG, Serra L. Human Milk, A Concrete Risk for Infection?. J Matern Fetal Neonatal Med (2012) 25(Suppl 4):75–7. doi: 10.3109/14767058.2012.715009
213. Stagno S, Pass RF, Cloud G, Britt WJ, Henderson RE, Walton PD, et al. Primary Cytomegalovirus Infection in Pregnancy. Incidence, Transmission to Fetus, and Clinical Outcome. JAMA (1986) 256:1904–8. doi: 10.1001/jama.1986.03380140074025
214. Nigro G, Adler SP, La Torre R, Best AM, Congenital Cytomegalovirus Collaborating G. Passive Immunization During Pregnancy for Congenital Cytomegalovirus Infection. N Engl J Med (2005) 353:1350–62. doi: 10.1056/NEJMoa043337
215. Revello MG, Lazzarotto T, Guerra B, Spinillo A, Ferrazzi E, Kustermann A, et al. A Randomized Trial of Hyperimmune Globulin to Prevent Congenital Cytomegalovirus. N Engl J Med (2014) 370:1316–26. doi: 10.1056/NEJMoa1310214
216. Society for Maternal-Fetal, M, Hughes BL, Gyamfi-Bannerman C. Diagnosis and Antenatal Management of Congenital Cytomegalovirus Infection. Am J Obstet Gynecol (2016) 214:B5–B11. doi: 10.1016/j.ajog.2016.02.042
217. Pass RF, Fowler KB, Boppana SB, Britt WJ, Stagno S. Congenital Cytomegalovirus Infection Following First Trimester Maternal Infection: Symptoms at Birth and Outcome. J Clin Virol (2006) 35:216–20. doi: 10.1016/j.jcv.2005.09.015
218. Lazar K, Rabe T, Goelz R, Hamprecht K. Human Cytomegalovirus Reactivation During Lactation: Impact of Antibody Kinetics and Neutralization in Blood and Breast Milk. Nutrients (2020) 12(2):338. doi: 10.3390/nu12020338
219. Saccoccio FM, Jenks JA, Itell HL, Li SH, Berry M, Pollara J, et al. Humoral Immune Correlates for Prevention of Postnatal Cytomegalovirus Acquisition. J Infect Dis (2019) 220:772–80. doi: 10.1093/infdis/jiz192
220. Osterholm EA, Schleiss MR. Impact of Breast Milk-Acquired Cytomegalovirus Infection in Premature Infants: Pathogenesis, Prevention, and Clinical Consequences? Rev Med Virol (2020) 30:1–11. doi: 10.1002/rmv.2117
221. Scarpini S, Morigi F, Betti L, Dondi A, Biagi C, Lanari M. Development of a Vaccine Against Human Cytomegalovirus: Advances, Barriers, and Implications for the Clinical Practice. Vaccines (Basel) (2021) 9(6):551. doi: 10.3390/vaccines9060551
222. Nelson CS, Huffman T, Jenks JA, Cisneros De La Rosa E, Xie G, Vandergrift N, et al. HCMV Glycoprotein B Subunit Vaccine Efficacy Mediated by Nonneutralizing Antibody Effector Functions. Proc Natl Acad Sci USA (2018) 115:6267–72. doi: 10.1073/pnas.1800177115
223. Jenks JA, Goodwin ML, Permar SR. The Roles of Host and Viral Antibody Fc Receptors in Herpes Simplex Virus (HSV) and Human Cytomegalovirus (Hcmv) Infections and Immunity. Front Immunol (2019) 10:2110. doi: 10.3389/fimmu.2019.02110
224. Diamond DJ, La Rosa C, Chiuppesi F, Contreras H, Dadwal S, Wussow F, et al. A Fifty-Year Odyssey: Prospects for a Cytomegalovirus Vaccine in Transplant and Congenital Infection. Expert Rev Vaccines (2018) 17:889–911. doi: 10.1080/14760584.2018.1526085
225. Clinicaltrials.Gov. Safety, Reactogenicity, and Immunogenicity of Cytomegalovirus Vaccines mRNA-1647 and mRNA-1443 in Healthy Adults . Available at: https://clinicaltrials.gov/ct2/show/NCT03382405.
226. Clinicaltrials.Gov. Dose-Finding Trial to Evaluate the Safety and Immunogenicity of Cytomegalovirus (CMV) Vaccine mRNA-1647 in Healthy Adults. https://clinicaltrials.gov/ct2/show/NCT04232280.
227. Clinicaltrials.Gov. A Study to Evaluate the Efficacy, Safety, and Immunogenicity of Mrna-1647 Cytomegalovirus (Cmv) Vaccine in Healthy Participants 16 to 40 Years of Age . Available at: https://clinicaltrials.gov/ct2/show/NCT05085366.
228. Gerna G, Sarasini A, Patrone M, Percivalle E, Fiorina L, Campanini G, et al. Human Cytomegalovirus Serum Neutralizing Antibodies Block Virus Infection of Endothelial/Epithelial Cells, But Not Fibroblasts, Early During Primary Infection. J Gen Virol (2008) 89:853–65. doi: 10.1099/vir.0.83523-0
229. Genini E, Percivalle E, Sarasini A, Revello MG, Baldanti F, Gerna G. Serum Antibody Response to the gH/gL/pUL128–131 Five-Protein Complex of Human Cytomegalovirus (HCMV) in Primary and Reactivated HCMV Infections. J Clin Virol (2011) 52:113–8. doi: 10.1016/j.jcv.2011.06.018
230. Britt WJ. Neutralizing Antibodies Detect a Disulfide-Linked Glycoprotein Complex Within the Envelope of Human Cytomegalovirus. Virology (1984) 135:369–78. doi: 10.1016/0042-6822(84)90193-4
231. Britt WJ, Vugler L, Stephens EB. Induction of Complement-Dependent and -Independent Neutralizing Antibodies by Recombinant-Derived Human Cytomegalovirus gp55-116 (Gb). J Virol (1988) 62:3309–18. doi: 10.1128/jvi.62.9.3309-3318.1988
232. Jackson JW, Sparer T. There Is Always Another Way! Cytomegalovirus' Multifaceted Dissemination Schemes. Viruses (2018) 10(7):383. doi: 10.3390/v10070383
233. Griffith BP, Lucia HL, Hsiung GD. Brain and Visceral Involvement During Congenital Cytomegalovirus Infection of Guinea Pigs. Pediatr Res (1982) 16:455–9. doi: 10.1203/00006450-198206000-00010
234. Woolf NK, Koehrn FJ, Harris JP, Richman DD. Congenital Cytomegalovirus Labyrinthitis and Sensorineural Hearing Loss in Guinea Pigs. J Infect Dis (1989) 160:929–37. doi: 10.1093/infdis/160.6.929
235. Woolf NK. Guinea Pig Model of Congenital CMV-induced Hearing Loss: A Review. Transplant Proc (1991) 23:32–4.
236. Park AH, Gifford T, Schleiss MR, Dahlstrom L, Chase S, Mcgill L, et al. Development of Cytomegalovirus-Mediated Sensorineural Hearing Loss in a Guinea Pig Model. Arch Otolaryngol Head Neck Surg (2010) 136:48–53. doi: 10.1001/archoto.2009.210
237. Roark HK, Jenks JA, Permar SR, Schleiss MR. Animal Models of Congenital Cytomegalovirus Transmission: Implications for Vaccine Development. J Infect Dis (2020) 221:S60–73. doi: 10.1093/infdis/jiz484
238. Li M, Brokaw A, Furuta AM, Coler B, Obregon-Perko V, Chahroudi A, et al. Non-Human Primate Models to Investigate Mechanisms of Infection-Associated Fetal and Pediatric Injury, Teratogenesis and Stillbirth. Front Genet (2021) 12:680342. doi: 10.3389/fgene.2021.680342
239. Webster H, Valencia S, Kumar A, Chan C, Dennis M, Roark H, et al. Pre-Existing Immunity to Cytomegalovirus in Macaques Influences Human CMV Vaccine Responses in Preclinical Models. Vaccine (2021) 39:5358–67. doi: 10.1016/j.vaccine.2021.08.011
240. Bialas KM, Tanaka T, Tran D, Varner V, de la Rosa CE, Chiuppesi F, et al. Maternal CD4+ T Cells Protect Against Severe Congenital Cytomegalovirus Disease in a Novel Nonhuman Primate Model of Placental Cytomegalovirus Transmission. Proc Natl Acad Sci (2015) 112:13645–50. doi: 10.1073/pnas.1511526112
241. Nelson CS, Cruz DV, Tran D, Bialas KM, Stamper L, Wu H, et al. Preexisting Antibodies can Protect Against Congenital Cytomegalovirus Infection in Monkeys. JCI Insight (2017) 2(13):e94002. doi: 10.1172/jci.insight.94002
242. Itell HL, Kaur A, Deere JD, Barry PA, Permar SR. Rhesus Monkeys for a Nonhuman Primate Model of Cytomegalovirus Infections. Curr Opin Virol (2017) 25:126–33. doi: 10.1016/j.coviro.2017.08.005
243. Albrecht M, Arck PC. Vertically Transferred Immunity in Neonates: Mothers, Mechanisms and Mediators. Front Immunol (2020) 11. doi: 10.3389/fimmu.2020.00555
244. Sadiq A, Bostan N, Yinda KC, Naseem S, Sattar S. Rotavirus: Genetics, Pathogenesis and Vaccine Advances. Rev Med Virol (2018) 28:e2003. doi: 10.1002/rmv.2003
245. Crawford SE, Ramani S, Tate JE, Parashar UD, Svensson L, Hagbom M, et al. Rotavirus Infection. Nat Rev Dis Primers (2017) 3:17083. doi: 10.1038/nrdp.2017.83
246. Gomez-Rial J, Rivero-Calle I, Salas A, Martinon-Torres F. Rotavirus and Autoimmunity. J Infect (2020) 81:183–9. doi: 10.1016/j.jinf.2020.04.041
247. Kirkwood CD, Ma LF, Carey ME, Steele AD. The Rotavirus Vaccine Development Pipeline. Vaccine (2019) 37:7328–35. doi: 10.1016/j.vaccine.2017.03.076
248. Cárcamo-Calvo R, Muñoz C, Buesa J, Rodríguez-Díaz J, Gozalbo-Rovira R. The Rotavirus Vaccine Landscape, an Update. Pathog (Basel Switzerland) (2021) 10:520. doi: 10.3390/pathogens10050520
249. Anderson EJ, Weber SG. Rotavirus Infection in Adults. Lancet Infect Dis (2004) 4:91–9. doi: 10.1016/S1473-3099(04)00928-4
250. Hagbom M, Sharma S, Lundgren O, Svensson L. Towards a Human Rotavirus Disease Model. Curr Opin Virol (2012) 2:408–18. doi: 10.1016/j.coviro.2012.05.006
251. Cortese MM, Haber P. Rotavirus (2021). Centers for Disease Control and Prevention. Available at: https://www.cdc.gov/vaccines/pubs/pinkbook/rota.html#features (Accessed 2021).
252. Payne DC, Mcneal M, Staat MA, Piasecki AM, Cline A, Defranco E, et al. Persistence of Maternal Anti-Rotavirus Immunoglobulin G in the Post-Rotavirus Vaccine Era. J Infect Dis (2021) 224:133–6. doi: 10.1093/infdis/jiaa715
253. Otero CE, Langel SN, Blasi M, Permar SR. Maternal Antibody Interference Contributes to Reduced Rotavirus Vaccine Efficacy in Developing Countries. PloS Pathog (2020) 16:e1009010. doi: 10.1371/journal.ppat.1009010
254. Appaiahgari MB, Glass R, Singh S, Taneja S, Rongsen-Chandola T, Bhandari N, et al. Transplacental Rotavirus IgG Interferes With Immune Response to Live Oral Rotavirus Vaccine ORV-116E in Indian Infants. Vaccine (2014) 32:651–6. doi: 10.1016/j.vaccine.2013.12.017
255. Trang NV, Braeckman T, Lernout T, Hau VT, Anh Le TK, Luan Le T, et al. Prevalence of Rotavirus Antibodies in Breast Milk and Inhibitory Effects to Rotavirus Vaccines. Hum Vaccin Immunother (2014) 10:3681–7. doi: 10.4161/21645515.2014.980204
256. Zweigart MR, Becker-Dreps S, Bucardo F, González F, Baric RS, Lindesmith LC. Serological Humoral Immunity Following Natural Infection of Children With High Burden Gastrointestinal Viruses. Viruses (2021) 13:2033. doi: 10.3390/v13102033
257. Ward RL, Mcneal MM, Sheridan JF. Development of an Adult Mouse Model for Studies on Protection Against Rotavirus. J Virol (1990) 64:5070–5. doi: 10.1128/jvi.64.10.5070-5075.1990
258. Langel S, Steppe J, Chang J, Travieso T, Webster H, Otero C, et al. Protective Transfer: Maternal Passive Immunization With a Rotavirus-Neutralizing Dimeric IgA Protects Against Rotavirus Disease in Suckling Neonates. bioRxiv (2021) 2021.2009.2021.461116. doi: 10.1101/2021.09.21.461116
259. Padilla-Carlin DJ, Mcmurray DN, Hickey AJ. The Guinea Pig as a Model of Infectious Diseases. Comp Med (2008) 58:324–40.
260. Glenn GM, Fries LF, Smith G, Kpamegan E, Lu H, Guebre-Xabier M, et al. Modeling Maternal Fetal RSV F Vaccine Induced Antibody Transfer in Guinea Pigs. Vaccine (2015) 33:6488–92. doi: 10.1016/j.vaccine.2015.08.039
261. Lakatos K, Mcadams D, White JA, Chen D. Formulation and Preclinical Studies With a Trivalent Rotavirus P2-VP8 Subunit Vaccine. Hum Vaccin Immunother (2020) 16:1957–68. doi: 10.1080/21645515.2019.1710412
262. Chepngeno J, Diaz A, Paim FC, Saif LJ, Vlasova AN. Rotavirus C: Prevalence in Suckling Piglets and Development of Virus-Like Particles to Assess the Influence of Maternal Immunity on the Disease Development. Vet Res (2019) 50:84. doi: 10.1186/s13567-019-0705-4
263. Yin N, Yang FM, Qiao HT, Zhou Y, Duan SQ, Lin XC, et al. Neonatal Rhesus Monkeys as an Animal Model for Rotavirus Infection. World J Gastroenterol (2018) 24:5109–19. doi: 10.3748/wjg.v24.i45.5109
264. Meier AF, Suter M, Schraner EM, Humbel BM, Tobler K, Ackermann M, et al. Transfer of Anti-Rotavirus Antibodies During Pregnancy and in Milk Following Maternal Vaccination With a Herpes Simplex Virus Type-1 Amplicon Vector. Int J Mol Sci (2017) 18(2):431. doi: 10.3390/ijms18020431
265. Chhabra P, De Graaf M, Parra GI, Chan MC, Green K, Martella V, et al. Updated Classification of Norovirus Genogroups and Genotypes. J Gen Virol (2019) 100:1393–406. doi: 10.1099/jgv.0.001318
266. Bhar S, Jones MK. In Vitro Replication of Human Norovirus. Viruses (2019) 11(6):547. doi: 10.3390/v11060547
267. Centers for Disease Control and Prevention (Cdc). Common Settings of Norovirus Outbreaks (2021). Centers for Disease Control and Prevention Available at: https://www.cdc.gov/norovirus/trends-outbreaks/outbreaks.html (Accessed 2021).
268. Cdc. Burden of Norovirus Illness in the U.S (2021). Available at: https://www.cdc.gov/norovirus/trends-outbreaks/burden-US.html (Accessed 2022).
269. Banyai K, Estes MK, Martella V, Parashar UD. Viral Gastroenteritis. Lancet (2018) 392:175–86. doi: 10.1016/S0140-6736(18)31128-0
270. Cannon JL, Lopman BA, Payne DC, Vinje J. Birth Cohort Studies Assessing Norovirus Infection and Immunity in Young Children: A Review. Clin Infect Dis (2019) 69:357–65. doi: 10.1093/cid/ciy985
271. Lopman BA, Trivedi T, Vicuña Y, Costantini V, Collins N, Gregoricus N, et al. Norovirus Infection and Disease in an Ecuadorian Birth Cohort: Association of Certain Norovirus Genotypes With Host Fut2 Secretor Status. J Infect Dis (2015) 211:1813–21. doi: 10.1093/infdis/jiu672
272. Menon VK, George S, Sarkar R, Giri S, Samuel P, Vivek R, et al. Norovirus Gastroenteritis in a Birth Cohort in Southern India. PloS One (2016) 11:e0157007–e0157007. doi: 10.1371/journal.pone.0157007
273. Mallory ML, Lindesmith LC, Graham RL, Baric RS. Gii.4 Human Norovirus: Surveying the Antigenic Landscape. Viruses (2019) 11:177. doi: 10.3390/v11020177
274. Graziano VR, Wei J, Wilen CB. Norovirus Attachment and Entry. Viruses (2019) 11(6):495. doi: 10.3390/v11060495
275. Labayo HKM, Pajuelo MJ, Tohma K, Ford-Siltz LA, Gilman RH, Cabrera L, et al. Norovirus-Specific Immunoglobulin A in Breast Milk for Protection Against Norovirus-Associated Diarrhea Among Infants. EClinicalMedicine (2020) 27:100561. doi: 10.1016/j.eclinm.2020.100561
276. Rouhani S, Penataro Yori P, Paredes Olortegui M, Siguas Salas M, Rengifo Trigoso D, Mondal D, et al. Norovirus Infection and Acquired Immunity in 8 Countries: Results From the MAL-ED Study. Clin Infect Dis (2016) 62:1210–7. doi: 10.1093/cid/ciw072
277. Taube S, Kolawole AO, Hohne M, Wilkinson JE, Handley SA, Perry JW, et al. A Mouse Model for Human Norovirus. mBio (2013) 4(4):e00450-13. doi: 10.1128/mBio.00450-13
278. Todd KV, Tripp RA. Human Norovirus: Experimental Models of Infection. Viruses (2019) 11(2):151. doi: 10.3390/v11020151
279. De Rijk EPCT, Van Esch E. The Macaque Placenta—a Mini-Review. Toxicol Pathol (2008) 36:108S–18S. doi: 10.1177/0192623308326095
280. Jorquera PA, Anderson L, Tripp RA. Human Respiratory Syncytial Virus: An Introduction. In: Tripp RA, Jorquera PA, editors. Human Respiratory Syncytial Virus: Methods and Protocols. New York, NY: Springer New York (2016). p. 1–12.
281. González-Ortiz AM, Bernal-Silva S, Comas-García A, Vega-Morúa M, Garrocho-Rangel ME, Noyola DE. Severe Respiratory Syncytial Virus Infection in Hospitalized Children. Arch Med Res (2019) 50(6):377–83. doi: 10.1016/j.arcmed.2019.10.005
282. Manti S, Esper F, Alejandro-Rodriguez M, Leonardi S, Betta P, Cuppari C, et al. Respiratory Syncytial Virus Seropositivity at Birth Is Associated With Adverse Neonatal Respiratory Outcomes. Pediatr Pulmonol (2020) 55(11):3074–9. doi: 10.1002/ppul.25001
283. Wheeler SM, Dotters-Katz S, Heine RP, Grotegut CA, Swamy GK. Maternal Effects of Respiratory Syncytial Virus Infection during Pregnancy. Emerg Infect Dis (2015) 21(11):1951–5. doi: 10.3201/eid2111.150497
284. Regan AK, Klein NP, Langley G, Drews SJ, Buchan S, Ball S, et al. Respiratory Syncytial Virus Hospitalization During Pregnancy in 4 High-income Countries, 2010-2016. Clin Infect Dis (2018) 67(12):1915–8. doi: 10.1093/cid/ciy439
285. Brown PM, Harford TJ, Agrawal V, Yen-Lieberman B, Rezaee F, Piedimonte G. Prenatal Exposure to Respiratory Syncytial Virus Alters Postnatal Immunity and Airway Smooth Muscle Contractility During Early-Life Reinfections. PloS One (2017) 12:e0168786. doi: 10.1371/journal.pone.0168786
286. Buchwald AG, Graham BS, Traore A, Haidara FC, Chen M, Morabito K, et al. Respiratory Syncytial Virus (Rsv) Neutralizing Antibodies at Birth Predict Protection From RSV Illness in Infants in the First 3 Months of Life. Clin Infect Dis (2021) 73:e4421–7. doi: 10.1093/cid/ciaa648
287. Jans J, Wicht O, Widjaja I, Ahout IML, De Groot R, Guichelaar T, et al. Characteristics of RSV-Specific Maternal Antibodies in Plasma of Hospitalized, Acute RSV Patients Under Three Months of Age. PloS One (2017) 12:e0170877. doi: 10.1371/journal.pone.0170877
288. Schwarz TF, Johnson C, Grigat C, Apter D, Csonka P, Lindblad N, et al. Three Dose Levels of a Maternal Respiratory Syncytial Virus Vaccine Candidate Are Well Tolerated and Immunogenic in a Randomized Trial in Nonpregnant Women. J Infect Dis (2021) 19:jiab317. doi: 10.1093/infdis/jiab317
289. Madhi SA, Polack FP, Piedra PA, Munoz FM, Trenholme AA, Simões E, et al. Respiratory Syncytial Virus Vaccination During Pregnancy and Effects in Infants. New Engl J Med (2020) 383:426–39. doi: 10.1056/NEJMoa1908380
290. Taylor G. Animal Models of Respiratory Syncytial Virus Infection. Vaccine (2017) 35:469–80. doi: 10.1016/j.vaccine.2016.11.054
291. Belshe RB, Richardson LS, London WT, Sly DL, Lorfeld JH, Camargo E, et al. Experimental Respiratory Syncytial Virus Infection of Four Species of Primates. J Med Virol (1977) 1:157–62. doi: 10.1002/jmv.1890010302
292. Citron MP, Mcanulty J, Callahan C, Knapp W, Fontenot J, Morales P, et al. Transplacental Antibody Transfer of Respiratory Syncytial Virus Specific IgG in Non-Human Primate Mother-Infant Pairs. Pathogens (2021) 10:1441. doi: 10.3390/pathogens10111441
293. Who. Herpes Simplex Virus (2022). Available at: https://www.who.int/news-room/fact-sheets/detail/herpes-simplex-virus (Accessed 2022).
294. Hammad W, Konje JC. Herpes Simplex Virus Infection in Pregnancy - An Update. Eur J Obstet Gynecol Reprod Biol (2021) 259:38–45. doi: 10.1016/j.ejogrb.2021.01.055
295. James SH, Sheffield JS, Kimberlin DW. Mother-to-Child Transmission of Herpes Simplex Virus. J Pediatr Infect Dis Soc (2014) 3(Suppl 1):S19–23. doi: 10.1093/jpids/piu050
297. Amin I, Vajeeha A, Younas S, Afzal S, Shahid M, Nawaz R, et al. Hsv-1 Infection: Role of Viral Proteins and Cellular Receptors. Crit Rev Eukaryot Gene Expr (2019) 29:461–9. doi: 10.1615/CritRevEukaryotGeneExpr.2019025561
298. Samies NL, James SH, Kimberlin DW. Neonatal Herpes Simplex Virus Disease: Updates and Continued Challenges. Clin Perinatol (2021) 48:263–74. doi: 10.1016/j.clp.2021.03.003
299. James SH, Sheffield JS, Kimberlin DW. Mother-to-Child Transmission of Herpes Simplex Virus. J Pediatr Infect Dis Soc (2014) 3(Suppl 1):S19–23. doi: 10.1093/jpids/piu050
300. Duff P. Prevention of Opportunistic Infections in Women With HIV Infection. Clin Obstet Gynecol (2019) 62:816–22. doi: 10.1097/GRF.0000000000000483
301. Looker KJ, Magaret AS, May MT, Turner KME, Vickerman P, Newman LM, et al. First Estimates of the Global and Regional Incidence of Neonatal Herpes Infection. Lancet Glob Health (2017) 5:e300–9. doi: 10.1016/S2214-109X(16)30362-X
302. Patel CD, Backes IM, Taylor SA, Jiang Y, Marchant A, Pesola JM, et al. Maternal Immunization Confers Protection Against Neonatal Herpes Simplex Mortality and Behavioral Morbidity. Sci Transl Med (2019) 11(487):eaau6039. doi: 10.1126/scitranslmed.aau6039
303. Patel CD, Taylor SA, Mehrbach J, Awasthi S, Friedman HM, Leib DA. Trivalent Glycoprotein Subunit Vaccine Prevents Neonatal Herpes Simplex Virus Mortality and Morbidity. J Virol (2020) 94(11):e02163-19. doi: 10.1128/JVI.02163-19
304. Jiang Y, Patel CD, Manivanh R, North B, Backes IM, Posner DA, et al. Maternal Antiviral Immunoglobulin Accumulates in Neural Tissue of Neonates to Prevent Hsv Neurological Disease. mBio (2017) 8(4):e00678-17. doi: 10.1128/mBio.00678-17
305. Yorty JL, Bonneau RH. Impact of Maternal Stress on the Transmammary Transfer and Protective Capacity of Herpes Simplex Virus-Specific Immunity. Am J Physiol Regul Integr Comp Physiol (2004) 287:R1316–1324. doi: 10.1152/ajpregu.00685.2003
306. Bernstein DI, Cardin RD, Smith GA, Pickard GE, Sollars PJ, Dixon DA, et al. The R2 non-Neuroinvasive HSV-1 Vaccine Affords Protection From Genital HSV-2 Infections in a Guinea Pig Model. NPJ Vaccines (2020) 5:104. doi: 10.1038/s41541-020-00254-8
307. Stanfield BA, Kousoulas KG, Fernandez A, Gershburg E. Rational Design of Live-Attenuated Vaccines Against Herpes Simplex Viruses. Viruses (2021) 13(8):1637. doi: 10.3390/v13081637
308. Friedman HM, Cohen GH, Eisenberg RJ, Seidel CA, Cines DB. Glycoprotein C of Herpes Simplex Virus 1 Acts as a Receptor for the C3b Complement Component on Infected Cells. Nature (1984) 309:633–5. doi: 10.1038/309633a0
309. Fries LF, Friedman HM, Cohen GH, Eisenberg RJ, Hammer CH, Frank MM. Glycoprotein C of Herpes Simplex Virus 1 is an Inhibitor of the Complement Cascade. J Immunol (1986) 137:1636–41. doi: 10.1038/309633a0
310. Mcnearney TA, Odell C, Holers VM, Spear PG, Atkinson JP. Herpes Simplex Virus Glycoproteins gC-1 and gC-2 Bind to the Third Component of Complement and Provide Protection Against Complement-Mediated Neutralization of Viral Infectivity. J Exp Med (1987) 166:1525–35. doi: 10.1084/jem.166.5.1525
311. Dubin G, Socolof E, Frank I, Friedman HM. Herpes Simplex Virus Type 1 Fc Receptor Protects Infected Cells From Antibody-Dependent Cellular Cytotoxicity. J Virol (1991) 65:7046–50. doi: 10.1128/jvi.65.12.7046-7050.1991
312. Gerber SI, Belval BJ, Herold BC. Differences in the Role of Glycoprotein C of HSV-1 and HSV-2 in Viral Binding may Contribute to Serotype Differences in Cell Tropism. Virology (1995) 214:29–39. doi: 10.1006/viro.1995.9957
313. Sattentau Q. Avoiding the Void: Cell-to-Cell Spread of Human Viruses. Nat Rev Microbiol (2008) 6:815–26. doi: 10.1038/nrmicro1972
314. Aschner CB, Herold BC. Alphaherpesvirus Vaccines. Curr Issues Mol Biol (2021) 41:469–508. doi: 10.21775/cimb.041.469
315. Belshe RB, Leone PA, Bernstein DI, Wald A, Levin MJ, Stapleton JT, et al. Efficacy Results of a Trial of a Herpes Simplex Vaccine. N Engl J Med (2012) 366:34–43. doi: 10.1056/NEJMoa1103151
316. Bernstein DI. Use of the Guinea Pig Model of Genital Herpes to Evaluate Vaccines and Antivirals: Review. Antiviral Res (2020) 180:104821. doi: 10.1016/j.antiviral.2020.104821
317. Felker AM, Nguyen P, Kaushic C. Primary HSV-2 Infection in Early Pregnancy Results in Transplacental Viral Transmission and Dose-Dependent Adverse Pregnancy Outcomes in a Novel Mouse Model. Viruses (2021) 13(10):1929. doi: 10.3390/v13101929
318. Lo M, Zhu J, Hansen SG, Carroll T, Farr Zuend C, Nöel-Romas L, et al. Acute Infection and Subsequent Subclinical Reactivation of Herpes Simplex Virus 2 After Vaginal Inoculation of Rhesus Macaques. J Virol (2019) 93(2):e01574-18. doi: 10.1128/JVI.01574-18
319. Tsukuda S, Watashi K. Hepatitis B Virus Biology and Life Cycle. Antiviral Res (2020) 182:104925. doi: 10.1016/j.antiviral.2020.104925
320. Schweitzer A, Horn J, Mikolajczyk RT, Krause G, Ott JJ. Estimations of Worldwide Prevalence of Chronic Hepatitis B Virus Infection: A Systematic Review of Data Published Between 1965 and 2013. Lancet (2015) 386:1546–55. doi: 10.1016/S0140-6736(15)61412-X
321. Polaris Observatory C. Global Prevalence, Treatment, and Prevention of Hepatitis B Virus Infection in 2016: A Modelling Study. Lancet Gastroenterol Hepatol (2018) 3:383–403. doi: 10.1016/S2468-1253(18)30056-6
322. Cdc. Hepatitis B (2021). Available at: https://www.cdc.gov/hepatitis/hbv/index.htm (Accessed 2022).
323. Yeung LT, Roberts EA. Hepatitis B in Childhood: An Update for the Paediatrician. Paediatr Child Health (2001) 6:655–9. doi: 10.1093/pch/6.9.655
324. Cdc. Perinatal Transmission (2022). Available at: https://www.cdc.gov/hepatitis/hbv/perinatalxmtn.htm (Accessed 2022).
325. Global Burden of Disease Study, C. Global, Regional, and National Incidence, Prevalence, and Years Lived With Disability for 301 Acute and Chronic Diseases and Injuries in 188 Countries 1990-2013: A Systematic Analysis for the Global Burden of Disease Study 2013. Lancet (2015) 386:743–800. doi: 10.1016/S0140-6736(15)60692-4
326. Collaboration G.B.O.D.L.C. The Burden of Primary Liver Cancer and Underlying Etiologies From 1990 to 2015 at the Global, Regional, and National Level: Results From the Global Burden of Disease Study 2015. JAMA Oncol (2017) 3:1683–91. doi: 10.1001/jamaoncol.2017.3055
327. Abdel-Hady M, Kelly D. Chronic Hepatitis B in Children and Adolescents: Epidemiology and Management. Paediatr Drugs (2013) 15:311–7. doi: 10.1007/s40272-013-0010-z
328. Kappus MR, Sterling RK. Extrahepatic Manifestations of Acute Hepatitis B Virus Infection. Gastroenterol Hepatol (N Y) (2013) 9:123–6.
329. Stevens CE, Beasley RP, Tsui J, Lee W-C. Vertical Transmission of Hepatitis B Antigen in Taiwan. N Engl J Med (1975) 292:771–4. doi: 10.1056/NEJM197504102921503
330. Mcmahon BJ, Alward WL, Hall DB, Heyward WL, Bender TR, Francis DP, et al. Acute Hepatitis B Virus Infection: Relation of Age to the Clinical Expression of Disease and Subsequent Development of the Carrier State. J Infect Dis (1985) 151:599–603. doi: 10.1093/infdis/151.4.599
331. Beasley RP. Rocks Along the Road to the Control of HBV and HCC. Ann Epidemiol (2009) 19:231–4. doi: 10.1016/j.annepidem.2009.01.017
332. Keane E, Funk AL, Shimakawa Y. Systematic Review With Meta-Analysis: The Risk of Mother-to-Child Transmission of Hepatitis B Virus Infection in sub-Saharan Africa. Aliment Pharmacol Ther (2016) 44:1005–17. doi: 10.1111/apt.13795
333. Indolfi G, Easterbrook P, Dusheiko G, Siberry G, Chang MH, Thorne C, et al. Hepatitis B Virus Infection in Children and Adolescents. Lancet Gastroenterol Hepatol (2019) 4:466–76. doi: 10.1016/S2468-1253(19)30042-1
334. Terrault NA, Levy MT, Cheung KW, Jourdain G. Viral Hepatitis and Pregnancy. Nat Rev Gastroenterol Hepatol (2021) 18:117–30. doi: 10.1038/s41575-020-00361-w
335. Schillie S, Walker T, Veselsky S, Crowley S, Dusek C, Lazaroff J, et al. Outcomes of Infants Born to Women Infected With Hepatitis B. Pediatrics (2015) 135:e1141–1147. doi: 10.1542/peds.2014-3213
336. Eke AC, Eleje GU, Eke UA, Xia Y, Liu J. Hepatitis B Immunoglobulin During Pregnancy for Prevention of Mother-to-Child Transmission of Hepatitis B Virus. Cochrane Database Syst Rev (2017) 2:CD008545. doi: 10.1002/14651858.CD008545.pub2
337. Ingardia CJ, Kelley L, Lerer T, Wax JR, Steinfeld JD. Correlation of Maternal and Fetal Hepatitis B Antibody Titers Following Maternal Vaccination in Pregnancy. Am J Perinatol (1999) 16:129–32. doi: 10.1055/s-2007-993846
338. Makris MC, Polyzos KA, Mavros MN, Athanasiou S, Rafailidis PI, Falagas ME. Safety of Hepatitis B, Pneumococcal Polysaccharide and Meningococcal Polysaccharide Vaccines in Pregnancy: A Systematic Review. Drug Saf (2012) 35:1–14. doi: 10.2165/11595670-000000000-00000
339. Wieland SF. The Chimpanzee Model for Hepatitis B Virus Infection. Cold Spring Harb Perspect Med (2015) 5(6):a021469. doi: 10.1101/cshperspect.a021428
340. Hu J, Lin YY, Chen PJ, Watashi K, Wakita T. Cell and Animal Models for Studying Hepatitis B Virus Infection and Drug Development. Gastroenterology (2019) 156:338–54. doi: 10.1053/j.gastro.2018.06.093
Keywords: pregnancy, mother-to-child transmission, congenital disease, early-life, breastmilk, vaccination
Citation: Goswami R, Pavon CG, Miller IG, Berendam SJ, Williams CA, Rosenthal D, Gross M, Phan C, Byrd A, Pollara J, Permar SR and Fouda GG (2022) Prenatal Immunization to Prevent Viral Disease Outcomes During Pregnancy and Early Life. Front.Virol. 2:849995. doi: 10.3389/fviro.2022.849995
Received: 07 January 2022; Accepted: 19 April 2022;
Published: 19 May 2022.
Edited by:
Louise Kuhn, Columbia University, United StatesReviewed by:
Filemón Bucardo, University of Nicaragua, León, NicaraguaAlessandra Pierangeli, Sapienza University of Rome, Italy
Dana Wolf, Hadassah-Hebrew University Medical Center, Israel
Copyright © 2022 Goswami, Pavon, Miller, Berendam, Williams, Rosenthal, Gross, Phan, Byrd, Pollara, Permar and Fouda. This is an open-access article distributed under the terms of the Creative Commons Attribution License (CC BY). The use, distribution or reproduction in other forums is permitted, provided the original author(s) and the copyright owner(s) are credited and that the original publication in this journal is cited, in accordance with accepted academic practice. No use, distribution or reproduction is permitted which does not comply with these terms.
*Correspondence: Genevieve G. Fouda, genevieve.fouda@duke.edu; Ria Goswami, rig4007@med.cornell.edu